DOI:
10.1039/C5RA19341A
(Paper)
RSC Adv., 2015,
5, 94903-94908
Phenothiazine based sensor for naked-eye detection and bioimaging of Hg(II) and F− ions†
Received
19th September 2015
, Accepted 27th October 2015
First published on 29th October 2015
Abstract
The design and development of new phenothiazine-based fluorescent probes, which display a selective fluorescence response to mercury and fluoride ions, were described. The probe is the first example that facilitates the detection of Hg2+ at nanomolar concentrations. The sensitivity of probe P-1 to Hg2+ and F− was demonstrated in living cells, and cell toxicity assays revealed that probe P-1 can be used for selective imaging of Hg2+ and F− in living cells. Moreover, the simple probe design presented here may contribute to the development of more efficient and more useful dual-mode probes.
1. Introduction
The development of new probes capable of selective and sensitive detection of toxic heavy metal ions has attracted considerable attention because of their wide use in biological, analytical and environmental fields.1 One of the most toxic heavy metal elements, Hg2+ is considered to be highly dangerous, because both elemental and ionic mercury can be converted into methyl mercury by bacteria in the environment, which subsequently bioaccumulates through the food chain.2a It leads to the dysfunction of the brain, kidney, stomach, and central nervous system because of the thiophilic nature in proteins and enzymes.2b Mercury pollution has sparked interest in the design of new tactics to monitor Hg2+ in biological and environmental samples.3 The Environmental Protection Agency (EPA) standard for the maximum allowable level of inorganic Hg2+ in drinking water is 2 ppb.4 Several optical methods using organic fluorophores,5 nanoparticles,6 have been developed for simple and rapid detection of Hg2+. Among the optical sensors, the fluorescence approach is simple and sensitive for the detection of Hg2+ and also for rapid tracking in biological, toxicological and other environments.7–10
Anions are ubiquitous and play a crucial role as co-factors and enzyme substrates in biosystems. Apart from these anion channels are known to be involved in cell migration, cell proliferation and volume homeostasis.11 On the other hand certain anions such as fluoride, phosphate, nitrate, cyanide are convicted as pollutants.12 Among these pollutants fluoride is somewhat unique in that, it is used for clinical treatments such as osteoporosis, orthodontics, enamel demineralisation and fluoride toxicity. Nevertheless, the excessive level entry of fluoride into the body leads to fluorosis and urolithiasis. Fluoride toxicity generally leads to the enhanced bone density and cancer.13,14 The acquaintance of fluoride ions in the body is generally monitored by its concentration in urine.15–18 Due to these adverse and beneficial effects of fluoride the development of sensor for fluoride has fascinated many researchers.19–21 The homeostasis of fluoride ions demands designing a suitable sensor for fluoride. In the complexation mode with fluorophore containing thiosemicarbazone have been described so far explain basic mode of NS boding with metal ions.22
Phenothiazine and its derivatives are used in different areas such as dyes, probes, pharmaceuticals and electrochemistry.23 Phenothiazine can provide with strong fluorescence, structural regulation and good hole transport capacity. However only few metal ion fluorescent probes based on phenothiazine.24 In this work we have developed a phenothiazine based Hg2+ sensor for nano molar concentration. Moreover, detecting of mercury and fluoride based on one fluorescence sensor with imine functionality is more convenient also great significance to cell imaging as it is a reversible probe.
2. Results and discussion
To a mixture of thiosemicarbazide (1 mmol) and dry ethanol (60 mL), 10-hexyl-10H-phenothiazine-3-carbaldehyde 3 (1 mmol) is added (1 mmol) under nitrogen atmosphere. The reaction mixture was refluxed at 70 °C for 24 h. After the reaction was completed, the solvent was removed under reduced pressure to give the crude product, which was recrystallized from hot methanol to afford pure compound of P-1 as yellow powder in good yield (Scheme 1). P-1 was characterized by 1H-NMR, and ESI-MS (ESI Fig. S1 and S2†).
