DOI:
10.1039/C5RA18130E
(Paper)
RSC Adv., 2015,
5, 96785-96798
Water-soluble star-shaped brush-like block copolymers: synthesis and application as multicompartment nanoreactors for fabrication of quantum dot colloidal nanocrystal clusters†
Received
5th September 2015
, Accepted 25th October 2015
First published on 28th October 2015
Abstract
A series of novel water-soluble multi-arm star-shaped brush-like block copolymers, composed of poly(ethylene oxide) (PEO) as the main chain, poly(acrylic acid) (PAA) as functional graft chains, and a second PEO block as the shell (i.e., multi-arm star-shaped brush-like block copolymer (PEO-g-PAA)-b-PEO) with different molecular weights and grafting density, were rationally designed and synthesized by a combination of anionic copolymerization and atom transfer radical polymerization (ATRP). The anionic ring-opening copolymerization of ethylene oxide (EO) and ethoxyethyl glycidyl ether (EEGE) was conducted first by using α-cyclodextrin (α-CD) with 18 hydroxyl groups and diphenylmethyl sodium (DPMNa) as co-initiator system. The monomer reactivity ratios for EO and EEGE were also determined: r1(EO) = 1.18 ± 0.03 and r2(EEGE) = 0.79 ± 0.01, respectively. Then the resulting multi-arm star-shaped copolymers of poly(EO-co-EEGE) with hydroxyls as end functional groups were utilized as star-shaped macroinitiators to sequentially initiate the anionic ring-opening polymerization of monomer EO for the second block hydrophilic homopolymer PEO chain grown on the first block poly(EO-co-EEGE) arm end, and then the ethoxyethyl groups of the star-shaped block copolymers of poly(EO-co-EEGE)-b-PEO obtained were removed by hydrolysis. The formed multi-arm star-shaped block copolymers of EO and glycidol (Gly) with multi-pendant hydroxymethyls (star-shaped block copolymer poly(EO-co-Gly)-b-PEO) were then esterified by the reaction of the pendant hydroxyl groups of glycidol units of copolymers with 2-bromoisobutyryl bromide, and the resulting star-shaped brush-like macroinitiators were used to initiate the grafting polymerization of t-butyl acrylate (tBA) by ATRP technique. Finally, the tert-butyl ester groups of PtBA grafting side chains were selectively hydrolyzed in trifluoroacetic acid (TFA) to obtain the water-soluble multi-arm star-shaped brush-like block copolymer (PEO-g-PAA)-b-PEO. The water-soluble star-shaped brush-like (PEO-g-PAA)-b-PEO, which consisted of a functional PAA domain as the core and a hydrophilic PEO domain as the shell, were exploited as polymeric nanoreactors to structure-direct in situ fabrication of CdSe quantum dots (QDs) colloidal nanocrystal clusters composed of primary CdSe nanocrystals as subunits, with the secondary structures of CdSe nanocrystals. Spherical CdSe colloidal nanocrystal clusters were intimately and permanently capped with hydrophilic PEO chains on the surface to render the resulting clusters with high water dispersible property.
Introduction
When two or more different hydrophilic polymer segments are organized into one copolymer architecture, a class of copolymers, double-hydrophilic copolymers are formed.1 Owing to some special properties, such as polarity transformation with changing pH or temperature, interaction with metal ions, solid substrates, colloids and biomolecules, etc., the synthesis of these copolymers has been paid much attention. Typical hydrophilic segments include poly(ethylene oxide) (PEO), poly(acrylic acid) (PAA), poly(vinyl pyridine), poly(N,N′-dimethyl aminoethyl methacrylate), poly(N-isopropylacrylamide), etc.2–5 For hydrophilic PEO and PAA segments: PEO segments are not only hydrophilic, but also nonionic and crystalline, and PAA is a sort of hydrophilic weak polyelectrolyte, in which the degree of ionization is controlled by the pH values and the ionic strength of the aqueous solution,6 can be used for the interaction of PAA segments with metal ions.7–9 Copolymers with different structures comprising such hydrophilic segments have been applied in many fields, such as reverse-assembly,5 stabilization of inorganic particles,10 crystal growth modifiers,11 drug carriers12 and gene therapies.13
Star-shaped polymers comprising more than three linear polymer chains covalently joined to a core have the simplest structure of numerous possible branched topologies.14–17 Owing to their compact architectures (i.e., smaller hydrodynamic volume and radius of gyration), globular shapes, and high concentration of terminal functional groups, compared with linear analogues of identical molar mass, star-shaped polymers exhibit high solubility in common solvents, low viscosity, and modified thermal properties.18–21 As such, star-shaped polymeric architectures provide most of the properties of high molecular weight materials without the solution viscosity penalty of linear polymers of similar molecular weight for potential applications in coatings, additives, drug and gene delivery, and supramolecular science.22–26 In addition, copolymer brushes composed of a flexible linear backbone and densely grafted side chains are a unique class of copolymers with intriguing structures, and can be prepared by different approaches: “grafting onto”, “grafting through” and “grafting from”.27 Due to the distinctive chemical and physical properties originating from their intrinsically complex architectures, copolymer brushes have received much attention to investigate the structure–property relationship27,28 for potential applications in supramolecular science, biomaterials, advanced materials,29 photonic crystals,30 etc.
With the development in controlled/living polymerization techniques, such as living anionic polymerization, controlled radical polymerization (e.g., atom transfer radical polymerization (ATRP)),21,31–33 functional copolymers with well-defined yet complex architectures can be rationally designed and synthesized, such as multicomponent bottlebrush copolymers,34 coil–rod brush copolymers,35 star-like block copolymers,36 four-arm star molecular brushes,37,38 etc. However, it is noteworthy that these copolymers have linear polymeric brush structures, or the number of arms of star molecular brushes was rarely larger than four, or these polymeric architectures are not double-hydrophilic. In order to prepare star-shaped polymeric structures, the arm-first strategy or the core-first strategy was usually used.39,40 In the arm-first method, the linear arms are prepared first, followed by connecting the arms to the core. The connection of the arms is performed by using either a difunctional monomer or a multifunctional terminating agent. When a difunctional monomer was used as a cross-linking agent to form star-shaped structures, the number of arms in these star-shaped polymers is difficult to precisely control, especially for linear copolymer brushes with arms with a strong steric hindrance effect. If star-shaped structures (especially linear copolymer brushes as arms) are synthesized by grafting onto a core having multifunctional terminating groups, the main problem is steric hindrance leading to the difficulty of the reaction between the linear polymer arm end group and the multifunctional coupling agent. Comparing with the arm-first strategy, in the core-first method, star-shaped structures are prepared by using a multifunctional initiator as the core to initiate the growth of arms by the monomer addition. The main difficulty is the choice of multifunctional initiators with precise initiating sites, well-defined structures and combined ability to form star-shaped structures and brush-like structures. So it is still a challenge to assemble more linear copolymer brushes as arms with two functional hydrophilic segments into star-shaped structures.
The macromolecule micelles from linear amphiphilic block copolymers have been used as soft templates to stabilize inorganic nanocrystals for a long time. For example, amphiphilic block copolymers, polystyrene-block-poly(4-vinylpyridine) (PS-b-P4VP), have been utilized as soft templates to fabricate a series of nanocrystals (e.g., Au, Ag and Pt, etc.).41,42 However, using these macromolecular micelles as templates, aggregates of conventional linear amphiphilic block copolymers were formed above their critical micelle concentration,43–45 and their characteristics in a given system depend heavily on the thermodynamic properties of the solvent and on temperature. The shapes and structures of the micelles can change with varying ambient conditions, such as solvent, concentration, pH, temperature and so on.21 In contrast, dendritic or star-shaped macromolecules with compact structures form macromolecular architectures, in which different functional polymeric segments are covalently linked to small molecule cores. So these polymeric architectures are static and structurally stable.46,47
In this contribution, a series of novel water-soluble multi-arm star-shaped brush-like block copolymers, composed of poly(ethylene oxide) (PEO) as the main chain, poly(acrylic acid) (PAA) as functional graft chains, and a second PEO block as the shell (i.e., multi-arm star-shaped brush-like block copolymer (PEO-g-PAA)-b-PEO) with different molecular weights and grafting density, were rationally designed and synthesized via a combination of anionic copolymerization and atom transfer radical polymerization (ATRP). The anionic ring-opening copolymerization of ethylene oxide (EO) and ethoxyethyl glycidyl ether (EEGE) was conducted first by using α-cyclodextrin (α-CD) with 18 hydroxyl groups and diphenylmethyl sodium (DPMNa) as co-initiator system. The monomer reactivity ratios for EO and EEGE were also determined: r1(EO) = 1.18 ± 0.03 and r2(EEGE) = 0.79 ± 0.01, respectively. Then the resulting multi-arm star-shaped copolymers of poly(EO-co-EEGE) with hydroxyls as end functional groups were utilized as star-shaped macroinitiators to sequentially initiate the anionic ring-opening polymerization of monomer EO for the second block hydrophilic homopolymer PEO chain growth on the first block poly(EO-co-EEGE) arm end, and then ethoxyethyl groups of the star-shaped block copolymers of poly(EO-co-EEGE)-b-PEO obtained were removed by hydrolysis. The formed multi-arm star-shaped block copolymers of EO and glycidol (Gly) with multi-pendant hydroxymethyls (star-shaped block copolymer poly(EO-co-Gly)-b-PEO) were then esterified by the reaction of the pendant hydroxyl groups of glycidol units of copolymers with 2-bromoisobutyryl bromide, and the resulting star-shaped brush-like macroinitiators were used to initiate the grafting copolymerization of t-butyl acrylate (tBA) by the ATRP technique. Finally, the tert-butyl ester groups of PtBA as grafting side chains were selectively hydrolyzed in trifluoroacetic acid to obtain the water-soluble multi-arm star-shaped brush-like block copolymer (PEO-g-PAA)-b-PEO. The water-soluble star-shaped brush-like (PEO-g-PAA)-b-PEO, consisting of a functional PAA domain as the core and a hydrophilic PEO domain as the shell, were exploited as polymeric nanoreactors to structure-direct in situ fabrication of CdSe quantum dot (QD) colloidal nanocrystal clusters composed of primary CdSe nanocrystals as subunits, the secondary structures of CdSe nanocrystals. Spherical CdSe colloidal nanocrystal clusters were intimately and permanently capped with hydrophilic PEO chains on the surface to render the resulting clusters with high water dispersible property.
