DOI:
10.1039/C5RA19541A
(Paper)
RSC Adv., 2015,
5, 99313-99321
The effect of functionalized benzoxazine with allyl groups on the dielectric, mechanical and thermal properties of BMI/BADCy composites
Received
22nd September 2015
, Accepted 6th November 2015
First published on 6th November 2015
Abstract
Functionalized benzoxazine with allyl groups has recently attracted a great deal of attention due to its polymerizable group. In order to improve the properties of 4,4′-bismaleimidodiphenyl methane (BMI)/cyanate ester (BADCy), benzoxazine (Boz) was added into the BMI/BADCy system in this paper. The effect of functionalized Boz with allyl groups on the dielectric, mechanical and thermal properties of BMI/BADCy composites was systematically investigated in detail using mechanical measurements, scanning electron microscopy (SEM), dynamic mechanical analysis (DMA) and thermo-gravimetric analysis (TGA). The results showed that the formation of Mannich bridge networks and an interpenetrating polymer network through the polymerization of Bz-allyl and BMI/BADCy increased the cross-linking densities and thermal stability. Allyl-based polymers also exhibited high glass transition temperatures, and higher char yields. The dielectric constant value and the dielectric loss factor of BBz2 reached minimum values of 3.17 and 0.083 at 106 Hz respectively. The mechanical properties (a high flexural modulus of 4.18 GPa and flexural strength of 120 MPa) of BBz2 were superior. Scanning electron microscopy analysis showed a distinct characteristic of ductile fractures for the blends.
Introduction
Bismaleimides (BMIs) are an important class of addition-type polyimides which have gained much importance as a high-performance, thermoset matrix system. Their success in the area of aerospace structural applications is mostly due to their flame retardation and oxidative stability, excellent thermal stability and low propensity to moisture absorption at elevated temperatures, especially in hot/wet environments.1–5 Cyanate ester (BADCy) resin is a well-known thermosetting resin with outstanding thermal stability, moisture resistance, and a low dielectric constant and loss after being fully cured to form a triazine network.6–8
A bismaleimide/cyanate ester (BMI/BADCy) resin is selected as the model for modification owing to its widespread use in the electronics field, such as circuit boards, interconnects, and adhesives for microelectronics applications (e.g., flexible and rigid die attach adhesives).9,10 However, there are also several problems with BMIs; high curing temperature and brittleness. In addition, their relatively high dielectric constants are not suitable for application in the electronics industry.11,12 Previous studies have showed that 2,2′-diallylbisphenol A (DBA) was one of the most effective modifiers for improving their processibility and toughness. It can copolymerize with BMI through “Ene” and “Diels–Alder” reactions.13–15 Another point is that the phenol groups in this allyl compound may act as a catalyst for the cyclotrimerization of cyanate esters.16
Polybenzoxazines possess the typical characteristics of traditional phenolic resins such as excellent flame retardance, heat resistance and electronic properties. The major advantages of typical polybenzoxazines are associated with the existence of inter- and intramolecular hydrogen bonds in the network structure.17–19 Polymerizable group-containing monomers have attracted much attention because they can form a cross-linked network structure. Hence, it exhibits enhanced thermal and mechanical performance while preserving the beneficial properties of polybenzoxazine. Benzoxazine monomers (Boz) containing allyl groups can form highly cross-linked network structures after thermal treatment. The Ishida group20 has synthesized an allyl-containing benzoxazine monomer using allylphenol, aniline, and paraformaldehyde. Also, Tarek Agag21 reported the preparation of a similar benzoxazine monomer from phenol, bisphenol A with allylamine and formaldehyde. Therefore, it is of interest to study resin systems based on blends of Boz and BMI/BADCy resins. In such systems, Boz and BMI/BADCy are crosslinked through different reactions to form networks which interpenetrate each other in the cured resin.22 This characteristic structure may result in a synergistic effect on the properties of the polymer network, which is expected to couple the prominent dielectric properties of the cyanate ester resin with the high temperature resistance and remarkable mechanical and processing behaviors of the modified BMI resin.
Since the molecular structure of Boz can be designed,23 we synthesized several Boz monomers containing several allyl groups, which were used as new modifiers for the BMI/BADCy resin, and then a series of Boz modified BMI/BADCy resins were prepared. The focus of this paper is on evaluating the effect of Boz and the content of allyl groups on the thermal and dielectric properties, and consequently developing a new way for preparing high performance resins simultaneously with outstanding thermal and dielectric properties.
Experimental
Materials
Bisphenol A cyanate ester (BADCy) resins were purchased from Shangyu Shengda Biochemical Co. Ltd. (Shangyu, China). 4,4′-Bismaleimidodiphenyl methane (BMI) was received from Hubei Fengguang Chemicals, China. Bz-a, Bz-allyl-1 and Bz-allyl-2 were prepared from bisphenol A, aniline and paraformaldehyde, diallyl bisphenol A, aniline and paraformaldehyde, and diallyl bisphenol A, allylamine and paraformaldehyde, respectively, according to the method previously described in the literature.23 The chemical structures of BADCy, BMI, Bz-a, Bz-allyl-1 and Bz-allyl-2 are shown in Fig. 1.