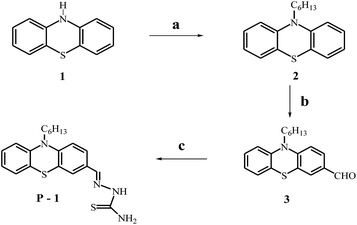 |
| Scheme 1 Synthesis of probe P-1 (a) 1-hexyl bromide, NaH, DMF, N2 atm, reflux 12 h. (b) DMF/POCl3 (1 : 1), DCE, N2 atm, reflux 6 h. (c) Thiosemicarbazide, MeOH, N2 atm, 70 °C, 24 h. | |
The changes in the photonic properties with the addition of metal ions like Zn2+, Mg2+, Co2+, Cu2+, Ba2+, Al3+, Mn2+, Li3+, Pb2+, Hg2+, Cd2+, Fe3+, Ni2+, Ca2+, Na+ and K+ (ESI Fig. S3†). The absorption spectra of the probe P-1 shows 3 characteristic peaks around 230, 326, 410 nm. The incremental addition of Hg2+ leads to a gradual enhancement of the absorption intensity and hence the probe P-1 is utilised as a sensor for Hg2+. Upon addition of Hg2+ (0–2 equiv.) the probe P-1 exhibited a decrease in the band at 326 nm and a red shift of 410 nm peak to 450 nm which indicates the presence of ground state interactions of probe and Hg2+ ion (Fig. 1). The probe also exhibited two isosbestic points which clearly indicate the presence of 2 species P-1 and [P-1 + Hg2+].
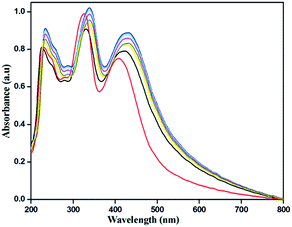 |
| Fig. 1 UV-vis spectrum of probe P-1 (10 μM) upon addition of Hg2+ (0–2 equiv.) in ethanol–water (7 : 3) mixture. | |
In general the fluorescence based detection of analyte is preferred over the UV-vis absorption method because of the capability of detecting even very low concentration of analyte. The fluorescent properties of the probe P-1 in the presence of various metal ions were studied in (ethanol–water 7
:
3). The probe P-1 displayed emission upon excitation at 450 nm. The addition of Hg2+ ion culminated in an intense emission band quenching at 557 nm (Fig. 2).
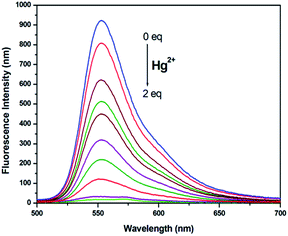 |
| Fig. 2 Fluorescence emission spectrum of probe P-1 (10 μM) upon addition of Hg2+ (λex = 450 nm) (0–2 equiv.) in ethanol–water (7 : 3) mixture. | |
The fluorescence titration of P-1 with 0–10 equivalent of Hg2+ ions gradually quenches the fluorescence intensity at 557 nm whereas addition of other ions like Zn2+, Mg2+, Co2+, Cu2+, Ba2+, Al3+, Mn2+, Li3+, Pb2+, Cd2+, Fe3+, Ni2+, Ca2+, Na+ and K+ did not resulted in any observable fluorescence quenching.
Addition of 2 equiv. of F− makes the quenched fluorescence restore at 557 nm. It is found that fluoride ion also increases the fluorescence intensity of [P-1 + Hg2+] ensemble in concentration dependence. [P-1 + Hg2+] ensemble displays a high sensitivity to fluorine to form the stable species compared to that of other anions (ESI Fig. S4†).