Experimental section
Materials
Ethylene oxide (EO, ≥99.5%), α-cyclodextrin (α-CD, ≥99%), N,N,N′,N′′,N′′-pentamethyldiethylene triamine (PMDETA, 99%), 1-methyl-2-pyrrolidinone (NMP, anhydrous, 99.5%), benzenecarbonyl chloride (≥99.0%) and trifluoroacetic acid (TFA, 99%) were purchased from Sigma-Aldrich, and used as received. CuBr (98%, Sigma-Aldrich) was stirred for 24 h in acetic acid, filtered, washed with ethanol and diethyl ether successively, and dried under vacuum. tert-Butyl acrylate (tBA, Sigma-Aldrich 98%), methyl ethyl ketone (99.9%, Fisher Scientific) and N,N-dimethylformamide (DMF, Fisher Scientific, 99.9%) were dried over CaH2 and distilled under reduced pressure prior to use. Diphenylmethyl sodium (DPMNa) (c = 0.51 M) was prepared according to the literature.48 Tetrahydrofuran (THF, 99%, BDH) was dried by refluxing over sodium, and then distilled from sodium naphthalenide solution. Ethoxyethyl glycidyl ether (EEGE) was prepared by protecting the hydroxyl group of glycidol (Gly) with ethyl vinyl ether according to the previously reported procedure.48 Cadmium acetylacetonate (Cd(acac)2, ≥99.9%), selenium powder (Se, 99.99%) and sodium borohydride (NaBH4, ≥99%) were purchased from Sigma-Aldrich and used as starting materials without further purification. All other reagents were purified by common purification procedures.
Characterization
Molecular weights of copolymers were characterized by gel permeation chromatography (GPC, Agilent 1100) equipped with a G1310A pump, a G1362A refractive detector and a G1314A variable wavelength detector. THF was used as the mobile phase at a flow rate of 1.0 mL min−1 at 35 °C. One LP 5 μm gel column and two 5 μm gel LP mixed bed columns were calibrated with 10 polystyrene (PS) standard samples with molecular weights ranging from 200 to 3 × 106 g mol−1. GPC characterizations of multi-arm star-shaped block copolymers of poly(EO-co-Gly)-b-PEO were performed by gel permeation chromatography (GPC, Agilent 1100) equipped with a G1315A diode-array detector. Aqueous NaNO3 (0.1 M) was used as the mobile phase at 40 °C with an elution rate of 0.5 mL min−1. Three TSK-gel PW columns in series (bead size: 6, 13, 13 μm; pore size: 200 Å, greater than 1000 Å, less than 100–1000 Å; molecular range: 0–5 × 104, 5 × 104 to 8 × 106, (5–8) × 106 g mol−1, respectively) were calibrated by standard samples (linear PEO). 1H nuclear magnetic resonance (1H-NMR) spectra were obtained using a Bruker 400 MHz spectrometer with the solvent resonances for tetramethylsilane (TMS) as the internal standard. CDCl3 and methanol-d4 (CD3OD) were used as the solvent. FTIR spectra were obtained on a Magna-550 Fourier transform infrared spectrometer. The morphology of star-shaped brush-like (PEO-g-PAA)-b-PEO polymeric nanoreactors and the resulting colloidal CdSe nanocrystal clusters were characterized by a transmission electron microscope (JEOL 1200EX TEM; operated at 80 kV). TEM samples of template copolymers were prepared by applying a drop of star-shaped brush-like block copolymer (PEO-g-PAA)-b-PEO DMF solution (∼5 μL at c = 1 mg mL−1) onto a carbon coated copper TEM grid (300 mesh) and allowing DMF to evaporate in a vacuum oven at room temperature. Then the copolymer samples were stained with uranyl acetate. A droplet of freshly prepared saturated uranyl acetate aqueous solution (∼20 μL) was deposited onto dried TEM samples.49,50 The excess solution was removed using a filter paper after 5 min. For TEM characterization of the star-shaped brush-like block copolymer (PEO-g-PtBA)-b-PEO, TEM samples were prepared by using a drop of star-shaped copolymer dichloromethane (CH2Cl2) solution (c = 1 mg mL−1) onto a carbon-coated copper TEM grid (300 mesh) and leaving CH2Cl2 to completely evaporate at room temperature, and then the TEM grid was exposed to vapors of ruthenium tetroxide (RuO4) with which the PEO and PtBA blocks were stained.51–53 Morphologies of the multi-arm star-shaped brush-like block copolymer (PEO-g-PAA)-b-PEO were measured by atomic force microscopy (AFM, Bruker Dimension 3000) in the tapping mode. AFM characterization samples were prepared by spin-coating the DMF solution (c = 1 mg mL−1) on a silicon substrate at 2000 rpm for 1 min. Dynamic light scattering (DLS) data was acquired by a laser light scattering spectrometer (Malvern Autosizer 4700) at 25 °C. The crystalline structures of samples were measured by X-ray diffraction (XRD; SCINTAG XDS-2000, Cu Kα radiation). The energy dispersive spectroscopy (EDS) microanalysis of the samples was conducted by field emission scanning electron microscopy (FE-SEM; FEI Quanta 250). The absorption spectra were recorded with a UV-Vis spectrometer (UV-2600, Shimadzu). The emission spectra were obtained by a spectrofluorophotometer (RF-5301 PC, Shimadzu), and all samples were excited at λex = 350 nm.
Synthesis of the star-shaped copolymers poly(EO-co-EEGE) based on α-CD
The copolymerization of two monomers (EO and EEGE) was carried out in a stainless steel kettle, α-CD with 18 hydroxyl groups and DPMNa as co-initiator system. In a typical process for the preparation of multi-arm star-shaped copolymers, a 200 mL stainless steel kettle was vacuumed at 60 °C for 5 h, and then cooled to −30 °C. α-CD (0.1 g, 0.1 mmol), vacuum-dried at 80 °C overnight, was dissolved in 60 mL anhydrous mixed solvent of 1-methyl-2-pyrrolidinone (NMP) and THF (v/v = 2/1), and then a THF DPMNa solution (1.8 mL, 0.51 M) was slowly introduced into the reaction system. With alkoxide formation under stirring, the color of the homogeneous initiator system was changed to yellow. After that, the initiator solution was added into the reaction kettle, sequentially, the two monomers: EO (22.0 g, 0.5 mol) and EEGE (14.6 g, 0.1 mol) were introduced. The anionic polymerization reaction was allowed to continue at 60 °C for 48 h, and then the anionic polymerization was terminated by the addition of 2 mL acidified methanol. All the solvents and un-reacted monomers were removed by distillation under reduced pressure. The crude copolymers were dissolved in 100 mL dichloromethane, and then washed with DI water. After removal of dichloromethane solvent, transparent and yellowy viscous multi-arm star-shaped copolymer poly(EO-co-EEGE) was obtained. The molar ratio of EO to EEGE in the star-shaped copolymers can be well tuned by adjusting the feed molar ratio of EO to EEGE during the anionic copolymerization process. In addition, samples with different molecular weight can be prepared by changing the molar ratio of initiator to monomers.
