DOI:
10.1039/C5RA17857F
(Paper)
RSC Adv., 2015,
5, 100362-100368
Synthesis and characterization of a CaFe2O4 catalyst for oleic acid esterification
Received
2nd September 2015
, Accepted 16th November 2015
First published on 17th November 2015
Abstract
Esterification of free fatty acid (oleic acid) with ethanol over a calcium ferrite catalyst was investigated in the present study. The calcium ferrite catalyst (CaFe2O4) was synthesized by the sol–gel method, which exhibited high catalytic activity for esterification of oleic acid. The morphology and size (500–1000 nm) of the synthesized catalyst were observed by scanning electron microscopy (SEM) and energy-dispersive X-ray spectroscopy (EDS) was used to ensure the absence of impurities. The orthorhombic structure of calcium ferrite was exposed by X-ray diffractometry (XRD). The effects of reaction variables such as catalyst loading, methanol to acid ratio, reaction time and temperature on the conversion of fatty acids were studied. The optimum conditions for the esterification process was a molar ratio of alcohol to oleic acid at 12
:
1 with 5 wt% of CaFe2O4 at 70 °C with a reaction time of 2 h. XRD patterns of the recycled catalyst evidenced that the catalyst structure was unchanged up to the 3rd cycle, which indicated the long life of the catalyst.
Introduction
Long chain methyl and ethyl esters derived from vegetable oil or animal fat, known as biodiesel, is considered as a potential alternative of diesel fuel. Theoretically, such oils and fats should not contain more than 1% free fatty acids (FFAs) since saponification of these FFAs reduces the yield of fatty acid alkyl esters (FAAEs) in alkaline transesterification reactions.1–3 Saponification is an undesirable reaction since it leads to extra cost for separation of biodiesel from glycerin and reduces the efficiency of the alkaline catalyst.2,4 Practically, higher levels of FFAs (up to 20%) were detected in waste oil, byproducts of refining of vegetable oils, some nonedible oils, animal fats and oils that are presently used as a starting material for biodiesel production.2,5,6 To efficiently utilize these low-cost feedstocks for biodiesel synthesis, a preliminary acid-catalyzed esterification pretreatment is necessary to reduce their FFA contents.7
Although liquid acids such as H2SO4, HF, H3PO4 and HCl are often used to lower the FFA level in those oils because of their high conversion and low cost, their usage are associated with effluent disposal problems, loss of catalyst and high equipment cost due to the corrosive nature of acids.8–10 The replacement of these hazardous and polluting corrosive homogeneous acid catalysts by heterogeneous reusable catalysts is one of the major demands of the present society. Already a number of heterogeneous catalysts have been developed,9,11,12 but most of them are not practicable due to either low conversion efficiency or higher oil to alcohol molar ratio or short life time and requirements of high reaction time and temperature. Srilatha et al.9 used heteropoly tungstate supported on niobia catalysts at the conditions 25% of catalyst loading, 14
:
1 of methanol to palmitic acid molar ratio, 4 h of reaction time at 65 °C and they achieved ∼90% conversion of palmitic acid. Besides, tungstated zirconia7 was used to esterify lauric acid in a well-stirred semi-batch reactor at 130 °C at atmospheric pressure and 85% conversion was achieved by 2 h. Another solid acid catalyst ferric-alginate was studied by ref. 13 with 0.16
:
1 ferric-alginate to lauric acid mass ratio, 16
:
1 methanol to lauric acid molar ratio, 3 h and they obtained 97.7% methyl laurate.
In the advanced level of solid acid catalyst study, attention has been focused on the porosity and particle size of the catalyst. Though Mekala et al.14 proposed a novel pore diffusion model where a major conversion of acidic acid occurs inside the catalyst pore, but the internal pore diffusion resistance was higher compared to bulk solution. Contrary, the large molecules have limited access to the internal pores of the catalyst pellets; hence the reaction occurs at the pore entrance, restricting further diffusion.15 On the other hand, catalyst with high surface area can provide highest fatty acid methyl ester (FAME) yield.16 Therefore, nano sized catalyst has become more attractive for the esterification of FFA. Wang et al.17 obtained ∼96% conversion of waste cooking oil using aluminumdodecatungstophosphate (AIPW) nanotube as catalyst at 55 °C, 1
:
34 oil/methanol ratio, 14 h of reaction time and 3 wt% of catalyst.