To confirm the stoichiometry of the binding of probe P-1 with Hg2+ ion Job's plots (continuous-variation plots) analysis was carried out. The plot of the absorbance variation at 450 nm against mole fraction which clearly showed the maxima with a mole fraction at 0.6 indicating 1
:
2 stoichiometry and the proposition is further supported by the peak at 969.73 (P-1 + Hg2+) ESI-MS analysis (ESI Fig. S5†). Similarly Job's plots were derived from fluorescence analysis which also conforms to the 1
:
2 binding of P-1 with Hg2+ ions (Fig. 3). The linear plot was obtained when plotting fluorescence intensity vs. concentration of Hg2+ ions indicating that the probe P-1 can be used to determine Hg2+ ion concentration. The binding constant of P-1 with Hg2+ was calculated and it is found to be 3.4 × 103 M−1.25
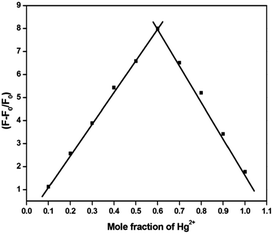 |
| Fig. 3 The Job's plot of mole fraction of Hg2+ vs. fluorescence intensity at λex = 557 nm. | |
The titration profile shows a steady decrease of fluorescence intensity with increasing concentration of Hg2+, after addition of 2.3 equivalents it reaches the saturation level. For the practical quantitative detection, fluorescence spectral changes should vary linearly with the concentration of Hg2+. To test this, we carried out fluorescence titration studies of P-1 (0.2 μM) with Hg2+. A linear response of the fluorescence intensity as a function of [Hg2+] was observed from 20 nM to 0.33 μM (R2 = 0.998). The detection limit (DL) was calculated from this, the lower detection limit was found to be 1.5 × 10−8 M.26
From the absorption and fluorometric results it is revealed that the probe P-1 is very selective and sensitive over other metal ions. The fluorescence response of probe with other metal ions have been investigated, only Hg2+ shows quenching in the intensity of probe over other metal ions (Fig. 4). This may be ascribed to an ICT (internal charge transfer) process, the N-hexyl phenothiazine is an strong electron-donating group when thiourea moieties were complexed with Hg2+, weaker electron-withdrawing effect will inhibit the original ICT (internal charge transfer) process from the N-hexyl phenothiazine group to the thiourea moieties. The emission is almost quenched by addition of Hg2+ and recovered again with the addition of F− (Fig. 5). All properties mentioned above indicates that the capability of P-1 and [P-1 + Hg2+] ensemble for quantitative detection Hg2+and F− as an “on–off–on” type probe (Fig. 6).
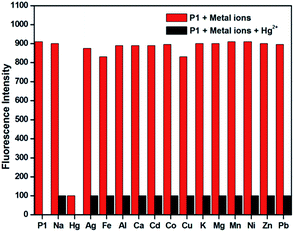 |
| Fig. 4 Fluorescence response of 10 μM P-1 with various metal ions. The red bars represent the addition of the corresponding metal ion to P-1. The black bars represent the change of the emission that occurs upon the subsequent addition of Hg2+ to the above solution. Excitation at 450 nm, (slit width = 5 nm). | |
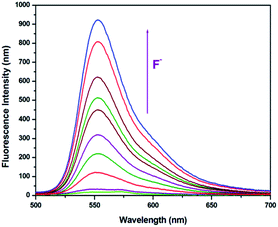 |
| Fig. 5 Fluorescence response of [P-1 + Hg2+] ensemble (10 μM) to F− λex = 450 nm, (slit width = 5 nm). | |
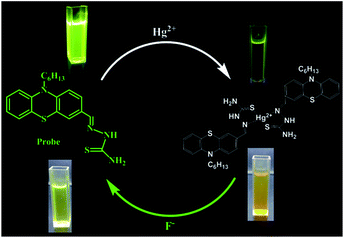 |
| Fig. 6 Plausible mechanism for sensing Hg2+ and F− by P-1. | |
Furthermore, 1H NMR titrations experiment was performed to understand the nature of interactions between the P-1 and the Hg2+ ion, and the selected spectra are given in (Fig. 7). The comparison of the 1H NMR spectra of P-1 and P-1 mixed with 0.5 equivalent of Hg2+ ion suggest that the addition of Hg2+ to the P-1 solution caused a downfield shift in the signal corresponding to –NH (11.33 to 11.97) and N–CH– (7.92 to 7.94) Protons, it shows that Hg2+ is bound to the receptor through coordination of Hg2+ to the lone pair electrons sulphur atom and imine nitrogen.27 The 1H NMR titration, the phenothiazine ring sulphur atom interact with Hg2+ ion, the protons attached with phenothiazine aromatic system usually shift towards down field,24c,28 but here phenothiazine protons Ha (d, 7.48 to 7.47), Hb (d, 8.08 to 7.76) and Hc (s, 7.72 to 7.40) shift to upfield after complex with Hg2+ ion it strongly shows that Hg2+ ion is not interact with phenothiazine ring sulphur atom, and shift may be due to increase the electron donating ability of N-hexyl phenothiazine ring towards semicarbazone after complex with Hg2+ ion and this was attributed to the increase of the electronic density of the aromatic system attached with semicarbazone and caused by upfield shift of the signals.