Measurement of reactivity ratios of the two monomers: EO and EEGE54
Reactivity ratios of the two monomers (EO and EEGE) were determined according to the YBR method during the anionic copolymerization.54 The anionic copolymerization of two monomers (EO and EEGE) with different feed molar ratio was performed under the same reaction conditions as for the synthesis of the multi-arm star-shaped copolymers poly(EO-co-EEGE). The anionic copolymerization reactions were stopped at below 10% monomer conversion. After copolymerization reaction for 1 h, all the solvents and residual monomers were removed by vacuum distillation, all the products were purified and dried, then structure and composition were determined by 1H NMR. The reactivity ratios were calculated by eqn (1): |
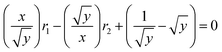 | (1) |
where x is the feed molar ratio of EO to EEGE, y is the molar ratio of EO to EEGE in the resulting copolymer. r1 and r2 are reactivity ratios of EO and EEGE, respectively.
Synthesis of star-shaped block copolymers poly(EO-co-EEGE)-b-PEO
Similar to the synthesis procedure for star-shaped copolymers poly(EO-co-EEGE), multi-arm star-shaped block copolymers poly(EO-co-EEGE)-b-PEO were prepared by sequential anionic polymerization, star-shaped copolymers poly(EO-co-EEGE) with end hydroxyl groups and DPMNa as co-initiator system. The anionic polymerization of monomer EO was carried out in stainless steel kettle. In a typical polymerization process, a 200 mL stainless steel kettle was dried at 60 °C for 5 h under vacuum, and then cooled to −30 °C. Vacuum-dried multi-arm star-shaped poly(EO-co-EEGE) (5.0 g) was dissolved in 50 mL anhydrous DMSO and THF (v/v = 3/2), and then a THF DPMNa solution (2.4 mL) was injected into the solution to form the initiator system. Then the initiator solution was added into the reaction kettle, sequentially, EO monomer (5.0 g, 0.11 mol) was injected into the polymerization reaction system. Under stirring, the polymerization reaction was carried out at 60 °C for 48 h. Then the polymerization reaction was stopped by the addition of 1 mL acidified methanol. After all the solvents were removed by distillation under reduced pressure, the crude copolymers were dissolved in 20 mL dichloromethane, and then precipitated in cold diethyl ether. The chain length of PEO can be well controlled by adjusting the molar ratio of EO to end hydroxyl groups of macroinitiator star-shaped copolymers poly(EO-co-EEGE) during the anionic polymerization process.
End-capping reaction of star-shaped block copolymers poly(EO-co-EEGE)-b-PEO
The end hydroxyl groups of multi-arm star-shaped block copolymers poly(EO-co-EEGE)-b-PEO were end-capped by the reaction between hydroxyl groups and benzenecarbonyl chloride as end-capping reagent. The typical end-capping reaction procedure was as follows: purified multi-arm star-shaped block copolymer poly(EO-co-EEGE)-b-PEO (4.0 g) was added to 50 mL anhydrous CH2Cl2, and then 1 mL triethylamine and benzenecarbonyl chloride (2.0 g) were introduced into the reaction system at room temperature under stirring. The end-capping reaction solution was stirred for 24 h, and then precipitated in cold diethyl ether. The product was purified twice by dissolution/precipitation with CH2Cl2–diethyl ether. The precipitate was separated and dried under vacuum at 50 °C.
Preparation of multi-arm star-shaped block copolymers poly(EO-co-Gly)-b-PEO by hydrolysis of the ethoxyethyl groups of poly(EO-co-EEGE) arms
The cleavage reaction of ethoxyethyl groups was carried out according to the literature.55 Multi-arm star-shaped block copolymer poly(EO-co-EEGE)-b-PEO (2.0 g) was added into 20 mL concentrated hydrochloric acid. The reaction system solution was stirred at room temperature for 2 h. The pH of the reaction solution was adjusted to 3.0 by addition of potassium hydroxide aqueous solution (5.0 M). The product was extracted with CH2Cl2 (100 mL × 2), and then the organic layer was dried over anhydrous MgSO4. All the solvents were removed under reduced pressure, the crude product was dissolved in water and purified by dialysis. The purified aqueous solution was dried under vacuum at 80 °C. Transparent viscous multi-arm star-shaped block copolymers poly(EO-co-Gly)-b-PEO were obtained.
Synthesis of macroinitiator multi-arm star-shaped block copolymer poly(EO-co-BiBGE)-b-PEO
Multi-arm star-shaped block copolymer poly(EO-co-Gly)-b-PEO (1.0 g) was dissolved in 50 mL of anhydrous NMP, then 5.2 mL of 2-bromoisobutyryl bromide was added dropwise at 0 °C for 30 min under vigorous stirring. The reaction system was stirred for 2 h at 0 °C, followed by stirring at room temperature for 24 h. After that, NMP solvent was removed by distillation under reduced pressure. The residue was dissolved in CH2Cl2, and washed with NaOH aqueous solution (1 mol L−1), HCl aqueous solution (1 mol L−1), and DI water, respectively. After the solvent was removed by distillation under vacuum, the final sample multi-arm star-shaped block copolymer poly(EO-co-2-bromoisobutyryloxyglycidyl ether)-b-PEO (poly(EO-co-BiBGE)-b-PEO) was dried under vacuum at 50 °C for 5 h.
Preparation of star-shaped brush-like block copolymer (PEO-g-PtBA)-b-PEO by ATRP
ATRP of tert-butyl acrylate (tBA) was performed using multi-arm star-shaped block copolymer poly(EO-co-BiBGE)-b-PEO as a macroinitiator possessing side initiation sites. In a typical polymerization process, an ampoule charged with CuBr (0.1415 g), PMDETA (0.3414 g), multi-arm star-shaped macroinitiator (0.2 g), tBA (46.8 mL), and 46 mL methyl ethyl ketone was degassed by three freeze–pump–thaw cycles in liquid nitrogen, then sealed and placed in an oil bath at 60 °C. The ampoule was taken out from the oil bath and dipped in an ice–water bath at different desired times to terminate the polymerization. The solution was then diluted with acetone and passed through a neutral alumina column to remove the catalyst, and precipitated in a mixture of methanol–water (v/v = 1/1). After filtration, the final product star-shaped brush-like block copolymer (PEO-g-PtBA)-b-PEO was purified by dissolution/precipitation twice with acetone and methanol–water, and dried at 60 °C under vacuum for 12 h.
Preparation of water-soluble star-shaped brush-like block copolymer (PEO-g-PAA)-b-PEO by hydrolysis of the tert-butyl ester groups on side chains
In a typical process, star-shaped brush-like block copolymer (PEO-g-PtBA)-b-PEO (0.5 g) was dissolved in 50 mL CH2Cl2, and then 10 mL trifluoroacetic acid was added. The reaction solution was stirred at room temperature for 24 h. The resulting star-shaped brush-like block copolymer (PEO-g-PAA)-b-PEO was gradually precipitated in CH2Cl2 during the hydrolysis. The final sample was washed with CH2Cl2, and thoroughly dried under vacuum at 60 °C for 12 h.
Preparation of multicompartment polymeric nanoreactors of water-soluble multi-arm star-shaped brush-like block copolymers of (PEO-g-PAA)-b-PEO
10 mg of multi-arm star-shaped brush-like block copolymer (PEO-g-PAA)-b-PEO was dissolved in 10 mL anhydrous DMF, a good solvent for both PAA and PEO segments (c = 1 mg mL−1) at room temperature. The resulting DMF solution was stirred for 2 days.
Fabrication of hybrid inorganic–organic core–shell CdSe QDs colloidal nanocrystal clusters capped with hydrophilic PEO as shell
NaHSe was firstly prepared by reacting sodium borohydride and selenium powder in DI water.56,57 In a typical process, 1 mmol of selenium powder was introduced into 50 mL of DI water in a three-neck flask. After that, 3 mmol of NaBH4 was added into the reaction system under argon, and the reaction was carried out at room temperature for 8 h under argon atmosphere. After that, the solvent was removed under vacuum, and NaHSe was re-dispersed in dry DMF to prepare a DMF solution. After that, hybrid inorganic–organic core–shell CdSe QDs colloidal nanocrystal clusters capped with hydrophilic PEO as shell were fabricated by the reaction of NaHSe and Cd(acac)2. In a typical process, 10 mg star-shaped brush-like block copolymer (PEO-g-PAA)-b-PEO nanoreactors was dissolved in 10 mL DMF at room temperature, followed by the addition of an appropriate amount of precursor Cd(acac)2 that were selectively loaded into the inner PAA template compartment. The molar ratio of acrylic acid (AA) units in the PAA phase to precursors was 1
:
2. After that, an equimolar amount of freshly prepared NaHSe DMF solution was added dropwise at room temperature, the stirring was maintained for 1 h under argon to ensure that all the precursors were well dissolved in the DMF solvent. The reaction system was then slowly heated to the boiling point of DMF and refluxed for 2 h. The resulting CdSe colloidal nanocrystal clusters were washed by ethanol three times, and then dispersed into DI water.