Most of the esterification of FFA studies used methanol1,9 as a short chain alcohol, now a days ethanol has become more rational because of some advantages over methanol, propanol, butanol and some other larger chain alcohols.18–20 Ethanol can be derived from agricultural products and is renewable and biologically less objectionable in the environment.18
In the present work, we have studied the structural and morphological properties of calcium ferrite catalysts and analyzed its catalytic activities in order to ascertain its application in the biodiesel field.
Experimental section
Catalyst preparation
The catalyst was prepared by a sol–gel method. A stoichiometric ratio of Ca(NO3)2·4H2O and Fe(NO3)3·9H2O were mixed in a three-neck flask with 30% aqueous NH3 solution, and the mixture was stirred at 200 rpm and at room temperature for 24 h and sol was obtained.21,22 The solution was then slowly heated to 80 °C and maintained at that temperature level until the water evaporated. The resulting brown dry gel-like slurry was calcined at 450 °C for 2 h followed by heat treatment at 1050 °C for 10 h using the furnace to obtained CaFe2O4 powder. Finally, CaFe2O4 powders were ground in the mortar. To recycle the catalyst, it was recovered by vacuum filtration unit using nylon membrane (pore size = 0.22 μm) followed by ethanol washing. The filter cake was dried in an oven at 100 °C, weighted and reused in the reaction system. Additionally, the method described by Oliveira et al.20 was followed to regenerate the catalyst, where after filtrating the catalyst was washed by n-hexane followed by drying in an oven at 100 °C. Finally the catalyst was calcined in a muffle furnace at 300 °C for 3 h.
Characterization of the catalyst
The acidity of the solid catalyst was determined by using Hammett indicator method. Acidic strength of the catalyst was tested using the modified method adopted from other studies,13,23 where 0.1 g of dried catalyst was added to a test tube and suspended in 3–5 mL of anhydrous methanol. Then, one drop of 0.1% Hammett indicator (methyl violet, thymol blue, and methyl orange) was added and left to equilibrate for 2 h. Any changes in color were noted.
Nitrogen adsorption isotherms of the fresh catalyst and the used catalyst (after 3rd cycle) were measured at −196 °C using an ASAP 2010 apparatus (Micromeritics). Firstly, all samples were outgassed at room temperature then at 200 °C to a pressure of <0.2 Pa for 5 h. The specific surface area was determined by the Brunauer Emmett Teller (BET) method. The pore size distributions were measured by Barrett–Joyner–Halenda (BJH) method and the total pore volume was calculated from the amount of N2 adsorbed up to P/P0 = 0.97.
X-Ray diffraction patterns of the prepared catalyst were collected using X-ray diffractometer (Rigaku MiniFlex II) operated at accelerating voltage of 30 kV and emission current of 15 mA with graphite-monochromatized Cu-Kα radiation and scanning speed 1° min−1. The scanning step size 0.02° was over a range of 2θ = 20–80°.
X-Ray photoelectron spectroscopy (XPS) analysis was carried out on a Quantum 2000 scanning ESCA microprobe system (Physical Electronics, Inc.). The microcrystalline structure and surface characteristics of the catalyst have been investigated by using Scanning Electron Microscope, SEM (Zeiss EVO MA 15). Sample powder was placed on an aluminum foil with double-sided carbon tape for energy dispersive X-ray spectroscopic (EDS) micro-analysis to determine the elemental compositions of the catalyst using JEOL JSM-7600, USA.
Esterification of oleic acid with ethanol
The esterification reaction of oleic acid was performed in a 500 mL three necked round bottom flask and equipped with a reflux condenser. Oleic acid (50 mL) was added into the flask followed by a mixture of ethanol (18.5–166.5 mL) and CaFe2O4 catalyst of 1, 3, 5, 7 and 8 wt% to the weight of oleic acid. The molar ratio of ethanol to oleic acid was 2
:
1, 4
:
1, 6
:
1, 8
:
1, 12
:
1 and 18
:
1. The reaction temperature was followed as 40, 50, 60 and 70 °C. The reaction was initiated by stirring, typically at 250 rpm, and stopped after reaction time of 10 h for product analysis. The product solution was centrifuged and amount of FFA was determined by titration method (ASTM D5555). In brief, 4–5 g of samples were dispersed in isopropanol (75 mL) and hexane (15 mL) followed by titration against 0.25 N NaOH solution.