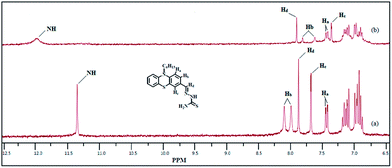 |
| Fig. 7 Partial 1H NMR (300 MHz) spectra of P-1 (a), P-1 + 0.5 equivalent Hg2+ (b) in DMSO-d6. | |
The well-established MTT assay, which is based on mitochondrial dehydrogenase activity of viable cells, was adopted to study cytotoxicity of the probe P-1 at varying dose and time dependent assay which shows that the probe P-1 did not exert any adverse effect on cell viability (ESI Fig. S11 and S12†). However, exposure of HeLa cells to probe + Hg2+ ensemble resulted in a declined in cell viability above a concentration of 20 μM. The effect was more pronounced at higher concentrations and showed an adverse cytotoxic effect in a dose-dependent manner which is in agreement with previous literature reports suggesting cytotoxic and anti-proliferative effects of probe + Hg2+ ensemble on cancer cells.29 The viability of HeLa cells was not influenced by the solvent (DMSO) leading to the conclusion that the observed cytotoxic effect could be attributed to the probe + Hg ensemble formation.
The results obtained in the in vitro cytotoxic assay suggested that, in order to pursue fluorescence imaging studies of P-1 in live cells, it would be prudent to choose a working concentration of 20 μM. Hence, to assess the effectiveness of P-1 as a probe for intracellular detection of Hg2+ by confocal microscopy, HeLa cells were treated with 5 μM of P-1 for 30 min which revealed a green fluorescence in HeLa cells (Fig. 8). Upon incubation with probe followed by Hg2+ striking switch-off fluorescence was observed inside HeLa cells, which indicated the formation of probe + Hg2+ ensemble, as observed earlier in solution studies. The probe + Hg2+ treated cells are further incubated with tetra butyl ammonium fluoride showed the enhanced green fluorescence in cells. DAPI nuclear staining of HeLa cells indicates that the P-1 is not affecting the nucleus of the cells and merged image of DAPI and P-1 treated cells provided that the nuclei of cells are intact. It is significant that the bright field images of DAPI and probe P-1 treated cells did not reveal any morphological changes, which suggest that HeLa cells are viable (Fig. 9). These findings shows that P-1 is biocompatible in nature and can be used for detecting Hg2+ and F− ions in cells rapidly.
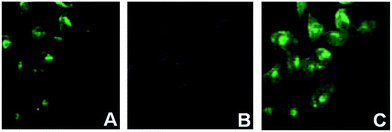 |
| Fig. 8 Bright field image and fluorescence images of HeLa cells. (A) Fluorescence image of HeLa cells incubated with P-1 (5.0 μM) for 3 h at 37 °C. (B) Bright-field image of P-1 treated HeLa cells with Hg2+ (10 μM) (C) fluorescence image of HeLa cells incubated with P-1 + Hg2+ and subsequently treated with (20 μM) F− λex = 450 nm. | |
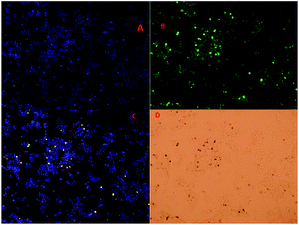 |
| Fig. 9 (A) Fluorescence image of HeLa cells incubated with DAPI for 1 h at 37 °C. (B) Fluorescence image of HeLa cells incubated with P-1 for 1 h at 37 °C. (C) Fluorescence overlaid image of (A) and (B). (D) Bright field image of P-1 treated cells. | |
In order to understand further the absorption and fluorescence behaviour of the P-1 and the Hg2+ complex, we carried out the DFT calculations with B3LYP and 6-311G/LANL2DZ basis sets using Gaussian 09 program.30 From the optimized geometries the TDDFT calculations were carried out using above mentioned sets. Frontier molecular orbitals derived from the optimized geometries, In HOMO is spread over on the whole pi moiety and LUMO on the one of the thiocarbazide unit. In P-1 − Hg2+ HOMO is localized on part of the pi moiety and LUMO is spread on Hg2+ only (Fig. 10). These results clearly shows that the disturbance of internal charge transfer on the appendage of Hg2+ with probe-1.