Results and discussion
Synthesis of star-shaped brush-like block copolymer (PEO-g-PAA)-b-PEO template
The procedure for the preparation of star-shaped brush-like block copolymer (PEO-g-PAA)-b-PEO template is described in Scheme 1. Firstly, the multi-arm star-shaped copolymer poly(EO-co-EEGE) was synthesized by anionic copolymerization of EO and EEGE based on an α-CD core. The glycidol (Gly) was protected with ethyl vinyl ether first to form EEGE monomer, and then was copolymerized with EO using a co-initiator system of α-CD and DPMNa. A multi-arm star-shaped copolymer poly(EO-co-EEGE) was formed. In the copolymerization system, DPMNa was utilized as deprotonating agent, which can easily react with hydroxyl groups to form alkoxides (i.e., anionic polymerization initiating sites). α-CD is a cyclic oligosaccharide consisting of six glucose units linked by α-1,4-glycosidic bonds.58,59 The 18 substitutable hydroxyl groups on the outer surface of the α-CD provide the capability of making a core with 18 initiation sites to form 18-arm, star-shaped architectures. A mixture of NMP and THF (v/v: 2/1) was used as the solvent for copolymerization instead of THF to improve the solubility of α-CD and propagating alkoxides. In order to control the anionic polymerization rate, only about 20% hydroxyl groups of α-CD were activated. In addition, owing to low solubility in organic solvent, if a higher percentage of hydroxyl groups were deprotonated, alkoxides would precipitate from the polymerization system. Under such conditions, all of the hydroxyl groups of α-CD could efficiently initiate the copolymerization of EO and EEGE because of the rapid exchange of protons between dormant hydroxyls and propagating alkoxides, and all the arms grew at the same rate.60 Four multi-arm star-shaped copolymers poly(EO-co-EEGE) with different molar ratios of EO and EEGE, and different arm length could be synthesized by adjusting the monomer feed molar ratio and the molar ratio of monomers to initiator as shown in Table 1 (Fig. S1†).
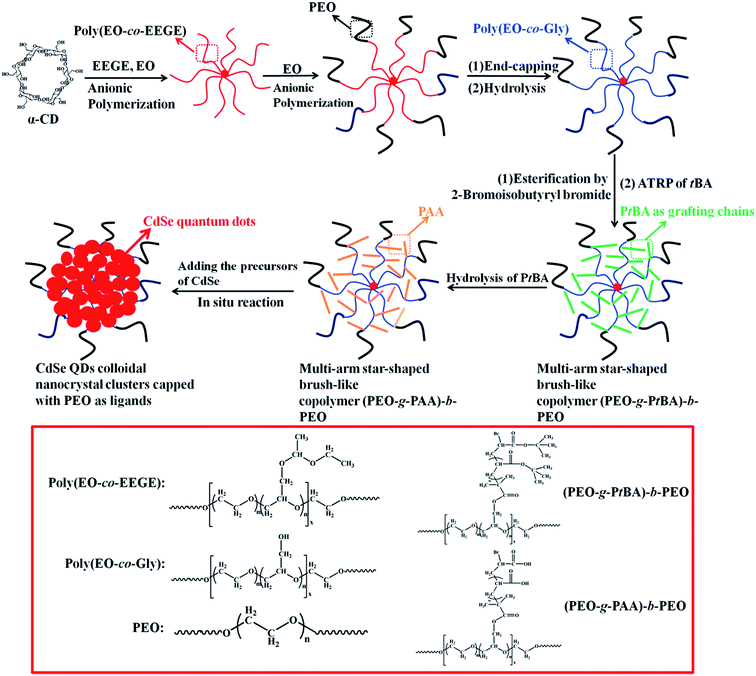 |
| Scheme 1 Schematic illustration of the synthesis of water-soluble multi-arm star-shaped brush-like block copolymer (PEO-g-PAA)-b-PEO used as polymeric nanoreactors for the fabrication of CdSe quantum dot colloidal nanocrystal clusters capped with PEO as shell. | |
Table 1 Summary of multi-arm star-shaped copolymers poly(EO-co-EEGE)
Sample |
Rfa |
Time (h) |
RTb |
Conversionc (%) |
Mn,GPCd (kg mol−1) |
PDIe |
Mn,theoryf (kg mol−1) |
Mn,armg (kg mol−1) |
NEEGEh |
The feed molar ratio of EEGE to EO. The unit molar ratio of EEGE to EO in multi-arm star-shaped copolymers poly(EO-co-EEGE) measured by 1H NMR. Determined by gravimetric method. Number-average molecular weight determined by GPC. The polydispersity (PDI) determined by GPC. The theoretical values of Mn calculated from the monomer conversion and the concentration of initiators. Mn of each poly(EO-co-EEGE) arm calculated from the theoretical values of Mn. The number of EEGE units in multi-arm star-shaped copolymers poly(EO-co-EEGE) calculated by the integration of protons from 1H NMR. |
A-1 |
1/5 |
48 |
1/6.1 |
84.7 |
122 |
1.11 |
310 |
17 |
40 |
A-2 |
1/5 |
48 |
1/6.6 |
89.6 |
192 |
1.08 |
630 |
35 |
77 |
B-1 |
1/10 |
48 |
1/13.3 |
86.5 |
102 |
1.14 |
260 |
14 |
19 |
B-2 |
1/10 |
48 |
1/13.6 |
87.3 |
168 |
1.06 |
560 |
31 |
42 |
Fig. 1 shows a typical 1H NMR spectrum of multi-arm star-shaped copolymers poly(EO-co-EEGE), the quartet at δ = 4.69–4.74 ppm is assigned to the methyl protons (Hc) of EEGE moiety, the doublets at δ = 1.30, 1.29 and the triplet at δ = 1.21, 1.19, 1.18 are assigned to methyl protons of the EEGE moiety (Hd, Ha), the chemical shift at δ = 3.53–3.80 is assigned to protons of the main chain (Hf, Hg, Hh, Hi) and protons of the side chains (Hb, He). The copolymer composition can be calculated by using following equation based on the 1H NMR spectrum:
|
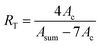 | (2) |
where
RT is the molar ratio of EEGE to EO in the resulting multi-arm star-shaped copolymers poly(EO-
co-EEGE);
Asum and
Ac represent the peak integral area sum of the protons (b + e + f + g + h + i) and the peak integral areas of the methine protons of the EEGE moiety, respectively. The
RT values of four samples are shown in
Table 1, which are nearly equivalent to the monomer feed molar ratio of EEGE to EO. In addition, the average number of protected hydroxyls on each arm could be evaluated by the combination of the theoretical values of molecular weight of each arm and
1H NMR spectrum using the following
eqn (3):
|
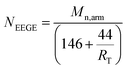 | (3) |
in which
Mn,arm is the theoretical value of molecular weight of each arm, 146 and 44 are the molar masses of EEGE and EO, and
RT is the molar ratio of EEGE units to EO units in copolymers; all the
NEEGE values are listed in
Table 1.
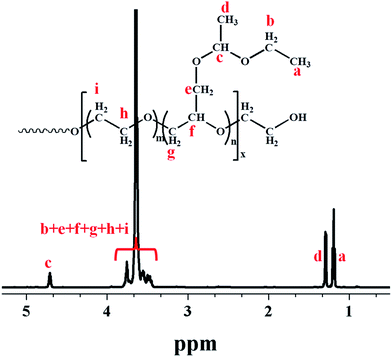 |
| Fig. 1 1H-NMR spectrum of multi-arm star-shaped copolymers poly(EO-co-EEGE) (A-1 sample as shown in Table 1, solvent: CDCl3). | |
Based the theory of copolymerization, the copolymer composition and the distribution of two monomers in the resulting multi-arm star-shaped copolymers poly(EO-co-EEGE), are dependent on the monomer reactivity ratios. So, it is necessary to determine the monomer reactivity ratios. Reactivity ratio values of EO and EEGE may be evaluated by the YBR method based on Table 2 and Fig. 2.54 The monomer reactivity ratios for EO and EEGE are r1(EO) = 1.18 ± 0.03 and r2(EEGE) = 0.79 ± 0.01. Due to r1(EO) > 1, r2(EEGE) < 1, and r1 × r2 ≈ 1, the theory of copolymerization leads the conclusion that the anionic copolymerization of EO and EEGE is close to the ideal nonazeotropic copolymerization. During the copolymerization, the incorporation of two monomers into copolymer chains of star-shaped copolymer poly(EO-co-EEGE) is random, and EEGE units are randomly distributed along the copolymer chains.