Characterization of spent catalysts
To reuse the catalyst, after the first cycle, it was recovered by simple filtration, washed with ethanol, dried in an oven at 100 °C, weighted and inserted in the reaction system again. To improve the activity of used catalyst, they were regenerated by calcination process. The recycled catalyst was washed a sequence with n-hexane, dried in an oven at 100 °C and calcined at 300 °C for 3 h, before the catalyst was placed in the reactor, the procedure was adopted from Oliveira et al.20 XRD patterns of the reused catalyst were analyzed.
Results and discussion
Catalyst characterization
Catalyst acidity. The color change of indicators corresponding to their pH range which represent the acidity of the catalyst is presented in Table 1. The acid strength of the catalyst lies in the range between pH 1.6 and 2.8. This proves that the catalyst was strongly acidic and was most suitable for esterification reaction.
Table 1 Acidity of CaFe2O4, determined by Hammett indicator method
Indicators |
Color change |
pH range |
Observed color (fresh → after 3rd cycle) |
Methyl orange |
Yellow → red |
3.2–4.4 |
Red (<3.2) |
Thymol blue |
Yellow → red |
1.2–2.8 |
Red (<1.2) |
Methyl violet |
Blue → yellow |
0.0–1.6 |
Blue |
SEM and EDS analysis. SEM was used to exhibit the morphology and particle sizes of the samples. SEM images of calcined CaFe2O4 samples are shown in Fig. 1a. It can be observed that CaFe2O4 are nearly ball like spherical particles with the average diameter of 500–1000 nm. The particles are agglomerated form cluster structure and the sizes are relatively consisted with the broad size distribution, clearly represented in SEM analysis.
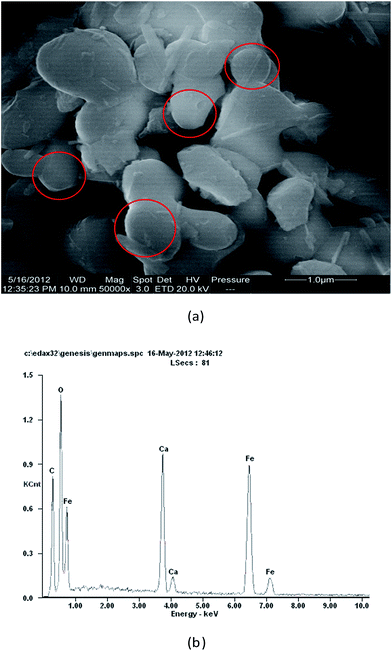 |
| Fig. 1 (a) SEM and (b) EDS of CaFe2O4 catalyst. | |
Crystallites of the CaFe2O4 samples could be consisted with the core–shell structure. The nonuniform crystalline core–shell structures of similar nanoparticles have recently been reported for MgFe2O4, LiNbO3, NiFe2O4.24–26 Meanwhile, the EDS spectrum, in Fig. 1b reveals that the CaFe2O4 spherical particles are mostly consisted with the higher purity of Ca, Fe, and O elements. In addition concentration and annealed temperature are significantly dominant to obtain the CaFe2O4. The signal of O may be from contributions, first it is from the oxygen absorbed on the surface of the sample and second is from the sample itself.
BET analysis
The specific surface area of the catalyst was determined by the modelling of N2 adsorption data with BET isotherm. The linearized BET plot is presented in Fig. 2. The obtained BET surface area, average pore diameter and specific pore volume for the fresh catalyst, were 2.48 m2 g−1, 96.11 Å, and 0.0078 cm3 g−1, respectively. The recycled catalyst after 3rd cycle showed the BET surface area, average pore diameter and specific pore volume as 1.97 m2 g−1, 91.18 Å, and 0.0063 cm3 g−1, respectively. Around 20% decrease in the surface area in the recycled catalyst might be due to the blockage of the pores during the reaction and regeneration process.