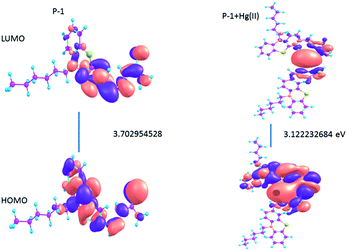 |
| Fig. 10 Frontier molecular orbitals of P-1 and P-1 + Hg2+ obtained from the DFT calculations using Gaussian 09 program. | |
3. Conclusion
We have designed and synthesized a phenothiazine based derivative P-1 as highly sensitive and selective chemosensor for Hg2+. To the best of our knowledge, the probe P-1 is the first example based on phenothiazine that facilitates the detection of Hg2+ at nanomolar concentration. The sensitivity of probe P-1 to Hg2+ and F− was demonstrated in living cells, and cell toxicity assay reveals that probe P-1 can be used for selective imaging of Hg2+ and F− in living cells. Moreover, the simple probe design presented here may contribute to the development of more efficient and more useful dual-mode probes.
4. Experimental methods
The precursor and compounds 2 and 3 were prepared by earlier literature procedures.31
Synthesis of P-1
To a mixture of thiosemicarbazide (1 mmol) and dry ethanol (60 mL) were added 10-hexyl-10H-phenothiazine-3-carbaldehyde 3 (1 mmol) under nitrogen atmosphere. The reaction mixture was refluxed at 70 °C for 24 h. After the reaction was completed, the solvent was removed under reduced pressure to give the crude product, which was recrystallized from hot methanol to afford pure compound P-1 as yellow powder. Yield: 90%; Mp: 191 °C; IR KBr (cm−1): 3422 (NH2), 3259 (–NH, str), 3141 (
C–H), 1599 (NH, bend), 1459 (NH2), 1238 (C
S), 1102 (
C–H, bend); 1H NMR (300 MHz, DMSO-d6): 0.82 (t, 3H); 1.24–1.25 (m, 4H); 1.38 (m, 2H); 1.67 (m, 2H); 3.88 (t, 2H); 6.93–7.05 (m, 2H); 7.12–7.29 (m, 2H); 7.48 (d, 1H); 7.72 (s, 1H); 7.92 (s, 1H); 8.08 (d, 1H); 11.33 (s, 1H); mass (ESI-MS): 385.09.
MTT assay
The cell viability of the probe P-1 were tested against HeLa cell lines using the 3-(4,5-dimethylthiazole-2-yl)-2,5-diphenyl tetrazolium bromide (MTT) assay. The cells were seeded into a 96-well plate and incubated in medium containing the probe P-1 at different concentrations for different time intervals. 100 μL of MTT was added and the plates were incubated at 37 °C for 4 h to allow MTT to form formazan crystals. Intracellular formazan crystals were dissolved by adding 100 μL of DMSO to each well and the plates were shaken for 10 min. The absorbance was read at 570 nm and 630 nm using plate reader. The percentage of survival was calculated using the formula: % survival = [live cell number (test)/live cell number (control)] × 100.
Cell culture and fluorescence imaging
HeLa cells were grown in modified Eagle's medium supplemented with 10% FBS (fetal bovine serum) at 37 °C. The HeLa cells were incubated with DAPI (5.0 μM) in PBS and imaged through the microscope and the DAPI treated cells were incubated with P-1 (5.0 μM in DMSO/H2O (2
:
1, v/v) buffered with PBS, pH = 7.54) and imaged through the fluorescence microscope. After washing with PBS three times to remove the excess of the probe P-1 in the cells, the cells were further incubated with Hg(ClO4)2 (20.0 μM in H2O) for 10 min at 37 °C and imaged with Nikon fluorescence microscope. Then P-1 and Hg(ClO4)2 (20.0 μM in H2O) again incubated with tetra butyl ammonium fluoride and imaged through the fluorescence microscope.