Table 2 Data summary for the copolymerization of EO and EEGE
Sample |
Rfa |
Time (h) |
RTb |
Conversionc (%) |
The feed molar ratio of EEGE to EO. The molar ratio of EEGE to EO in multi-arm star-shaped copolymer poly(EO-co-EEGE) calculated based on 1H NMR data. Obtained by a gravimetric method. |
1 |
1/19 |
1 |
1/22.5 |
6.1 |
2 |
1/15 |
1 |
1/17.6 |
5.5 |
3 |
1/8 |
1 |
1/10.1 |
5.8 |
4 |
1/3 |
1 |
1/3.2 |
5.2 |
5 |
1/1 |
1 |
1/1.3 |
3.6 |
6 |
3/1 |
1 |
2.6/1 |
4.5 |
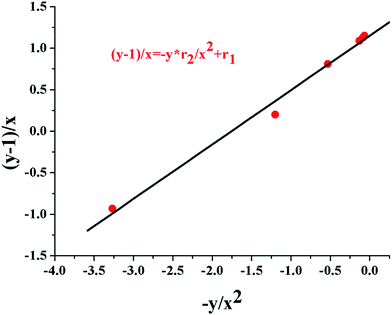 |
| Fig. 2 The dependence of (y − 1)/x and −y/x2 for the determination of monomer reactivity ratios during the anionic copolymerization of EO and EEGE, where x is the feed molar ratio of EO to EEGE, y is the molar ratio of EO to EEGE in the resulting copolymer. r1 and r2 are reactivity ratios of EO and EEGE, respectively. | |
In order to fabricate CdSe QD colloidal nanocrystal clusters capped with PEO as hydrophilic ligands using water-soluble multi-arm star-shaped brush-like block copolymer (PEO-g-PAA)-b-PEO as polymeric nanoreactors, a second block hydrophilic homopolymer PEO chain grown on the first block poly(EO-co-EEGE) arm end is necessary. Sequential anionic polymerization strategy was used to polymerize monomer EO, the end hydroxyl groups of each arm of multi-arm star-shaped copolymers poly(EO-co-EEGE) and DPMNa was exploited as the macroinitiator system. Details of the resulting multi-arm star-shaped block copolymers poly(EO-co-EEGE)-b-PEO samples are summarized in Table 3. After PEO chains had been grown on the end of poly(EO-co-EEGE) arm, obviously, the molecular weight of each arm increased (Fig. S2†). In addition, with the second block PEO capping the first block poly(EO-co-EEGE), the obvious increase of the EO unit molar ratio in multi-arm star-shaped block copolymer poly(EO-co-EEGE)-b-PEO demonstrates the successful growth of the second block PEO (Fig. 3).
Table 3 Summary of the details of the multi-arm star-shaped block copolymers poly(EO-co-EEGE)-b-PEO
Sample |
Time (h) |
Conversiona (%) |
RTb |
Mn,GPCc (kg mol−1) |
PDId |
Mn,arme (kg mol−1) |
Obtained by a gravimetric method. The unit molar ratio of EEGE to EO in multi-arm star-shaped block copolymers poly(EO-co-EEGE) measured by 1H NMR data. Number-average molecular weight determined by GPC. The polydispersity (PDI) determined by GPC. Mn of each PEO block arm calculated from the monomer conversion and the concentration of initiators. |
A-1a |
48 |
33.6 |
1/9.5 |
154 |
1.13 |
5.8 |
A-2a |
48 |
35.9 |
1/8.4 |
235 |
1.11 |
5.2 |
B-1a |
48 |
37.2 |
1/19.5 |
132 |
1.16 |
5.9 |
B-2a |
48 |
36.7 |
1/16.3 |
206 |
1.10 |
6.2 |
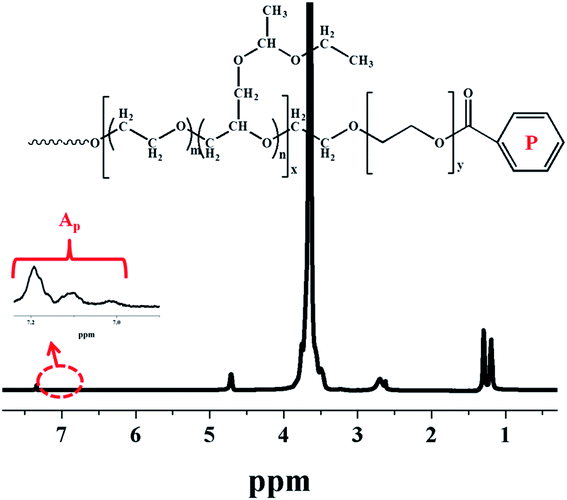 |
| Fig. 3 1H-NMR spectrum of multi-arm star-shaped block copolymers poly(EO-co-EEGE)-b-PEO after the end-capping reaction by benzenecarbonyl chloride (A-1a sample as shown in Table 3, solvent: CDCl3). | |
In order to obtain multi-arm star-shaped brush-like copolymers (PEO-g-PAA)-b-PEO with well-defined structures, the star-shaped brush-like PEO-g-PAA as core and PEO as shell, the end hydroxyl groups of multi-arm star-shaped block copolymers poly(EO-co-EEGE)-b-PEO must be deactivated by an end-capping reaction. The end hydroxyl groups of multi-arm star-shaped block copolymers poly(EO-co-EEGE)-b-PEO were end-capped by the reaction between hydroxyl groups and benzenecarbonyl chloride as end-capping reagent. Fig. 3 shows the 1H-NMR spectrum after the end-capping reaction, the appearance of a chemical shift at δ = 6.89–7.24 ppm is assigned to the protons of phenyl groups as the end group of arm chains. In addition, based on the 1H-NMR spectrum after the end-capping reaction, the initiating efficiency of hydroxyl groups of α-CD at the first anionic polymerization can be evaluated by the following eqn (4):
|
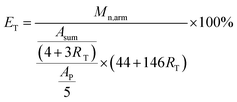 | (4) |
where
ET is the initiating efficiency of hydroxyl groups of α-CD at the first anionic polymerization;
Asum represents the integral area sum of the protons (b + e + f + g + h + i);
Ap represents the integral area of the protons of phenyl group as end-capping group;
RT is the molar ratio of EEGE units to EO units in copolymers; 146 and 44 are the molar masses of EEGE and EO;
Mn,arm is the molecular weight of each block copolymer poly(EO-
co-EEGE)-
b-PEO arm calculated from the monomer conversion and the concentration of initiators. The
ET value is nearly 100%, and this suggests that hydroxyl groups are almost completely grown into copolymer arms.
In Scheme 1, the procedure for the synthesis of multi-arm star-shaped brush-like copolymers (PEO-g-PtBA)-b-PEO is described. The ATRP of tBA monomer was carried out in methyl ethyl ketone at 60 °C, using multi-arm star-shaped block copolymers poly(EO-co-BiBGE)-b-PEO as the macroinitiator (Fig. S5 and S6†), and PMDETA–CuBr as the catalyst system. The resulting multi-arm star-shaped brush-like block copolymers consist of PEO as the main chain, PtBA as the graft chains and a second PEO block as the shell. Four multi-arm star-shaped brush-like block copolymer (PEO-g-PtBA)-b-PEO samples were prepared by using four corresponding star-shaped brush-like macroinitiators with different bromoisobutyryl group density, and all the results are shown in Table 4. Fig. 4 shows the 1H NMR spectrum of the resultant multi-arm star-shaped brush-like block copolymer (PEO-g-PtBA)-b-PEO sample A-1b in Table 4. The peaks at δ = 4.05–4.40 ppm were assigned to the methylene protons (He) linked to the ester and the end methine protons at the ω-end of the copolymer side chains. A characteristic strong peak at 1.45 ppm corresponding to the methyl protons in the t-butyl group of the tBA unit, and the chemical shift at δ = 3.46–3.75 ppm represents the protons of the PEO backbone. In addition, the molecular weight of the PtBA grafting side chains can be obtained by eqn (5):
|
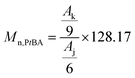 | (5) |
where
Mn,PtBA is the molecular weight of the P
tBA grafting side chains shown in
Table 4,
Ak and
Aj represent the integral area of the methyl protons in
t-butyl group of the grafted P
tBA chains and the integral area of methyl protons at the α-end of the P
tBA side chains, respectively. 128.17 is the molar mass of
tBA monomer.
Table 4 Summary of the details of multi-arm star-shaped brush-like copolymers (PEO-g-PtBA)-b-PEO
Sample |
Time (h) |
Conversion (%) |
Mn,GPCa (kg mol−1) |
PDIb |
Mn,PtBAc (kg mol−1) |
Number-average molecular weight determined by GPC. The polydispersity (PDI) determined by GPC. Mn of each PtBA graft chain calculated from 1H-NMR data. |
A-1b |
12 |
3.3 |
219 |
1.14 |
3.9 |
A-2b |
12 |
4.5 |
322 |
1.13 |
4.6 |
B-1b |
12 |
3.7 |
180 |
1.15 |
4.1 |
B-2b |
12 |
4.1 |
270 |
1.09 |
4.5 |
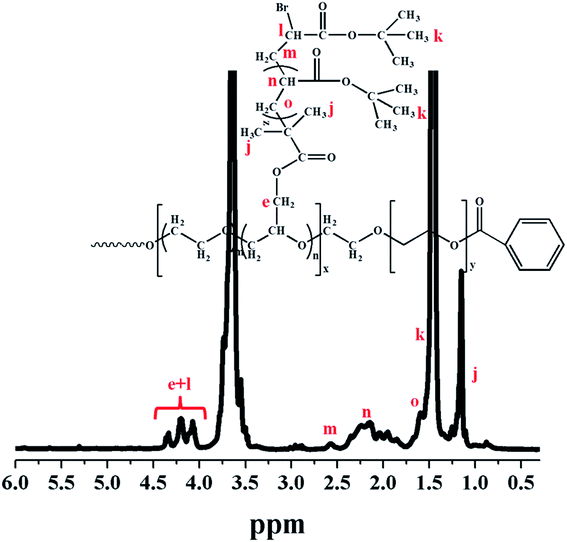 |
| Fig. 4 1H-NMR spectrum of multi-arm star-shaped brush-like block copolymer (PEO-g-PtBA)-b-PEO (A-1b sample in Table 4, solvent: CDCl3). | |
Finally, the water-soluble multi-arm star-shaped brush-like block copolymer (PEO-g-PAA)-b-PEO was prepared by the hydrolysis of the t-butyl groups of PtBA grafted side chains in trifluoroacetic acid. The characterization spectrum of the water-soluble multi-arm star-shaped brush-like block copolymer is shown in Fig. 5. The complete disappearance of the chemical shift at 1.45 ppm corresponding to the methyl protons of the t-butyl groups demonstrated the successful hydrolysis of PtBA grafting chains. In addition, FT-IR analysis was used to confirm the successful hydrolysis of the t-butyl groups. As shown in Fig. 6, the broad absorbance characteristic of a carboxylic acid group appeared at 2800–3600 cm−1 and the carbonyl stretch had shifted from 1726 to 1700 cm−1.