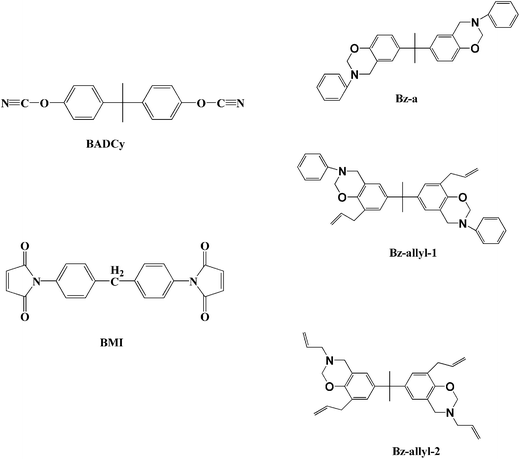 |
| Fig. 1 The chemical structures of BADCy, BMI, Bz-a, Bz-allyl-1 and Bz-allyl-2. | |
Preparation of ternary blends
Appropriate quantities of BADCy and BMI were placed in a three-necked flask with a mechanical stirrer and a thermometer. The mass ratio of BADCy and BMI was 4
:
3. The mixture was heated to 160 °C and maintained at that temperature with stirring until a homogeneous liquid was obtained. The liquid, which is a BMI/BADCy prepolymer, was maintained at that temperature for an additional 0.5 h. After that, the mixture was cooled to 140 °C and Boz was added, then the ternary mixture was stirred for 20 min and a brown-red transparent liquid was obtained. Bz-a, Bz-allyl-1 and Bz-allyl-2 were blended with BMI/BADCy, respectively. The blends were denoted as BBz, BBz1 and BBz2, respectively.
Preparation of cured resins
Firstly, the blends were poured into a preheated mold with a silicone coating on the inner surface. Then, the blends were degassed in a vacuum oven (120 °C for 0.5 h under vacuum). Finally, the blends were cured following the procedure of 150 °C/2 h + 180 °C/3 h + 200 °C/2 h, and post-cured at 240 °C/2 h.
Characterization
The impact strength was measured according to GB/T2567-2008 on a testing machine (ZBC-50A Plastic Pendulum Impact Testing Machine, Shenzhen Sans Measurement Technology Co. Ltd, Shenzhen, China). The sample dimension was (50 ± 0.02) × (7 ± 0.02) × (4 ± 0.02) mm3. The flexural strength and modulus were measured according to GB/T2567-2008 on a testing machine (CMT-6303 Electronic Tensile Testing Machine, Shenzhen Sans Measurement Technology Co. Ltd, Shenzhen, China). The sample dimension was (80 ± 0.02) × (10 ± 0.02) × (4 ± 0.02) mm3. The K1C value was determined using a three-point bending test for each precracked sample at room temperature according to STM Standard D 5528-01, using single edge notched bending tests measured in terms of the critical stress intensity factor (K1C). The samples were tested to failure at a cross-head speed of 1 mm min−1 at the loading point using a CMT-6303 Electronic Tensile Testing Machine. The density was measured according to GB 1033-1986 on a testing machine (JA2003J Electronic Analytical Balance, Shanghai Yueping scientific instrument Co. Ltd, Shanghai, China). Five samples were tested for each composition, and the results are presented as an average for the tested samples.
DSC measurements were performed with a Q1000DSC thermoanalyzer system (USA) ranging from room temperature to 380 °C under a N2 atmosphere at a heating rate of 10 °C min−1. A dry nitrogen flow of 40 ml min−1 was used as a purge gas. Samples of about 10 mg were enclosed in aluminium DSC capsules. Dynamic mechanical analysis (DMA) was performed with a Switzerland Mettler-Toledo DMA with a sample size of 45 mm × 6 mm × 3 mm. DMA tests were carried out from 25 to 380 °C with a heating rate of 3 °C min−1 at 1 Hz. TGA was performed using a Netzsch STA 449C thermogravimetric analyzer (Germany) at a heating rate of 10 °C min−1 under a N2 atmosphere from 20 to 800 °C.
The dielectric properties of the composites were tested using a broadband dielectric spectrometer (CONCEPE 80, Novocontrol Technology Company, Germany) with an Alpha-A high-performance frequency analyzer over the frequency range of 10−1 to 106 Hz.
Scanning electron micrographs were recorded on a TESCAN VEGA3 LMH instrument. The SEM accelerating voltage was 20 kV. The water absorption of a sample was determined by swelling in distilled water for 20 h at 100 °C. The sample dimension was (10 ± 0.02) × (10 ± 0.02) × (3 ± 0.02) mm3. FT-IR spectra were recorded as KBr pellets from 400 to 4000 cm−1 with a resolution of 4 cm−1 on a Nicolet IS10 IR spectrometer (USA).