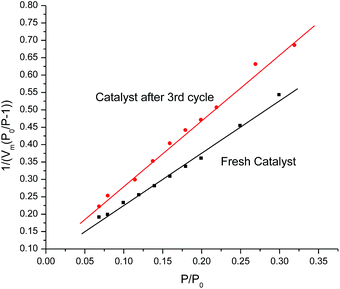 |
| Fig. 2 Linearized BET plot for fresh CaFe2O4 and after 3rd cycle. | |
XRD analysis. Crystalline structures of the CaFe2O4 particles could be clearly understood through the prominent use of XRD measurement. The sharp peaks from diffraction patterns show the crystalline nature of the samples. The XRD pattern of the synthesized calcium ferrite powder as shown in Fig. 3 can match well with the documented XRD data of CaFe2O4 (JCPDS card No. 32-0168), which indicated that well crystallized catalyst has been obtained. A typical XRD pattern of CaFe2O4 displays peaks at 2θ values of 28.68°, 32.4°, 47.68°, 56.12°, 58.96°, 69.36°, 76.56°, and 88.21° similar to those major XRD peaks of CaFe2O4 structure as reported in the literature.27
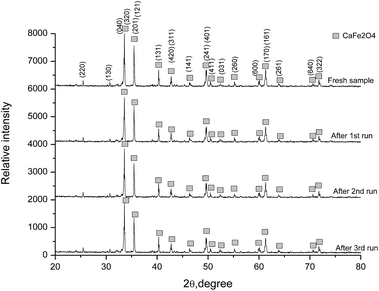 |
| Fig. 3 XRD patterns for the CaFe2O4 powder. | |
The diffraction peaks could be indexed to (220), (320), (040), (201), (121), (131), (311), (401), (170) and (322) planes. It is observed that a few major peaks of CaFe2O4—(040), (320), (201), (121), (241), (401) and (170) overlaps. Based on the analysis, the crystal structure of the mechanosynthesised product is found to be orthorhombic with the unit cell parameters a = 9.214(3) Å, b = 10.686(3) Å and c = 3.004(4) Å. It should be noted that these unit cell parameters are smaller to those reported for bulk CaFe2O4, (a = 9.230 Å, b = 10.705 Å, c = 3.024 Å).28
XPS analysis. Chemical composition of the CaFe2O4 particles was investigated by XPS analysis illustrated in the Fig. 4. It is perceived that oxygen on the sample surface exists at least in two specific forms with the following binding energies: 529.74 and 531.86 eV, as shown in Fig. 4a. The peak at 529.74 eV is mainly assigned to the oxygen in the sample lattice to the formation of CaFe2O4. On the other hand, the peak at 531.86 eV corresponds to oxygen in the sample surface adsorption of (–OH).
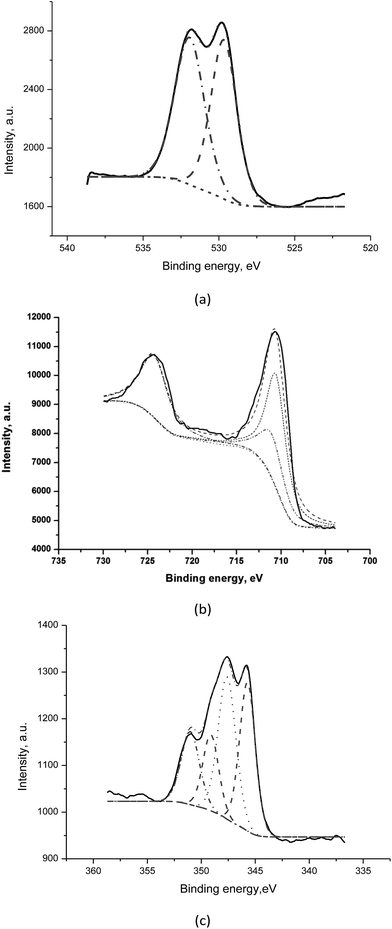 |
| Fig. 4 High-resolution XPS spectra of (a) O 1s, (b) Fe 2p, and (c) Ca 2p. | |
Fig. 4b shows the deconvoluted XPS spectrum of the two significant valence states of Fe in the Fe 2p region. The peaks at the binding energies of 710.55, 711.0 and 724.25 eV are attributed29–33 to Fe3+. This result contributes to the chemical existence of the formation of Fe based oxide material sample structure. The high resolution Ca 2p region spectra were deconvoluted into four peaks of the Fig. 4c, where, the peaks of 345.73 and 349.17 eV can be attributed to Ca 2p1/2 and consequently, 347.66 and 351.05 eV are represented as Ca 2p3/2. The peak located at 529.74 eV for O 1s in the sample reflects to the formation of CaFe2O4 structure. Additionally, the formation of CaFe2O4 materials is also substantiated by EDS and XRD analysis.