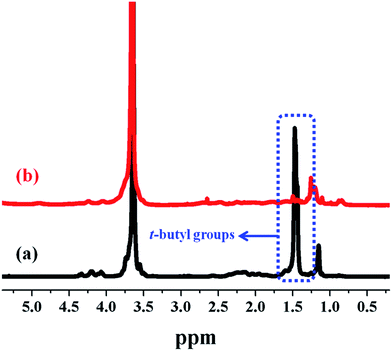 |
| Fig. 5 1H NMR spectra of multi-arm star-shaped brush-like block copolymers (a) (PEO-g-PtBA)-b-PEO (sample A-1b in Table 4) in CDCl3 and (b) the resulting (PEO-g-PAA)-b-PEO in CD3OD. | |
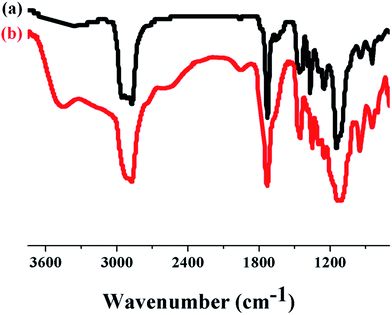 |
| Fig. 6 FT-IR spectra of multi-arm star-shaped brush-like block copolymers (a) (PEO-g-PtBA)-b-PEO (sample A-1b in Table S4†) and (b) the corresponding (PEO-g-PAA)-b-PEO. | |
Fabrication of hybrid inorganic–organic core–shell CdSe QD colloidal nanocrystal clusters capped with hydrophilic PEO as the shell
Quantum dots (QDs), as light-emitting particles on the nanometer scale, have emerged as a new class of materials for applications in solar cells,61 LEDs,62 tunable lasers,63 biosensors,64 bioimaging systems.65 For QDs such as cadmium selenide (CdSe), variation of particle size provides continuous and predictable changes in optical absorption and fluorescence due to their quantum-confined nature. QDs synthesized via a conventional organometallic high temperature growth procedure are coated with hydrophobic ligands (e.g., trioctylphosphine oxide (TOPO)). However, on many occasions, it is desirable to prepare water soluble QDs for bioimaging, biosensor, QD-based devices, etc.64–66 In addition, as one of the most important manipulations for applications, the assembly of these nanocrystals into secondary nanostructures is highly desired in order to combine the properties of individual nanocrystals and their secondary structures due to interactions of the subunits.67–69
Owing to their ability to fabricate functional inorganic nanomaterials with well-defined structures, multimolecular micelles formed from the aggregation of linear amphiphilic block copolymers were used as an attractive preparation strategy to fabricate functional colloidal nanocrystals via combination with sol–gel processes.43 Multimolecular micelles based on linear amphiphilic block copolymers, when their concentration is larger than the critical micelle concentration, are dynamically stable, and their morphology and structures in a given system depend heavily on temperature and solvent properties.43,70 With the changing of concentration, solvent properties, temperature, pH values, etc., the shapes and structures of multimolecular micelles may vary.45 Compared with multimolecular templates, polymeric nanoreactors with uniform and structurally stable spherical structures from multi-arm star-shaped brush-like block copolymer (PEO-g-PAA)-b-PEO are static rather than dynamic.21,71 After the water-soluble multi-arm star-shaped brush-like block copolymer (PEO-g-PAA)-b-PEO was completely dissolved in the polar solvent DMF, a good solvent for both PAA and PEO segments, polymeric nanoreactors were formed. AFM characterizations were performed to measure the characteristics of the polymeric nanoreactors. Clearly, the water-soluble multi-arm star-shaped brush-like block copolymer (PEO-g-PAA)-b-PEO formed spherical architectures with an average diameter of ∼44 nm (Fig. 7(a) and (b)). In order to further investigate the architectures of the star-shaped brush-like block copolymer (PEO-g-PAA)-b-PEO, TEM characterization was carried out. The dark spherical structures in the TEM micrographs correspond to the hydrophilic PAA brush core as uranyl acetate preferentially stained the PAA segments of macromolecular architectures (Fig. 7(c) and (d)). The average diameter of the cores was 34 ± 3.8 nm, and the size was smaller than that of AFM results owing to the second PEO as a shell.
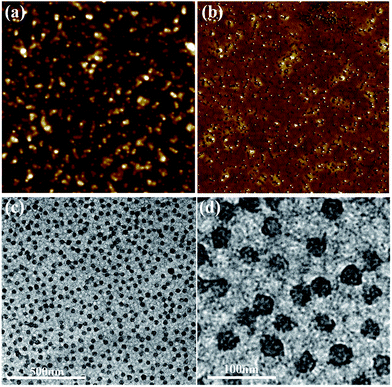 |
| Fig. 7 Morphology of the water-soluble multi-arm star-shaped brush-like block copolymer (PEO-g-PAA)-b-PEO macromolecular structures. (a and b) AFM height and phase images of the water-soluble multi-arm star-shaped brush-like block copolymer (PEO-g-PAA)-b-PEO (sample A-1b as precursor in Table 4), respectively. Image size = 5.0 × 5.0 μm2, Z range = 68 nm for (a) and 27° for (b). (c and d) TEM images of the water-soluble multi-arm star-shaped brush-like block copolymer (PEO-g-PAA)-b-PEO (sample A-1b as precursor in Table 4) with different scale bars. The samples were treated with uranyl acetate before imaging to selectively stain the hydrophilic core PAA segments. | |
Subsequently, the water-soluble multi-arm star-shaped brush-like block copolymer (PEO-g-PAA)-b-PEO was utilized as a multicompartment polymeric nanoreactor to structure-direct the precursors of CdSe QDs into highly water-dispersed CdSe QD colloidal nanocrystal clusters. Cd(acac)2 and NaHSe were used as precursor system, DMF as polar solvent. Precursors were loaded into the PAA compartment of templates owing to the strong coordination of carboxyl groups with the metal moiety of the precursors. After that, hybrid inorganic–organic core–shell CdSe QD colloidal nanocrystal clusters were fabricated by the reaction of NaHSe and Cd(acac)2, within which spherical CdSe colloidal nanocrystal clusters were intimately and permanently capped with hydrophilic PEO chains as the shell on the surface to render the resulting clusters with high water dispersible property. The morphology of spherical CdSe nanocrystal clusters with an average diameter of 29 ± 3.3 nm was characterized by TEM, as demonstrated in the representative TEM images at different magnification in Fig. 8. According to the close observation of these images, these uniform spherical colloids comprise small primary CdSe nanocrystals. The subunits of clusters can be investigated more clearly, and crystal lattices were clearly shown in Fig. 8(D), high resolution TEM (HR-TEM) image. Clearly, the spherical clusters consist of small primary nanocrystals as subunits with a diameter of 3.2 ± 0.3 nm. X-ray diffraction (XRD) measurements also confirm the subunit crystal structure of CdSe colloidal nanocrystal clusters (Fig. S10†). In addition, energy dispersive spectroscopy (EDS) microanalysis was also used to confirm the composition of CdSe QDs, as shown in Fig. S11.†
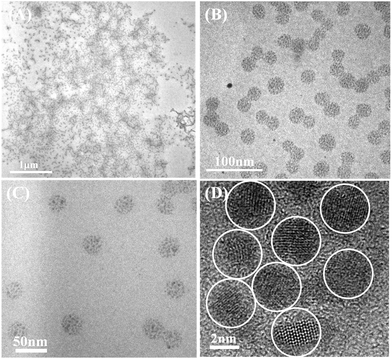 |
| Fig. 8 Representative TEM and HR-TEM images of CdSe colloidal nanocrystal clusters with different scale bars, the water-soluble multi-arm star-shaped brush-like block copolymer (PEO-g-PAA)-b-PEO (sample A-1b as precursor in Table 4) used as template. (a–c) Representative TEM images. (d) Typical HR-TEM image. | |
The UV-vis absorption and photoluminescence (PL) spectra of CdSe colloidal nanocrystal clusters coated with hydrophilic PEO polymer chains as shell dispersed in DI water are shown in Fig. 9. The absorption peak was at around 546 nm, and the emission peak was located at about 553 nm. Based on the absorption peak, the diameter of the CdSe crystals as subunits in colloidal clusters can be calculated according to previous reports in the literature,72,73 D ≈ 2.9 nm, which is correlated well with the TEM sizes of CdSe nanocrystals (see Fig. 8). Owing to surface-tethered hydrophilic PEO polymer chains as shell, the resulting hybrid inorganic–organic core–shell CdSe colloidal nanocrystal clusters from the star-shaped brush-like block copolymer polymeric nanoreactor are highly water-dispersed. Digital images of CdSe colloidal nanocrystal clusters dispersed into DI water are shown as insets in Fig. 9. Notably, the aqueous solution of CdSe colloidal nanocrystal clusters emitted green fluorescence under UV illumination.