Results and discussion
DSC analysis for the curing reaction
The curing procedures for the BMI/BADCy resin and the ternary blends were studied using DSC (Fig. 2). For the BMI/BADCy resin only one reaction exotherm was observed at a peak temperature of 305 °C. The BMI/BADCy resin polymerizes at a very high temperature, which does not usually required a catalyst. According to the figure, all of the exothermic peaks are single peaks, and the peak temperatures of the blends shift to lower temperatures as Boz is added. The curing reaction of BMI/BADCy is accelerated with the presence of Boz, because it occurs in the presence of phenolic hydroxyl groups, which are obtained from Boz. For the BBz, BBz1 and BBz2 blends, the content of allyl groups in the Boz monomer increases, which makes BMI/BADCy react with the allyl groups. The shift to a low temperature suggests that BMI/BADCy was induced to undergo a reaction in the presence of Boz and the allyl groups.
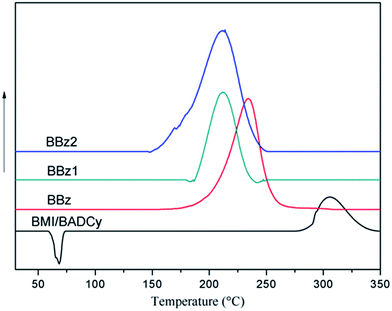 |
| Fig. 2 DSC curves of the BMI/BADCy resin and ternary blends. | |
Mechanical properties of the ternary blends
The tensile strength, impact strength, flexural strength, fracture toughness and density of the ternary blends are listed in Fig. 3 and 4 and Table 1. The three-point bend test was used to determine the flexural strength and modulus of the ternary blends. It is observed from Fig. 3 that BBz2 had the maximum impact strength (18.4 kJ m−2), which is increased by 54% compared with that of the BMI/BADCy resin. Our results also show that the flexural strength, flexural modulus and K1C of BBz2 can be enhanced by at least 41%, 14% and 30% compared with that of BMI/BADCy, respectively. Therefore, it can be concluded that the addition of Boz can efficiently improve the mechanical properties of the BMI/BADCy resin, especially when Boz has more allyl groups. The enhancement of the impact and flexural strength may be attributed to the network structure with a high crosslinking density and the flexible ether bonds in Boz/BMI/BADCy and the aliphatic chain in BBz2.
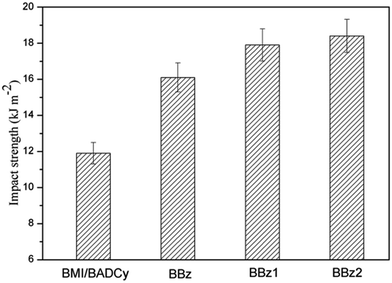 |
| Fig. 3 The impact strength of the BMI/BADCy resin and ternary blends. | |
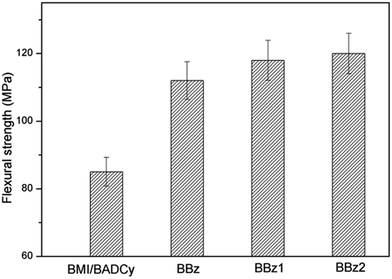 |
| Fig. 4 The flexural strength of the BMI/BADCy resin and ternary blends. | |
Table 1 The mechanical properties of the BMI/BADCy resin and ternary blends
Sample ID |
Impact strength (kJ m−2) |
Flexural strength (MPa) |
Flexural modulus (GPa) |
Tensile strength (MPa) |
Fracture toughness K1C (MPa m1/2) |
Density (g cm−3) |
BADCy/BMI |
11.9 (±0.2) |
85 (±2) |
3.65 (±0.05) |
41.6 (±0.3) |
0.91 (±0.02) |
1.2656 (±0.0001) |
BBz |
16.1 (±0.1) |
112 (±3) |
4.15 (±0.09) |
54.1 (±0.2) |
1.04 (±0.04) |
1.2499 (±0.0001) |
BBz1 |
17.9 (±0.2) |
118 (±3) |
4.09 (±0.03) |
61.8 (±0.5) |
1.16 (±0.02) |
1.2566 (±0.0001) |
BBz2 |
18.4 (±0.2) |
120 (±2) |
4.18 (±0.06) |
64.6 (±0.4) |
1.19 (±0.01) |
1.2563 (±0.0001) |
To investigate the possible curing reactions of the ternary blends, FT-IR was carried out. The FT-IR spectra of the BMI/BADCy resin and the ternary blends before and after curing are shown in Fig. 5. The absorption at 940 cm−1 assigned to the oxazine ring in the benzoxazine ring structure disappeared after curing, and the absorptions at 2271 and 2236 cm−1 assigned to the cyanate ester group also disappeared. The new absorptions at 1564 and 1371 cm−1 assigned to the triazine group appeared. The absorptions at 1638 cm−1 assigned to C
C decreased, due to the reaction between the allyl groups. The appearance of the absorption band at 1213 cm−1 revealed the formation of ether bonds, which may due to the reaction between BMI and PBoz as shown in Scheme 2e.