Catalytic activity
The initial catalytic activity of CaFe2O4 was slower than that of a homogeneous sulfuric acid catalyst up to 3 h. Fig. 5 illustrated that the rate of oleic acid conversion by both catalysts sulfuric acid and CaFe2O4 became almost same after 4 h. The maximum conversion (96%) was achieved by 4 h and there was no significant change with an extended time of reaction. Therefore, it would be very beneficial to replace the hazardous sulfuric acid catalyst by recyclable solid catalyst CaFe2O4. Moreover, it showed better conversion efficiency under favourable conditions compared to other solid catalysts (Table 2).
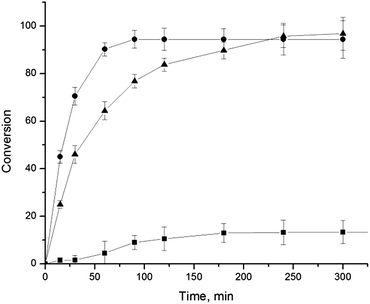 |
| Fig. 5 Catalytic activity of CaFe2O4 solid catalyst, sulphuric acid and noncatalytic (reaction temperature, 70 °C, mass amount of catalyst 5 wt%, ethanol/oleic acid mole ratio 12 : 1) ■ non-catalyzed ● H2SO4 catalyzed ▲ CaFe2O4 catalyzed. | |
Table 2 Esterification conditions of oleic acid by recently developed solid catalysts
Acid catalyst |
Temperature, °C |
Reaction time, h |
Alcohol : oleic acid |
Catalyst doses, wt% |
Oleic acid conversion, % |
Reference |
1-Butyl-3-methylimidazolium tetrachloroferrite ([BMIM][FeCl4]) |
65 |
3.6 |
22 : 1 |
— |
83.4 |
4 |
H3PW/ZrO2 |
100 |
4 |
6 : 1 |
20 |
88 |
20 |
Organophosphonic acid-functionalized silica SG–T–P |
112 |
10 |
8.8 : 1 |
14.5 |
77.02 |
36 |
Sulfonated cation exchange resin |
82 |
8 |
9 : 1 |
20 g |
93 |
37 |
Sulfonated carbons Starbons-300 |
80 |
3 |
10 : 1 |
1.33 |
60 |
38 |
CaFe2O4 |
70 |
4 |
12 : 1 |
5 |
96 |
Present study |
Effect of catalyst concentration
Fig. 6 shows the effect of catalyst doses and it was examined by dosing 1, 3, 5, 7 and 8 wt% of CaFe2O4 to oleic acid maintaining the reaction temperature 70 °C, ethanol/oleic acid mole ratio 12
:
1 and a reaction time 4 h. Using 1 wt% of catalyst, 70% conversion was achieved and the conversion ratio increased with the increasing catalyst amount, which could be attributed to the reason that more CaFe2O4 catalyst would provide more active reaction sites. It was clear that the amount of catalyst had a positive effect on the conversion ratio of oleic acid, and the conversion ratio (93%) became constant with catalyst amount above 5 wt%. Excess amount of catalyst may form emulsion which increased the viscosity and led to the formation of gels. The formation of emulsion will therefore block the reaction.34
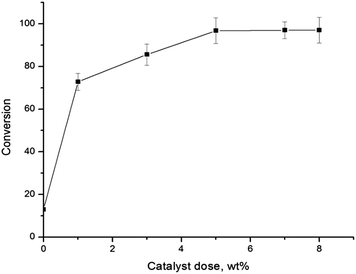 |
| Fig. 6 Conversion of the oleic acid with diverse catalyst doses (reaction temperature, 70 °C, ethanol/oleic acid mole ratio 12 : 1, reaction time 4 h). | |
Effect of ethanol/oleic acid molar ratio
Fig. 7 shows the effect of various ethanol/oleic acid molar ratios (6
:
1, 12
:
1, 18
:
1) on the ethyl ester conversion at 5 wt% of CaFe2O4 catalyst and 70 °C reaction temperature. As seen in Fig. 7, the ethyl ester conversion and the degree of the ethyl ester conversion depend largely upon ethanol/oleic acid molar ratio. The reaction was faster at the initial phase and reached a higher final conversion after 4 h and then it became constant. The conversion was increased from the ratio of 6
:
1 to 12
:
1 and it was unaffected for further increase to 18
:
1. The low yield at the feed ratio of 6
:
1 might be due to an insufficient quantity of ethanol for nucleophilic attack on the Brønsted acid sites of the catalyst.11 On the other hand, higher feed ratio did not increase the yield percentage and it might happen because of the production of water preferentially drives the hydrolysis of FAME into oleic acid.34