Acknowledgements
This work is supported by the Fast Track Programme for the young scientist of the DST-SERB (No. SR/FT/CS-062/2013).
Notes and references
-
(a) H. N. Kim, W. X. Ren, J. S. Kim and J. Yoon, Chem. Soc. Rev., 2012, 41, 3210 RSC
;
(b) M. Santra, D. Ryu, A. Chatterjee, S.-K. Ko, I. Shin and K. H. Ahn, Chem. Commun., 2009, 2115 RSC
;
(c) E. M. Nolan and S. J. Lippard, Chem. Rev., 2008, 108, 3443 CrossRef CAS PubMed
. -
(a) I. Onyido, A. R. Norris and E. Buncel, Chem. Rev., 2004, 104, 5911 CrossRef CAS PubMed
;
(b) H. H. Harris, I. J. Pickering and G. N. George, Science, 2003, 301, 1203 CrossRef CAS PubMed
. - Z. Guo, S. Park, J. Yoon and I. Shin, Chem. Soc. Rev., 2014, 43, 16 RSC
. - Mercury Update: Impact on Fish Advisories, EPA Fact Sheet EPA-823-F-01-011; EPA, Office of Water, Washington, DC, 2001.
-
(a) W. Shi and H. Ma, Chem. Commun., 2008, 1856 RSC
;
(b) C. Chen, R. Wang, L. Guo, N. Fu, H. Dong and Y. Yuan, Org. Lett., 2011, 13, 1162 CrossRef CAS PubMed
. -
(a) W. Guo, J. Yuan and E. Wang, Chem. Commun., 2009, 3395 RSC
;
(b) D. Liu, W. Qu, W. Chen, W. Zhang, Z. Wang and X. Jiang, Anal. Chem., 2010, 82, 9606 CrossRef CAS PubMed
;
(c) Y. Zhou, J. F. Zhang and J. Yoon, Chem. Rev., 2014, 114, 5511 CrossRef CAS PubMed
. -
(a) X. Zhang, Y. Xiao and X. Qian, Angew. Chem., Int. Ed., 2008, 47, 8025 CrossRef CAS PubMed
;
(b) A. Jana, J. S. Kim, H. S. Jung and P. K. Bharadwaj, Chem. Commun., 2009, 4417 RSC
. - D. T. Quang and J. S. Kim, Chem. Rev., 2010, 110, 6280 CrossRef CAS PubMed
. -
(a) G. Zhang, D. Zhang, S. Yin, X. Yang, Z. Shuai and D. Zhu, Chem. Commun., 2005, 2161 RSC
;
(b) B. Liu and H. Tian, Chem. Commun., 2005, 3156 RSC
. -
(a) Y. Shiraishi, S. Sumiya and T. Hirai, Org. Biomol. Chem., 2010, 8, 1310 RSC
;
(b) J.-S. Wu, I.-C. Hwang, K.-S. Kim and J. S. Kim, Org. Lett., 2007, 9, 907 CrossRef CAS PubMed
;
(c) M. H. Lee, J.-S. Wu, J. W. Lee, J. H. Jung and J. S. Kim, Org. Lett., 2007, 9, 2501 CrossRef CAS PubMed
;
(d) W. Shi and H. Ma, Chem. Commun., 2008, 1856 RSC
. - P. D. Beer and P. A. Gale, Angew. Chem., Int. Ed., 2001, 40, 486 CrossRef CAS
. -
(a) M. Kleerekoper, Endocrinol. Metab. Clin. North Am., 1998, 27, 441 CrossRef CAS PubMed
;
(b) B. D. Gessner, M. Beller, J. P. Middaugh and G. M. Whitford, N. Engl. J. Med., 1994, 330, 95 CrossRef CAS PubMed
. - J. Ekstrand and M. Ehrnebo, J. Occup. Environ. Med., 1983, 25, 745 CAS
. - M. Cametti and K. Rissanen, Chem. Commun., 2009, 2809 RSC
. -
(a) A. M. Brun, A. Harriman, V. Heitz and J.-P. Sauvage, J. Am. Chem. Soc., 1991, 113, 8657 CrossRef CAS
;
(b) J. Strauss and J. Daub, Adv.