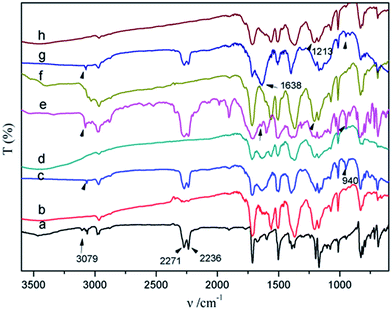 |
| Fig. 5 IR spectra of the BMI/BADCy resin and ternary blends before and after curing ((a): uncured BMI/BADCy, (b): cured BMI/BADCy, (c): uncured BBz, (d): cured BBz, (e): uncured BBz1, (f): cured BBz1, (g): uncured BBz2 and (h): cured BBz2). | |
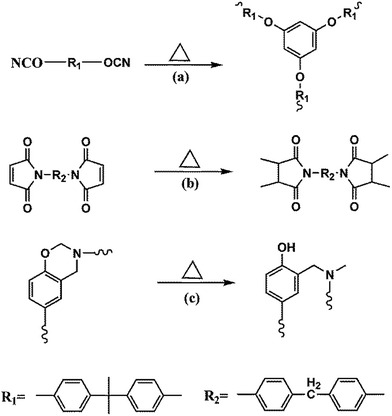 |
| Scheme 1 The curing procedures of BMI, BADCy and Boz. | |
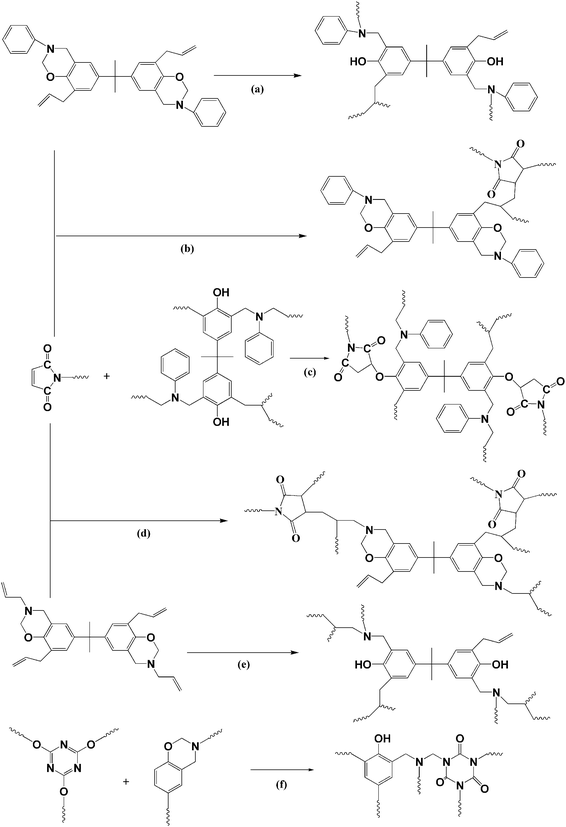 |
| Scheme 2 The curing procedure of the ternary blends. | |
In the process of BMI and BADCy curing, the thermal reactions of BMI and BADCy can occur as shown in Scheme 1a and b, respectively. However, the curing mechanism of the ternary blends is different from BMI/BADCy. Ene, Diels–Alder, homopolymerization and alternating copolymerization reactions are involved in the curing of BMI/BADCy modified with Boz.14,24 The ene reaction occurs at a lower temperature compared with that of the other reactions (Scheme 2a, b, d and e). The curing reactions of the blends of BADCy with BMI and Boz can occur (Scheme 1).25 Additionally, a part of Boz undergoes ring-opening polymerization to form polybenzoxazine and generate the phenolic hydroxyl group (Scheme 1c), which can react with BMI/BADCy (Scheme 2c and f). By increasing the temperature the oxazine ring can react completely. The allyl group on the oxazine ring can react completely (Scheme 2e). However, the allyl group on benzene seldom reacts (Scheme 2a) because of the stability of the radical by resonance.26 On one hand, the polymerization of the Boz/BMI/BADCy blends forms ether bonds, which result in good flexibility for the polymer. On the other hand, it may be attributed to the network structure with a high crosslinking density formed in BBz2. Moreover, the hydrogen bonds can break and reform during the loading and unloading, thus increasing the energy dissipation in the materials. Also, the hydrogen bond is a physical interaction which will increase the impact strength and flexural strength of the BMI/BADCy blends. Therefore, it can be concluded that the addition of Boz can efficiently improve the mechanical properties of the BMI/BADCy resin, especially Bz-allyl-2.