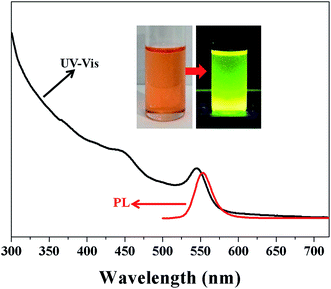 |
| Fig. 9 UV-vis absorption and photoluminescence (PL) spectra of CdSe colloidal nanocrystal clusters, the water-soluble multi-arm star-shaped brush-like block copolymer (PEO-g-PAA)-b-PEO (sample A-1b as precursor in Table 4) used as polymeric nanoreactor. Digital images of the aqueous solution of CdSe colloidal nanocrystal clusters before and after UV illumination are shown as insets. | |
Conclusion
In summary, a series of novel water-soluble multi-arm star-shaped brush-like block copolymers, composed of hydrophilic PEO as the main chain, hydrophilic polyelectrolyte PAA as functional graft chains, and a second PEO block as the shell (i.e., multi-arm star-shaped brush-like block copolymer (PEO-g-PAA)-b-PEO) with different molecular weights and grafting density, were rationally designed and synthesized by a combination of anionic copolymerization and ATRP. The anionic ring-opening copolymerization of EO and EEGE was conducted first by using α-CD with 18 hydroxyl groups and DPMNa as co-initiator system. The monomer reactivity ratios for EO and EEGE were also determined: r1(EO) = 1.18 ± 0.03 and r2(EEGE) = 0.79 ± 0.01, respectively. Then the resulting multi-arm star-shaped copolymers of poly(EO-co-EEGE) with hydroxyls as end functional groups were utilized as star-shaped macroinitiators to sequentially initiate the anionic ring-opening polymerization of monomer EO for the second block hydrophilic homopolymer PEO chain growing on the first block poly(EO-co-EEGE) arm end, and then ethoxyethyl groups of star-shaped block copolymers of poly(EO-co-EEGE)-b-PEO obtained were removed by hydrolysis. The formed multi-arm star-shaped block copolymers of EO and glycidol (Gly) with multi-pendant hydroxymethyls (star-shaped block copolymer poly(EO-co-Gly)-b-PEO) was then esterified by the reaction of pending hydroxyl groups of glycidol units of copolymers with 2-bromoisobutyryl bromide, and the resulting star-shaped brush-like macroinitiators were used to initiate the grafting copolymerization of tBA monomer by ATRP technique. Finally, the tert-butyl ester groups of PtBA grafting side chains were selectively hydrolyzed in trifluoroacetic acid to obtain the water-soluble multi-arm star-shaped brush-like block copolymer (PEO-g-PAA)-b-PEO. The water-soluble star-shaped brush-like (PEO-g-PAA)-b-PEO, consisted of functional PAA domain as the core and hydrophilic PEO domain as the shell, were exploited as polymeric nanoreactors to structure-direct in situ fabrication of CdSe QD colloidal nanocrystal clusters composed of primary CdSe nanocrystals as subunits. Spherical CdSe colloidal nanocrystal clusters were intimately and permanently capped with hydrophilic PEO chains on the surface to render the resulting clusters with high water dispersible property. The present synthetic approach of functional copolymers with complicated structures is simple yet robust and may be extended to create a myriad of copolymers with different conformations and functions for a fundamental study of the relationship between the architectures and their properties in solution and the solid state. Furthermore, going beyond the rational fabrication of CdSe colloidal nanocrystal clusters, we envision that, by judicious choice of different inorganic precursors, a family of intriguing functional colloidal nanocrystal clusters can be fabricated via the polymeric nanoreactor strategy.
Acknowledgements
We gratefully acknowledge funding support from National High Technology Research and Development Program of China (863 Program, No. 2011AA02A204-03) and 1000 Young Talent (to Xinchang Pang).
References
- H. Colfen, Macromol. Rapid Commun., 2001, 22, 219–252 CrossRef CAS.
- E. S. Gil and S. A. Hudson, Prog. Polym. Sci., 2004, 29, 1173–1222 CrossRef CAS.
- M. Oishi, Y. Nagasaki, K. Itaka, N. Nishiyama and K. Kataoka, J. Am. Chem. Soc., 2005, 127, 1624–1625 CrossRef CAS PubMed.
- B. L. Rivas, E. D. Pereira and I. Moreno-Villoslada, Prog. Polym. Sci., 2003, 28, 173–208 CrossRef CAS.
- J. Rodriguez-Hernandez and S. Lecommandoux, J. Am. Chem. Soc., 2005, 127, 2026–2027 CrossRef CAS PubMed.
- H. Mori and A. H. E. Müller, Prog. Polym. Sci., 2003, 28, 1403–1439 CrossRef CAS.
- M. Zhang, M. Drechsler and A. H. E. Müller, Chem. Mater., 2004, 16, 537–543 CrossRef CAS.
- X. Pang, L. Zhao, W. Han, X. Xin and Z. Lin, Nat. Nanotechnol., 2013, 8, 426–431 CrossRef CAS PubMed.
- A. A. Zezin, V. I. Feldman, S. S. Abramchuk, G. V. Danelyan, V. V. Dyo, F. A. Plamper, A. H. E. Muller and D. V. Pergushov, Phys. Chem. Chem. Phys., 2015, 17, 11490–11498 RSC.
- M. F. Zhang, C. Estournes, W. Bietsch and A. H. E. Muller, Adv. Funct. Mater., 2004, 14, 871–882 CrossRef CAS.
- W. Tjandra, J. Yao, P. Ravi, K. C. Tam and A. Alamsjah, Chem. Mater., 2005, 17, 4865–4872 CrossRef CAS.
- A. Harada and K. Kataoka, J. Am. Chem. Soc., 1999, 121, 9241–9242 CrossRef CAS.
- D. Wakebayashi, N. Nishiyama, Y. Yamasaki, K. Itaka, N. Kanayama, A. Harada, Y. Nagasaki and K. Kataoka, J. Controlled Release, 2004, 95, 653–664 CrossRef CAS PubMed.
- A. Guo, G. Liu and J. Tao, Macromolecules, 1996, 29, 2487–2493 CrossRef CAS.
- H. Gao and K. Matyjaszewski, Macromolecules, 2006, 39, 4960–4965 CrossRef CAS.
- N. Hadjichristidis, M. Pitsikalis, S. Pispas and H. Iatrou, Chem. Rev., 2001, 101, 3747–3792 CrossRef CAS PubMed.
- A. A. Steinschulte, B. Schulte, N. Drude, M. Erberich, C. Herbert, J. Okuda, M. Moller and F. A. Plamper, Polym. Chem., 2013, 4, 3885–3895 RSC.
- T. H. Mourey, S. R. Turner, M. Rubinstein, J. M. J. Frechet, C. J. Hawker and K. L. Wooley, Macromolecules, 1992, 25, 2401–2406 CrossRef CAS.
- C. J. Hawker, P. J. Farrington, M. E. Mackay, K. L. Wooley and J. M. J. Frechet, J. Am. Chem. Soc., 1995, 117, 4409–4410 CrossRef CAS.
- J. F. G. A. Jansen, E. M. M. de Brabander-van den Berg and E. W. Meijer, Science, 1994, 266, 1226–1229 CAS.
- X. Pang, L. Zhao, M. Akinc, J. K. Kim and Z. Lin, Macromolecules, 2011, 44, 3746–3752 CrossRef CAS.
- K. Hatada, T. Nishiura, T. Kitayama and K. Ute, Macromol. Symp., 1997, 118, 135–141 CrossRef CAS.
- D. T. Wu, Synth. Met., 2002, 126, 289–293 CrossRef CAS.
- K. Char, C. W. Frank and A. P. Gast, Langmuir, 1989, 5, 1335–1340 CrossRef CAS.