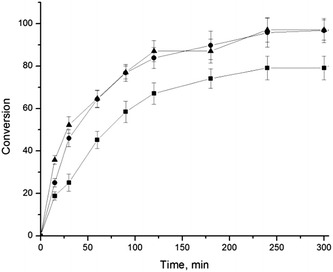 |
| Fig. 7 Conversion of the oleic acid with diverse ethanol/oleic acid molar ratio (reaction temperature 70 °C, mass amount of catalyst 5 wt%) ■ 6 : 1 ● 12 : 1 ▲ 18 : 1. | |
Effect of reaction temperature
The heterogeneous catalyzed esterification reaction is strongly influenced by the reaction temperature. The effect of the reaction temperature was studied from 40 to 70 °C with ethanol/oleic acid molar ratio 12
:
1 and 5 wt% of CaFe2O4 catalyst to oleic acid as shown in Fig. 8. The conversion rate of oleic acid was faster up to 1 h for all the batches, i.e., at 50 °C, 60 °C and 70 °C. Subsequently, the conversion reached to the steady state. However, the conversion rate was increased with the increase of temperature and the value was 95% for 70 °C. Fig. 8 also exhibits that the initial reaction rate was high, and then the reaction rate decreased. Fig. 8 displays that the initial reaction rate was high for all temperature ranges followed by a decrease in reaction rate leading to a plateau. In the initial reaction periods, the reaction mixture was free from water, which subsequently produced as the reaction progressed causing the backward reaction to occur.36
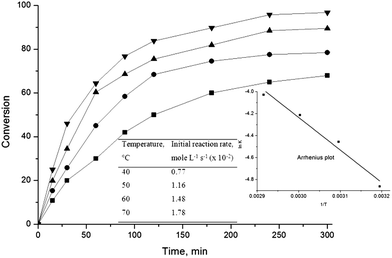 |
| Fig. 8 Conversion of the oleic acid with diverse temperature on oleic acid conversion (mass concentration of catalyst 5 wt%, ethanol/oleic acid mole ration 12 : 1) ■ 40 °C ● 50 °C ▲ 60 °C ▼ 70 °C, inset the figure: Arrhenius plot and table of initial reaction rates. | |
Initial reaction rate increased gradually with the increase of temperature (table inside the Fig. 8). The reaction rate constant (k) for the esterification reaction of the oleic acid was determined by fitting the experimental data to the first-order reaction kinetic model. The rate constants were calculated as 0.0077, 0.0116, 0.0148 and 0.0178 s−1 for 40, 50, 60 and 70 °C respectively. The activation energy was estimated as 24.60 kJ mol−1 from Arrhenius plot shown in the inset of Fig. 8. The smooth increase in rate constant with increase of temperature suggests that the mass transfer steps were not the rate limiting factor.
Catalyst reactivation and recyclability
To recycle the catalyst, it was recovered by simple filtration, washed with ethanol, dried in an oven at 100 °C, weighted and inserted in the reaction system again. Fig. 9 shows the activity of the calcinated and uncalcinated catalysts during multi-cycle uses. Without calcination, the conversion drops from 96 to 76% after the first cycle and at the fourth cycle it was about 65% (Fig. 9: column Y). To improve the activity of used catalyst, they were regenerated by calcination process. A better performance was obtained with a sequence of washing with n-hexane, drying in an oven at 100 °C and calcining at 300 °C for 3 h.20 In this case, the conversion was improved 13% after the first cycle and about 9% after the second cycle, though the improvement was insignificant after the third cycle (Fig. 9: column X). On the other hand, XRD patterns (Fig. 3) indicated that the synthesized particles were mainly composed of CaFe2O4. The fresh CaFe2O4 and used CaFe2O4 catalysts had well-crystallized structures with characteristic and symmetric reflections. Fresh CaFe2O4 and recycled CaFe2O4 (1st to 3rd cycles) catalysts had the same patterns, indicating that the CaFe2O4 catalyst was stable.