Fracture surfaces of the Bz-ally/BMI/BADCy resin systems
In order to further confirm the effect of Boz on the mechanical properties of the BMI/BADCy resin, SEM images of the fracture surfaces of samples after impact tests are taken and shown in Fig. 6. Fig. 6a–d presents SEM micrographs of the fracture surface of BMI/BADCy, BBz, BBz1 and BBz2, respectively. It can be observed that the BMI/BADCy resin showing a smooth and riverlike fracture surface (Fig. 6a) exhibits a typical brittle feature. While with the addition of Bz-a into the BMI/BADCy resin, the fracture surfaces become rougher and are accompanied with more ductile sunken areas, which is consistent with the improved impact strength of the composites. For BBz1, as shown in Fig. 6c, the ductile sunken area is more than that for BBz. In the case of BBz2, as shown in Fig. 6d, the fracture surface is much rougher than those of the BMI/BADCy resin, BBz and BBz1, and there are large amounts of ductile sunken areas, which can absorb the energy of the fracture and hinder the crack propagation, and it exhibits a typical rough feature. In summary, the BBz2 has a rough surface, more dimples and ductile sunken areas, and a high ductility and thus can absorb more energy during the impact process. The possible reason is that the co-curing of the allyl groups and BMI/BADCy has largely enhanced the toughness of the resin. The features of the fracture surfaces of the Boz/BMI/BADCy systems accord well with the mechanical properties.
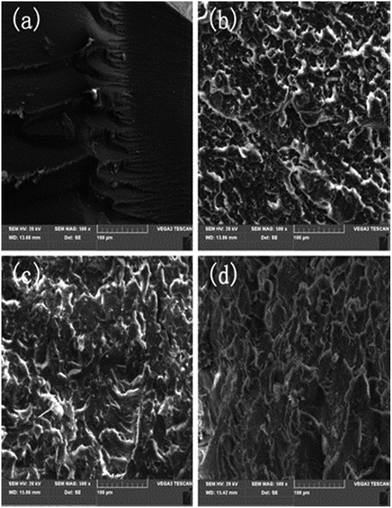 |
| Fig. 6 SEM images of the fracture surfaces of the BMI/BADCy resin and ternary blends. | |
DMA properties of the ternary blends
The changes of the Tg in the composites are particularly important, not only because they yield insights into the fundamental changes in polymer chain dynamics, but also because the associated gains in thermal stability are critical for several applications. Determined from the midpoints of the glass transition regions of the loss factor (tan
δ) curves in Fig. 7 and 8, the glass transition temperature (Tg) of various samples are listed in Table 2. From the dynamic mechanical spectra, it is observed that cured BMI/BADCy (Fig. 8) exhibits well-defined dynamic mechanical damping peaks centered at 245 and 308 °C, which are ascribed to the glass transitions of polycyanurate and polybismaleimide, respectively.27 However, it can be found that the Tg values for the composites with the different types of Boz correspond to one single glass transition. Lin et al. reported that the alkyl isocyanurate and diphenyl ether linkages, which result from the co-reaction of triazine and benzoxazine, randomly link the polycyanurate and polybenzoxazine domains, leading to a homogeneous thermoset between Boz and BADCy.28 However, one single glass transition indicates that the ternary blends are a homogeneous network. As there is only one single Tg we speculate further reactions take place between Boz and BMI/BADCy. The peak of tan
δ for the cured ternary blends has shifted to a lower temperature compared with that of cured BMI/BADCy and increases with increasing content of allyl groups in the Boz monomer. The increased allyl group loading improves the cross-linking between BMI/BADCy and Boz containing allyl groups, and thus restricts the segmental movement of the polymer chains, which leads to a higher Tg.15,16 In fact, Boz containing allyl groups has two opposite influences on the improvement of the Tg values of the composites. On one hand, the addition of Boz containing allyl groups can increase the cross-linking intensity of the cured system, which is beneficial to improve the Tg values of the composites. On the other hand, the thermal stability of an aliphatic chain is lower than that of rigid groups, which has a negative effect on increasing the Tg value of the composites.