Mater., 2002, 14, 1652 CAS
. -
(a) H. Spreitzer and J. Daub, Chem.–Eur. J., 1996, 2, 1150 CrossRef CAS
;
(b) A. Knorr and J. Daub, Angew. Chem., Int. Ed., 1995, 34, 2664 CrossRef CAS
. -
(a) F. Fungo, S. A. Jenekhe and A. J. Bard, Chem. Mater., 2003, 15, 1264 CrossRef CAS
;
(b) M. Y. Jo, S. S. Park and J. H. Kim, Synth. Met., 2012, 162, 70 CrossRef CAS
. -
(a) S. S. Park, Y. S. Won, Y. C. Choi and J. H. Kim, Energy Fuels, 2009, 23, 3732 CrossRef CAS
;
(b) H.-H. Lin, S.-Y. Su and C.-C. Chang, Org. Biomol. Chem., 2009, 7, 2036 RSC
. -
(a) R. Y. Lai, X. Kong, S. A. Jenekhe and A. Bard, J. Am. Chem. Soc., 2003, 125, 12631 CrossRef CAS PubMed
;
(b) N. S. Cho, J. H. Park, S. K. Lee, J. Lee, H. K. Shim, M. J. Park, D. H. Hwang and B. J. Jung, Macromolecules, 2006, 39, 177 CrossRef CAS
;
(c) G. W. Kim, M. J. Cho, Y. J. Yu, Z. H. Kim, J. I. Jin, D. Y. Kim and D. H. Choi, Chem. Mater., 2007, 19, 42 CrossRef CAS
. -
(a) M. Hauck, J. Schonhaber, A. J. Zucchero, K. I. Hardcastle, T. J. J. Muller and U. H. F. Bunz, J. Org. Chem., 2007, 72, 6714 CrossRef CAS PubMed
;
(b) G. Ajaykumar, K. Sreenath and K. R. Gopidas, Dalton Trans., 2009, 1180 RSC
;
(c) S. Y. Su, H.-H. Lin and C.-C. Chang, J. Mater. Chem., 2010, 20, 8653 RSC
;
(d) J. Wu, H. Guo, X. Zhang, T. D. James and J. Zhao, Chem.–Eur. J., 2011, 17, 7632 CrossRef PubMed
;
(e) J. Weng, Q. Mei, B. Zhang, Y. Jiang, B. Tong, Q. Fan, Q. Ling and W. Huang, Analyst, 2013, 138, 6607 RSC
;
(f) G. Sivaraman and D. Chellappa, RSC Adv., 2012, 2, 10605 RSC
;
(g) T. Anand, G. Sivaraman and D. Chellappa, Spectrochim. Acta, Part A, 2014, 123, 18–24 CrossRef CAS PubMed
. -
(a) H. Tian, X. Yang, R. Chen, Y. Pan, L. Li, A. Hagfeldt and L. Sun, Chem. Commun., 2007, 3741 RSC
;
(b) C. Chen, J.-Y. Liao, Z. Chi, B. Xu, X. Zhang, D.-B. Kuang, Y. Zhang, S. Liu and J. Xu, J. Mater. Chem., 2012, 22, 8994 RSC
. -
(a) S. Goswami, A. K. Das and S. Maity, Dalton Trans., 2013, 16259 RSC
;
(b) S. Goswami, S. Maity, A. C. Maity, A. K. Das, B. Pakhira, K. Khanra, N. Bhattacharyya and S. Sarkar, RSC Adv., 2015, 5, 5735 RSC
;
(c) S. Goswami, A. K. Das, A. Manna, A. K. Maity, H.-K. Fun, C. K. Quah and P. Saha, Tetrahedron Lett., 2014, 55, 2633 CrossRef CAS
;
(d) T. Anand, G. Sivaraman, M. Iniya, A. Siva and D. Chellappa, Anal. Chim. Acta, 2015, 876, 1 CrossRef CAS PubMed
. -
(a) L. Gaina, E. Gal and L. Mataranga-Popa, Tetrahedron, 2012, 68, 2465 CrossRef CAS
;
(b) A. Bieliauskasa, V. Martynaitisb and V. Getautis, Tetrahedron, 2012, 68, 3552 CrossRef
. -