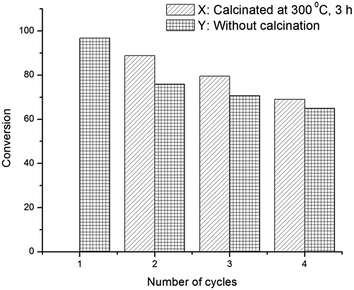 |
| Fig. 9 Conversion of the oleic acid with recycled catalyst (reaction time 4 h, mass concentration of catalyst 5 wt%, ethanol/oleic acid mole ratio 12 : 1, reaction temperature 70 °C). | |
Conclusions
The catalyst showed a remarkable success on the esterification of oleic acid with ethanol. The conversion of oleic acid reached equilibrium after an hour when all other reaction parameters were fixed. The maximum conversion of 96% was achieved under the conditions of ethanol to oil ratio 12
:
1, temperature 70 °C and catalyst dose 5 wt%. Preliminary study of recyclability for CaFe2O4 indicated that a treatment of calcining at 300 °C for 3 h recovered the conversion to about 88% and 80% after second and third cycles respectively. Further work is proceeding to increase its catalytic activity by impregnating precious metal to make the process industrially practicable.
Acknowledgements
Authors gratefully acknowledge the Faculty of Chemical and natural resources Engineering, University Malaysia Pahang for funding to carry out this work by a research grant (Project No.: RDU100395).
Notes and references
- M. Berrios, J. Siles, M. A. Martin and A. Martin, Fuel, 2007, 86, 2383–2388 CrossRef CAS.
- H. D. Hanh, N. T. Dong, K. Okitsu, R. Nishimura and Y. Maeda, Renewable Energy, 2009, 34, 780–783 CrossRef CAS.
- K.-S. Liu, J. Am. Oil Chem. Soc., 1994, 71, 1179–1187 CrossRef CAS.
- A. H. M. Fauzi, N. A. S. Amin and R. Mat, Appl. Energy, 2014, 114, 809–818 CrossRef.
- S. V. Ghadge and H. Raheman, Bioresour. Technol., 2006, 97, 379–384 CrossRef CAS PubMed.
- S. Zheng, M. Kates, M. A. Dubé and D. D. McLean, Biomass Bioenergy, 2006, 30, 267–272 CrossRef CAS.
- K. Suwannakarn, E. Lotero, K. Ngaosuwan and J. G. Goodwin Jr, Ind. Eng. Chem. Res., 2009, 48, 2810–2818 CrossRef CAS.
- F. Cao, Y. Chen, F. Zhai, J. Li, J. Wang, X. Wang, S. Wang and W. Zhu, Biotechnol. Bioeng., 2008, 101, 93–100 CrossRef CAS PubMed.
- K. Srilatha, N. Lingaiah, B. L. A. P. Devi, R. B. N. Prasad, S. Venkateswar and P. S. S. Prasad, Appl. Catal., A, 2009, 365, 28–33 CrossRef CAS.
- Y. Zhang, M. A. Dube, D. D. L. McLean and M. Kates, Bioresour. Technol., 2003, 89, 1–16 CrossRef CAS PubMed.
- K. U. Nandhini, B. Arabindoo, M. Palanichamy and V. Murugesan, J. Mol. Catal. A: Chem., 2006, 243, 183–193 CrossRef CAS.
- H. R. Ong, M. R. Khan, M. N. K. Chowdhury, A. Yousuf and C. K. Cheng, Fuel, 2014, 120, 195–201 CrossRef CAS.
- B. Peng-Lim, S. Ganesan, G. P. Maniam and M. Khairuddean, Energy, 2012, 46, 132–139 CrossRef CAS.
- M. Mekala, S. K. Thamida and V. R. Goli, Chem. Eng. Sci., 2013, 104, 565–573 CrossRef CAS.
- M. Absi-Halabi, A. Stanislaus, T. Al-Mughni, S. Khan and A. Qamra, Fuel, 1995, 74, 1211–1215 CrossRef CAS.