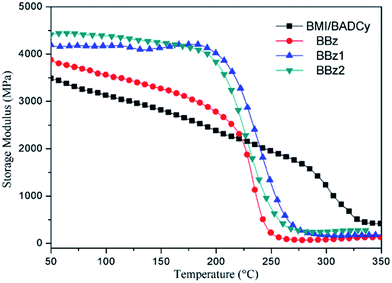 |
| Fig. 7 The storage modulus of the BMI/BADCy resin and ternary blends. | |
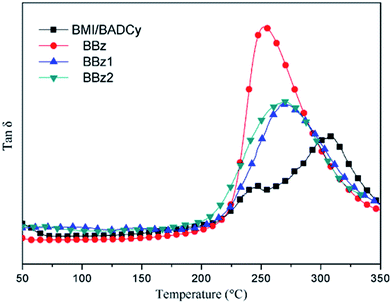 |
| Fig. 8 The temperature dependency of tan δ for the BMI/BADCy resin and ternary blends. | |
Table 2 Thermal properties of the BMI/BADCy resin and ternary blends
Sample |
BMI/BADCy |
BBz |
BBz1 |
BBz2 |
Td5 (°C) |
441 |
388 |
406 |
426 |
Td10 (°C) |
446 |
407 |
420 |
432 |
Char yield (%) |
35.8 |
44.2 |
41.9 |
40.4 |
Tg |
245, 308 |
252 |
271 |
268 |
Crosslinking density (mol m−3) |
103 |
103 |
103 |
103 |
Due to the strong interaction of intermolecular or intramolecular hydrogen bonds between the phenolic hydroxyl groups and the adjacent phenolic hydroxyl groups, or the N atoms on the Mannich bridges, or the π electrons of the benzene rings in the chemical structure of polybenzoxazine, copolymers of Boz with other resins have a higher initial modulus. With the increase in allyl groups in Boz, the initial storage modulus of the terpolymers improved. Based on the rubber elasticity theory and the following eqn (1),29–31 we calculated the crosslinking densities of the different crosslinked systems and the data are shown in Table 1.
where
ρ is the crosslinking density;
E is the storage modulus in the rubbery region (
Tg + 50 °C);
φ is the front factor, which is unity for ideal rubbers;
R is the gas constant and
T is the absolute temperature.
20 It should be noted that this equation is applicable to a polymer network that has a rubbery plateau region. So it is strictly valid only for lightly crosslinked materials and therefore is used only to qualitatively compare the level of crosslinking in the cast resins.
32
Compared to BMI/BADCy, the higher crosslinking density of Boz/BMI/BADCy led to a higher Tg. The formation of a more crosslinked structure, and more rigid and stable triazine rings in the crosslinking network will increase the Tg and the retention of the storage modulus of the terpolymers at high temperature. Therefore, BMI/BADCy modified by Boz containing allyl groups has a higher initial storage modulus compared to BMI/BADCy; and BBz2 exhibits better thermal stability at high temperatures than BBz.
Thermal properties of the ternary blends
The derivative curves of the TGA thermograms for the terpolymers reveal a great deal of information about the network structure of polybenzoxazine.33,34 In this work, we attempted to increase its crosslink density by adding Boz containing allyl groups. In an effort to explore the resulting improvement in thermal stability, the TGA thermograms and their derivative curves for the copolymers were obtained, and are presented in Fig. 9 and 10. The results for the decomposition temperatures and char yields of the copolymers were collected and are described in Table 2. As can be seen from Fig. 10, different from an observable weight loss peak around 410 °C on the differential curve of the Boz/BMI/BADCy systems, there was no obvious weight loss of Boz/BMI/BADCy under 390 °C. In Table 2, the Td5 of BMI/BADCy is 441 °C, while that of BBz2 is 426 °C. The char yield at 800 °C of BMI/BADCy is 35.8%, while that of BBz2 is 40.4%. The better thermal stability of BMI/BADCy compared to BBz2 may be attributed to the excellent thermal stability of BMI/BADCy, and more triazine rings in BMI/BADCy; the addition of Boz will not significantly sacrifice the thermal resistance of BMI/BADCy. However, the thermal stability of the BBz systems may be attributed to the increased cross-linking degree of the Boz/BMI/BADCy blends which results from Boz undergoing ring-opening polymerization to form polybenzoxazine, the allyl groups reacting with the BMI/CE resins and the formation of the phenolic hydroxyl group which increased the interactions between molecules.
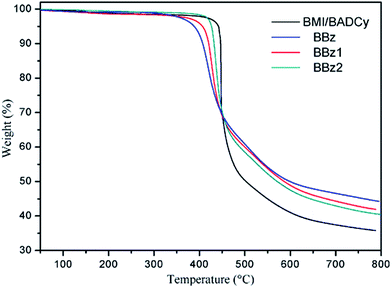 |
| Fig. 9 TGA curves of the BMI/BADCy resin and ternary blends. | |
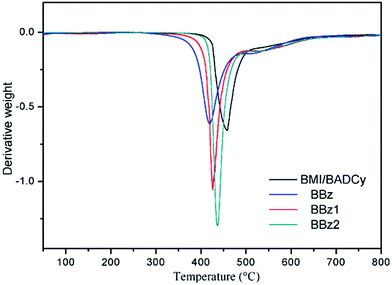 |
| Fig. 10 DTG curves of the BMI/BADCy resin and ternary blends. | |
Dielectric properties of the ternary blends
The dielectric constant and dielectric loss factor of the BMI/BADCy resin and other modified systems with different Boz are shown in Fig. 11 and 12, respectively. It can be seen that the dielectric constant values and the dielectric loss factor of the Boz/BMI/BADCy blends are smaller than BMI/BADCy in the frequency band from 10−1 to 106 Hz. It indicates that the Boz/BMI/BADCy terpolymers have good dielectric stability.