- K. Char, C. W. Frank and A. P. Gast, Langmuir, 1989, 5, 1096–1105 CrossRef CAS.
- K. Char, C. W. Frank and A. P. Gast, Macromolecules, 1989, 22, 3177–3180 CrossRef CAS.
- H. I. Lee, J. Pietrasik, S. S. Sheiko and K. Matyjaszewski, Prog. Polym. Sci., 2010, 35, 24–44 CrossRef CAS.
- L. H. He, J. Huang, Y. M. Chen, X. J. Xu and L. P. Liu, Macromolecules, 2005, 38, 3845–3851 CrossRef CAS.
- D. Neugebauer, Y. Zhang, T. Pakula, S. S. Sheiko and K. Matyjaszewski, Macromolecules, 2003, 36, 6746–6755 CrossRef CAS.
- J. Rzayev, Macromolecules, 2009, 42, 2135–2141 CrossRef CAS.
- S.-H. Lee, M. Ouchi and M. Sawamoto, Macromolecules, 2012, 45, 3702–3710 CrossRef CAS.
- K. Nakatani, T. Terashima and M. Sawamoto, J. Am. Chem. Soc., 2009, 131, 13600–13601 CrossRef CAS PubMed.
- M. Ouchi, T. Terashima and M. Sawamoto, ChemInform, 2010, 41, 4963–5050 Search PubMed.
- K. Huang and J. Rzayev, J. Am. Chem. Soc., 2009, 131, 6880–6885 CrossRef CAS PubMed.
- X. Pang, L. Zhao, C. Feng, R. Wu, H. Ma and Z. Lin, Polym. Chem., 2013, 4, 2025–2032 RSC.
- X. Pang, L. Zhao, C. Feng and Z. Lin, Macromolecules, 2011, 44, 7176–7183 CrossRef CAS.
- P. Li, Z. Li and J. Huang, Macromolecules, 2007, 40, 491–498 CrossRef CAS.
- D. Peng, X. Zhang and X. Huang, Macromolecules, 2006, 39, 4945–4947 CrossRef CAS.
- R. T. A. Mayadunne, J. Jeffery, G. Moad and E. Rizzardo, Macromolecules, 2003, 36, 1505–1513 CrossRef CAS.
- J. Teng and E. R. Zubarev, J. Am. Chem. Soc., 2003, 125, 11840–11841 CrossRef CAS PubMed.
- W. J. Cho, Y. Kim and J. K. Kim, ACS Nano, 2012, 6, 249–255 CrossRef CAS PubMed.
- Y. Liu, L. He, C. Xu and M. Han, Chem. Commun., 2009, 6566–6568 RSC.
- W. L. Leong, P. S. Lee, A. Lohani, Y. M. Lam, T. Chen, S. Zhang, A. Dodabalapur and S. G. Mhaisalkar, Adv. Mater., 2008, 20, 2325–2331 CrossRef CAS.
- G. Riess, Prog. Polym. Sci., 2003, 28, 1107–1170 CrossRef CAS.
- S. B. Darling, Prog. Polym. Sci., 2007, 32, 1152–1204 CrossRef CAS.
- M. Liu, K. Kono and J. M. J. Fréchet, J. Controlled Release, 2000, 65, 121–131 CrossRef CAS PubMed.
- A. W. Bosman, H. M. Janssen and E. W. Meijer, Chem. Rev., 1999, 99, 1665–1688 CrossRef CAS PubMed.
- Z. Li, P. Li and J. Huang, J. Polym. Sci., Part A: Polym. Chem., 2006, 44, 4361–4371 CrossRef CAS.
- H. Schuch, J. Klingler, P. Rossmanith, T. Frechen, M. Gerst, J. Feldthusen and A. H. E. Müller, Macromolecules, 2000, 33, 1734–1740 CrossRef CAS.
- H. Cui, Z. Chen, S. Zhong, K. L. Wooley and D. J. Pochan, Science, 2007, 317, 647–650 CrossRef CAS PubMed.
- J. Wu, Y. S. Thio and F. S. Bates, J. Polym. Sci., Part B: Polym. Phys., 2005, 43, 1950–1965 CrossRef CAS.
- J. M. Dean, P. M. Lipic, R. B. Grubbs, R. F. Cook and F. S. Bates, J. Polym. Sci., Part B: Polym. Phys., 2001, 39, 2996–3010 CrossRef CAS.
- L. C. Sawyer, D. T. Grubb and G. F. Meyers, Polymer Microscopy, Springer Science & Business Media, 2008 Search PubMed.
- I. Erol, C. Soykan and M. A. Erol, J. Polym. Sci., Part A: Polym. Chem., 2002, 40, 1756–1763 CrossRef CAS.
- Y. Zhang, G. Wang and J. Huang, J. Polym. Sci., Part A: Polym. Chem., 2010, 48, 5974–5981 CrossRef CAS.
- D. Pan, Q. Wang, S. Jiang, X. Ji and L. An, J. Phys. Chem. C, 2007, 111, 5661–5666 CAS.
- N. Ziqubu, K. Ramasamy, P. V. S. R. Rajasekhar, N. Revaprasadu and P. O’Brien, Chem. Mater., 2010, 22, 3817–3819 CrossRef CAS.
- Z. Liu, M. Frasconi, J. Lei, Z. J. Brown, Z. Zhu, D. Cao, J. Iehl, G. Liu, A. C. Fahrenbach, Y. Y. Botros, O. K. Farha, J. T. Hupp, C. A. Mirkin and J. Fraser Stoddart, Nat. Commun., 2013, 4, 1855 CrossRef PubMed.
- I. Tomatsu, A. Hashidzume and A. Harada, J. Am. Chem. Soc., 2006, 128, 2226–2227 CrossRef CAS PubMed.
- X.-S. Feng, D. Taton, E. L. Chaikof and Y. Gnanou, J. Am. Chem. Soc., 2005, 127, 10956–10966 CrossRef CAS PubMed.
- R. P. Raffaelle, S. L. Castro, A. F. Hepp and S. G. Bailey, Progress in Photovoltaics: Research and Applications, 2002, 10, 433–439 CrossRef CAS.
- N.-M. Park, T.-S. Kim and S.-J. Park, Appl. Phys. Lett., 2001, 78, 2575–2577 CrossRef CAS.
- K. T. Shimizu, R. G. Neuhauser, C. A. Leatherdale, S. A. Empedocles, W. K. Woo and M. G. Bawendi, Phys. Rev. B: Condens. Matter Mater. Phys., 2001, 63, 205316 CrossRef.
- W. W. Yu, E. Chang, R. Drezek and V. L. Colvin, Biochem. Biophys. Res. Commun., 2006, 348, 781–786 CrossRef CAS PubMed.
- J. Li and J.-J. Zhu, Analyst, 2013, 138, 2506–2515 RSC.
- B. S. Mashford, M. Stevenson, Z. Popovic, C. Hamilton, Z. Zhou, C. Breen, J. Steckel, V. Bulovic, M. Bawendi, S. Coe-Sullivan and P. T. Kazlas, Nat. Photonics, 2013, 7, 407–412 CrossRef CAS.
- J. Ge, Y. Hu, M. Biasini, W. P. Beyermann and Y. Yin, Angew. Chem., Int. Ed., 2007, 46, 4342–4345 CrossRef CAS PubMed.
- J. Ge, Y. Hu and Y. Yin, Angew. Chem., 2007, 119, 7572–7575 CrossRef.
- A. Sánchez-Iglesias, M. Grzelczak, T. Altantzis, B. Goris, J. Pérez-Juste, S. Bals, G. van Tendeloo, S. H. Donaldson, B. F. Chmelka, J. N. Israelachvili and L. M. Liz-Marzán, ACS Nano, 2012, 6, 11059–11065 CrossRef PubMed.
- J.-F. Gohy, in Block Copolymers II, ed. V. Abetz, Springer, Berlin Heidelberg, 2005, vol. 190, pp. 65–136 Search PubMed.
- G. R. Newkome, C. N. Moorefield, G. R. Baker, M. J. Saunders and S. H. Grossman, Angew. Chem., Int. Ed., 1991, 30, 1178–1180 CrossRef.
- J. Jasieniak, L. Smith, J. v. Embden, P. Mulvaney and M. Califano, J. Phys. Chem. C, 2009, 113, 19468–19474 CAS.
- S. Neeleshwar, C. L. Chen, C. B. Tsai, Y. Y. Chen, C. C. Chen, S. G. Shyu and M. S. Seehra, Phys. Rev. B: Condens. Matter Mater. Phys., 2005, 71, 201307 CrossRef.
Footnotes |
† Electronic supplementary information (ESI) available: GPC traces, 1H-NMR spectra, DLS characterization, TEM images of intermediates; XRD and EDS of CdSe colloidal nanocrystal clusters. See DOI: 10.1039/c5ra18130e |
‡ These authors contributed equally to this work. |
|
This journal is © The Royal Society of Chemistry 2015 |