- S. Yan, M. Kim, S. O. Salley and K. Y. S. Ng, Appl. Catal., A, 2009, 360, 163–170 CrossRef CAS.
- J. Wang, Y. Chen, X. Wang and F. Cao, BioResources, 2009, 4, 1477–1486 CAS.
- A. Demirbas, Biomass Bioenergy, 2009, 33, 113–118 CrossRef CAS.
- G. F. Ghesti, J. L. de Macedo, I. S. Resck, J. A. Dias and S. C. L. Dias, Energy Fuels, 2007, 21, 2475–2480 CrossRef CAS.
- C. F. Oliveira, L. M. Dezaneti, F. A. C. Garcia, J. L. de Macedo, J. A. Dias, S. C. L. Dias and K. S. P. Alvim, Appl. Catal., A, 2010, 372, 153–161 CrossRef CAS.
- B. Xin, P. Wang, D. Ding, J. Liu, Z. Ren and H. Fu, Appl. Surf. Sci., 2008, 254, 2569–2574 CrossRef CAS.
- J. Liqiang, S. Xiaojun, C. Weimin, X. Zili, D. Yaoguo and F. Honggang, J. Phys. Chem. Solids, 2003, 64, 615–623 CrossRef.
- P.-L. Boey, S. Ganesan, G. P. Maniam, M. Khairuddean and J. Efendi, Energy Convers. Manage., 2013, 65, 392–396 CrossRef CAS.
- H. U. Khan, I. Sterianou, S. Miao, J. Pokorny and I. M. Reaney, J. Appl. Phys., 2012, 111, 024107 CrossRef.
- V. K. Mittal, S. Bera, T. Saravanan, S. Sumathi, R. Krishnan, S. Rangarajan, S. Velmurugan and S. V. Narasimhan, Thin Solid Films, 2009, 517, 1672–1676 CrossRef CAS.
- V. Šepelák, I. Bergmann, A. Feldhoff, P. Heitjans, F. Krumeich, D. Menzel, F. J. Litterst, S. J. Campbell and K. D. Becker, J. Phys. Chem. C, 2007, 111, 5026–5033 Search PubMed.
- Z. Liu, Z.-G. Zhao and M. Miyauchi, J. Phys. Chem. C, 2009, 113, 17132–17137 CAS.
- L. J. Berchmans, M. Myndyk, K. L. Da Silva, A. Feldhoff, J. Šubrt, P. Heitjans, K. D. Becker and V. Šepelák, J. Alloys Compd., 2010, 500, 68–73 CrossRef CAS.
- T. Yamashita and P. Hayes, Appl. Surf. Sci., 2008, 254, 2441–2449 CrossRef CAS.
- A. P. Grosvenor, B. A. Kobe, M. C. Biesinger and N. S. McIntyre, Surf. Interface Anal., 2004, 36, 1564–1574 CrossRef CAS.
- P. C. J. Graat and M. A. J. Somers, Appl. Surf. Sci., 1996, 100, 36–40 CrossRef.
- S. J. Roosendaal, B. van Asselen, J. W. Elsenaar, A. M. Vredenberg and F. H. P. M. Habraken, Surf. Sci., 1999, 442, 329–337 CrossRef CAS.
- P. Zhang, Y. Gong, H. Li, Z. Chen and Y. Wang, RSC Adv., 2013, 3, 5121–5126 RSC.
- L. Lin, D. Ying, S. Chaitep and S. Vittayapadung, Appl. Energy, 2009, 86, 681–688 CrossRef CAS.
- S. Gan, H. K. Ng, P. H. Chan and F. L. Leong, Fuel Process. Technol., 2012, 102, 67–72 CrossRef CAS.
- P. Yin, L. Chen, Z. Wang, R. Qu, X. Liu and S. Ren, Bioresour. Technol., 2012, 110, 258–263 CrossRef CAS PubMed.
- Y. Jiang, J. Lu, K. Sun, L. Ma and J. Ding, Energy Convers. Manage., 2013, 76, 980–985 CrossRef CAS.
- A. Aldana-Pérez, L. Lartundo-Rojas, R. Gómez and M. E. Niño-Gómez, Fuel, 2012, 100, 128–138 CrossRef.
|
This journal is © The Royal Society of Chemistry 2015 |