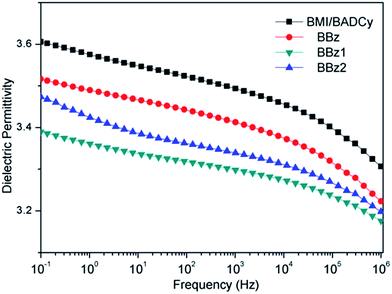 |
| Fig. 11 The dielectric constant of the BMI/BADCy resin and ternary blends. | |
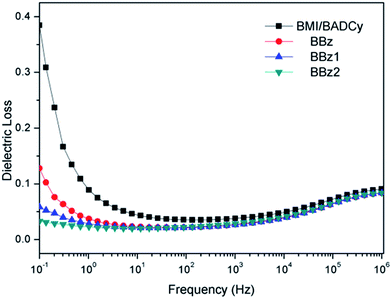 |
| Fig. 12 The dielectric loss factor of the BMI/BADCy resin and ternary blends. | |
It is known to all that polybenzoxazine possesses a lower electrical capacitance than other thermosetting materials but is also less sensitive to changes in frequency.20 As Fig. 11 and 12 show, it can be seen that the dielectric constant values and the dielectric loss factor of the BBz system are bigger than those of BBz1 and BBz2, but smaller than that of BMI/BADCy. The dielectric constant value and the dielectric loss factor of BBz2 reached minimum values of 3.17 and 0.083 at 106 Hz, respectively. In addition, it can be seen that BBz2 possesses good dielectric stability over the testing frequency band from 10−1 to 106 Hz. There are three possible reasons. Firstly, Boz has a lower dielectric constant than the copolymer of BMI/BADCy, which can cause the dielectric constant of the composites to become small. Secondly, it was due to the triazine rings and diphenyl ether with a low dielectric constant and dielectric loss factor. Furthermore, the dielectric constant could be reduced by the decrease in the dipole polarization in the higher crosslinked system. The dielectric loss factor is also related to the crosslinking density based on its effect on the reduction in dipole polarization. But at high frequency there is no time for the polarization of dipoles. So the dielectric loss factor was insensitive to the crosslinking density at high frequency, which led to no obvious difference in the measured values of the dielectric loss factor. Shieh et al. reported that the high crosslinking density and high viscosity during the final curing stage of cyanate ester hindered the mobility of residual cyanate groups.35 However, according to Shieh’s conclusion, the network structure with a high crosslinking density led to more free volume in the ternary blends, which reduced the dielectric constant of the Boz/BMI/BADCy blends.36,37 Therefore, both the dielectric constant and loss factor of the Boz/BMI/BADCy blends decrease compared with BMI/BADCy. It can be concluded that BBz2 has good dielectric properties and retains good dielectric stability.
Conclusion
A kind of high-performance polymer composite has been fabricated using a Boz/BMI/BADCy resin, in which a BMI/BADCy resin was modified with Boz containing allyl groups in order to improve its dielectric, thermal, and mechanical properties and the cross-linking degree after curing. The incorporation of Boz can catalyze the reaction of the BMI/BADCy resin and react with the BMI/BADCy resin. BBz2 shows the maximum impact strength and flexural strength compared with BMI/BADCy, BBz and BBz1, which are 54% and 41% higher than that of the BMI/BADCy resin, respectively. The Tg value of BBz2 is 268 °C. BBz2 still shows good thermal resistance, and the addition of Boz containing allyl groups does not significantly sacrifice the thermal resistance of the BMI/BADCy resin. Also, allyl groups can improve the dielectric properties of the BMI/BADCy resin. The dielectric constant values and the dielectric loss factor of BBz2 reached minimum values of 3.17 and 0.083 at 106 Hz. In addition, the SEM analysis shows that BBz2 has the distinct characteristic of a ductile fracture. The outstanding integrated properties of the BBz2 blend can be attributed to Mannich bridge networks and the network structure with a high crosslinking density formed in the BBz2 blends.
Acknowledgements
This work was financially supported by the National Science Foundation of China (No. 51407134), China Postdoctoral Science Foundation (No. 2014M562412), China Postdoctoral Science Special Foundation (No. 2015T81028) and the graduate starting seed fund of Northwestern Polytechnical University (No. Z2014175).
References
- Z. Fang, L. Liu, A. Gu, X. Wang and Z. Guo, Polym. Adv. Technol., 2009, 20, 849–856 CrossRef CAS.
- K. Barrett, SAMPE J., 1989, 25, 17–20 CAS.
- D. Zhuo, A. Gu, G. Liang, J.-t. Hu, L. Yuan and X. Chen, J. Mater. Chem., 2011, 21, 6584–6594 RSC.
- H. Jiang, R. Wang, S. Farhan and S. Zheng, J. Appl. Polym. Sci., 2015, 132 CAS.
- B. Sun, G. Liang, A. Gu and L. Yuan, Ind. Eng. Chem. Res., 2013, 52, 5054–5065 CrossRef CAS.