(a) J. H. Jia, P. C. Xue and Y. Zhang, Tetrahedron, 2014, 70, 5499 CrossRef CAS
;
(b) X. F. Zhang, X. P. Qiu and R. Lu, Talanta, 2010, 82, 1943 CrossRef CAS PubMed
;
(c) W. Yang, S. Yang, Q. Guo, T. Zhang, K. Wu and Y. Hu, Sens. Actuators, B, 2015, 213, 404 CrossRef CAS
;
(d) S. H. Mashraqui, T. Khan, S. Sundaram and S. Ghadigaonkar, Tetrahedron Lett., 2008, 49, 3739 CrossRef CAS
. - K. A. Conners, Binding Constants, The Measurement of Molecular Complex Stability, Wiley, New York, 1987 Search PubMed
. - M. Shortreed, R. Kopelman, M. Kuhn and B. Hoyland, Anal. Chem., 1996, 68, 1414 CrossRef CAS PubMed
. - A. K. Mahapatra, J. Roy, P. Sahoo, S. K. Mukhopadhyay and A. Chattopadhyay, Org. Biomol. Chem., 2012, 10, 2231 CAS
. - J. Weng, Q. Mei, B. Zhang, Y. Jiang, B. Tong, Q. Fan, Q. Linga and W. Huang, Analyst, 2013, 138, 6607 RSC
. -
(a) G. Chen, Z. Guo, G. Zeng and L. Tang, Analyst, 2015, 140, 5400 RSC
;
(b) P. Mahato, S. Saha, P. Das, H. Agarwal and A. Das, RSC Adv., 2014, 4, 36140 RSC
;
(c) A. R. Chowdhury, P. Ghosh, B. G. Roy, S. K. Mukhopadhyay, P. Mitrae and P. Banerjee, RSC Adv., 2015, 5, 62017 RSC
. - M. J. Risch, G. W. Trucks, H. B. Schlegel, G. E. Scuseria, M. A. Robb, J. R. Cheeseman, G. Scalmani, V. Barone, B. Mennucci, G. A. Petersson, H. Nakatsuji, M. Caricato, X. Li, H. P. Hratchian, A. F. Izmaylov, J. Bloino, G. Zheng, J. L. Sonnenberg, M. Hada, M. Ehara, K. Toyota, R. Fukuda, J. Hasegawa, M. Ishida, T. Nakajima, Y. Honda, O. Kitao, H. Nakai, T. Vreven, J. A. Montgomery Jr, J. E. Peralta, F. Ogliaro, M. Bearpark, J. J. Heyd, E. Brothers, K. N. Kudin, V. N. Staroverov, R. Kobayashi, J. Normand, K. Raghavachari, A. Rendell, J. C. Burant, S. S. Iyengar, J. Tomasi, M. Cossi, N. Rega, J. M. Millam, M. Klene, J. E. Knox, J. B. Cross, V. Bakken, C. Adamo, J. Jaramillo, R. Gomperts, R. E. Stratmann, O. Yazyev, A. J. Austin, R. Cammi, C. Pomelli, J. W. Ochterski, R. L. Martin, K. Morokuma, V. G. Zakrzewski, G. A. Voth, P. Salvador, J. J. Dannenberg, S. Dapprich, A. D. Daniels, O. Farkas, J. B. Foresman, J. V. Ortiz, J. Cioslowski and D. J. Fox, Gaussian 09, Revision A.02, Gaussian, Inc., Wallingford CT, 2009 Search PubMed
. -
(a) J. Choi, D. Kim, B. Lee and J. H. Kim, Bull. Korean Chem. Soc., 2009, 30, 1933 CrossRef CAS
;
(b) J.-H. Park, N. S. Cho, Y. K. Jung, H.-J. Cho, H.-K. Shim, H. Kim and Y. S. Lee, Org. Electron., 2007, 8, 272 CrossRef CAS
.
Footnote |
† Electronic supplementary information (ESI) available. See DOI: 10.1039/c5ra19341a |
|
This journal is © The Royal Society of Chemistry 2015 |