- M. S. Lakshmi and B. Reddy, Eur. Polym. J., 2002, 38, 795–801 CrossRef.
- G. Liang and M. Zhang, J. Appl. Polym. Sci., 2002, 85, 2377–2381 CrossRef CAS.
- A. Chaplin, I. Hamerton, B. J. Howlin and J. M. Barton, Macromolecules, 1994, 27, 4927–4935 CrossRef CAS.
- H. Tang, W. Li, X. Fan, X. Chen, Z. Shen and Q. Zhou, Polymer, 2009, 50, 1414–1422 CrossRef CAS.
- J.-G. Chen, C.-Y. Chen, C.-G. Wu and K.-C. Ho, J. Phys. Chem. C, 2010, 114, 13832–13837 CAS.
- C.-S. Wang, T.-S. Leu and K.-R. Hsu, Polymer, 1998, 39, 2921–2927 CrossRef CAS.
- H. Cao, R. Xu and D. Yu, J. Appl. Polym. Sci., 2008, 109, 3114–3121 CrossRef CAS.
- L. Guozheng, G. Aijuan and L. Liwen, J. Adv. Mater., 1996, 27, 61–64 CAS.
- Z. Wang, J. Zhao, Q. Ran, R. Zhu and Y. Gu, React. Funct. Polym., 2013, 73, 668–673 CrossRef CAS.
- Y. Wang, K. Kou, L. Zhuo, H. Chen, Y. Zhang and G. Wu, J. Polym. Res., 2015, 22, 1–8 CrossRef.
- Y. Wang, K. Kou, G. Wu, A. Feng and L. Zhuo, RSC Adv., 2015, 5, 58821–58831 RSC.
- C. Sawaryn, K. Landfester and A. Taden, Macromolecules, 2011, 44, 7668–7674 CrossRef CAS.
- M. G. Mohamed, K.-C. Hsu and S.-W. Kuo, Polym. Chem., 2015, 6, 2423–2433 RSC.
- Y.-C. Su, S.-W. Kuo, D.-R. Yei, H. Xu and F.-C. Chang, Polymer, 2003, 44, 2187–2191 CrossRef CAS.
- H. Ishida and D. J. Allen, J. Polym. Sci., Part B: Polym. Phys., 1996, 34, 1019–1030 CrossRef CAS.
- T. Agag and T. Takeichi, Macromolecules, 2003, 36, 6010–6017 CrossRef CAS.
- Y. Wang, K. Kou, G. Wu, L. Zhuo, J. Li and Y. Zhang, Polymer, 2015, 77, 354–360 CrossRef CAS.
- X. Ning and H. Ishida, J. Polym. Sci., Part A: Polym. Chem., 1994, 32, 1121–1129 CrossRef CAS.
- C. Gouri, C. R. Nair and R. Ramaswamy, High Perform. Polym., 2000, 12, 497–514 CrossRef CAS.
- X. Liu, Y. Yu and S. Li, Polymer, 2006, 47, 3767–3773 CrossRef CAS.
- A. Gu and G. Liang, Polym.-Plast. Technol. Eng., 1997, 36, 681–694 CrossRef CAS.
- C. Reghunadhan Nair, T. Francis, T. Vijayan and K. Krishnan, J. Appl. Polym. Sci., 1999, 74, 2737–2746 CrossRef.
- C. H. Lin, S. J. Huang, P. J. Wang, H. T. Lin and S. A. Dai, Macromolecules, 2012, 45, 7461–7466 CrossRef CAS.
- P. Musto, M. Abbate, G. Ragosta and G. Scarinzi, Polymer, 2007, 48, 3703–3716 CrossRef CAS.
- L. R. G. Treloar, The physics of rubber elasticity, Oxford university press, 1975 Search PubMed.
- M. Rubinstein and R. H. Colby, Polymer physics, OUP Oxford, 2003 Search PubMed.
- H. Ishida and D. J. Allen, Polymer, 1996, 37, 4487–4495 CrossRef CAS.
- K. Hemvichian and H. Ishida, Polymer, 2002, 43, 4391–4402 CrossRef CAS.
- K. Hemvichian, A. Laobuthee, S. Chirachanchai and H. Ishida, Polym. Degrad. Stab., 2002, 76, 1–15 CrossRef CAS.
- J. Y. Shieh, S. P. Yang, M. F. Wu and C. S. Wang, J. Polym. Sci., Part A: Polym. Chem., 2004, 42, 2589–2600 CrossRef CAS.
- G. Hougham, G. Tesoro and J. Shaw, Macromolecules, 1994, 27, 3642–3649 CrossRef CAS.
- C. H. Lin, Z. R. Jiang and C. S. Wang, J. Polym. Sci., Part A: Polym. Chem., 2002, 40, 4084–4097 CrossRef CAS.
|
This journal is © The Royal Society of Chemistry 2015 |