DOI:
10.1039/C5RA17557G
(Paper)
RSC Adv., 2015,
5, 107012-107019
A novel turn-on Schiff-base fluorescent sensor for aluminum(III) ions in living cells†
Received
30th August 2015
, Accepted 16th November 2015
First published on 27th November 2015
Abstract
(E)-4-Methoxy-N-((8-methylquionline-2yl)methylene)aniline (L) was successfully synthesized and characterized. The complexation behavior of L with different metal ions was studied using UV-Vis absorption spectroscopy and fluorescence spectroscopy. Results showed that the sensor (L) exhibited a 38-fold enhancement in fluorescence at 517 nm after adding 10 equiv. of Al3+ ions. Such fluorescent responses could be detected by the naked eye under a UV-lamp at 365 nm. The complex solution (L–Al3+) showed reversibility with EDTA and the complex was a 1
:
1 ratio according to Job’s plot and electrospray ionization mass spectroscopy (ESI-MS). A photoinduced electron transfer (PET) mechanism was considered to be operational for fluorescence enhancement. The detection limit of Al3+ was calculated to be 1.2 × 10−7 M, a satisfying level to detect Al3+ in the micromolar scale. Importantly, the chemosensor L could be used to detect and quantify Al3+ in living cells. Therefore, this sensor has the ability to be a practical system for monitoring Al3+ concentrations in biological systems.
1. Introduction
Aluminum is the third most abundant element in the earth’s crust. Due to acidic rain and human activities, Al3+ exists widely in the environment. Al3+ affects the activity of gastrointestinal enzymes and excess Al3+ is toxic in the central nervous system.1–3 The World Health Organization (WHO) recommends an average daily human intake of Al3+ of around 3–10 mg kg−1 and the tolerable weekly dietary intake as 7 mg kg−1 body weight. Excessive exposure of the human body to Al3+ leads to many diseases such as Alzheimer’s disease and Parkinson’s disease, bone softening, smoking related diseases and chronic renal failure.4–8 Up to now, many measurement technologies have been developed to sensitively and reliably detect Al3+ such as atomic absorption spectrometry, inductively coupled plasma-atomic emissions spectrometry and inductively coupled plasma-mass spectrometry.9 Compared to these approaches, fluorescent probes for detecting Al ions have attracted great interest from researchers due to their several outstanding advantages such as low cost, simplicity, high sensitivity and selectivity.
Since there is a close association between Al3+ and human health, the investigation of Al3+ detection has attracted increasing attention.10 In recent years, data showed that forty percent of children in China had an aluminum intake exceeding the limitation of the WHO recommended allowance.11 Therefore, the development of sensitive molecular signaling systems, especially for aluminum ions, has become increasingly important.12,13 To date, a good number of Schiff-base compounds have been reported very recently as a sensor for the detection of aluminum metal ions using fluorescence spectroscopy.14–23 J. Wang et al. reported commercially available compound, 2-hydroxy-1-naphthaldehyde, as a highly sensitive and selective fluorescent sensor for Al3+ for the first time in 2012.24 After that, a number of Schiff-bases have been synthesized over the past couple of years employing 2-hydroxy-1-naphthaldehyde as the aldehyde and have been applied as chemosensors for the detection of aluminum ions.25–29
Based on this knowledge, herein, a simple, high selectivity and high sensitivity Al3+ sensor has been designed and synthesized. We report the aluminum(III) sensing behavior of (E)-4-methoxy-N-((8-methylquionline-2yl)methylene)aniline (L) (Scheme 1). It has been prepared from the Schiff-base condensation between 8-hydroxyquinoline-2-carbaldehyde and anisidine. L has been characterized by different spectroscopic techniques.
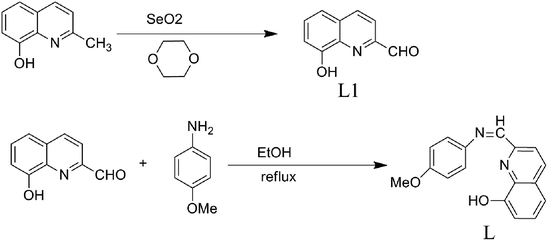 |
| Scheme 1 The synthetic routes of compound L. | |
2. Experimental
2.1. Materials and instrumentation
All reagents were purchased commercially of grade quality and used without any further purification. (E)-4-Methoxy-N-((8-methylquionline-2yl)methylene)aniline (L) was synthesized in the laboratory. Double-distilled water was used throughout the experiments. 1H NMR spectra were measured on a Bruker 500 MHz instrument using TMS as an internal standard. UV-Vis absorption spectra were determined on a U-3900 spectrophotometer. Fluorescence spectra were recorded with a Hitachi F-4600 spectrophotometer equipped with quartz cuvettes of 1 cm path length. MS spectra were measured on a Shimadzu GC-17A, QP-5000 GC/MS spectrometer. FT-IR spectra were recorded with a NICOLET 6700 spectrophotometer.
2.2. Synthesis and characterization
2.2.1. Synthesis of 8-hydroxyquinoline-2-carbaldehyde (L1). 2-methylquinoline-8-ol (3.2 g, 20 mmol) was dissolved in dioxane (90 mL), and SeO2 (2.8 g, 24.8 mmol) was added to this solution. The mixture was stirred at 80 °C for 24 h and then cooled to room temperature. The precipitate was filtered off. The solvents were evaporated to give the crude product, which was purified by flash chromatography on silica gel (5
:
1 petroleum ether/ethyl acetate as the eluent) to give a yellow solid L1 (3.28 g, 18.96 mmol, 95%). 1H NMR (500 MHz, CDCl3) δ 10.23 (s, 1H), 8.33 (d, J = 8.0 Hz, 1H), 8.15 (s, 1H), 8.07 (d, J = 8.3 Hz, 1H), 7.62 (d, J = 7.9 Hz, 1H), 7.44 (d, J = 7.4 Hz, 1H).
2.2.2. Synthesis of (E)-4-methoxy-N-((8-methylquionline-2yl)methylene)aniline (L). 8-Hydroxyquinoline-2-carbaldehyde (0.692 g, 4 mmol) was dissolved in ethanol (30 mL); then anisidine (0.492 g, 4 mmol) was added to the solution. The solution was refluxed for 4 h under stirring. The solution was cooled to room temperature and the solvent was evaporated under pressure to obtain a yellow crude product. It was purified by recrystallization in ethanol to give the light yellow solid L (0.689 g, 2.52 mmol, 62%). 1H NMR (500 MHz, DMSO) δ 9.95 (s, 1H), 8.82 (s, 1H), 8.41 (d, J = 8.6 Hz, 1H), 8.26 (d, J = 8.6 Hz, 1H), 7.51 (t, J = 7.8 Hz, 1H), 7.45 (d, J = 8.9 Hz, 3H), 7.16 (d, J = 7.5 Hz, 1H), 7.05 (d, J = 8.8 Hz, 2H), 3.81 (s, 3H). 13C NMR (126 MHz, DMSO) δ 159.71, 158.98, 154.63, 153.51, 143.89, 139.14, 137.65, 130.24, 129.76, 123.82, 119.15, 118.74, 115.58, 113.04, 56.30. ESI-mass (m/z): [L + H+]+: calcd: 279.31. Obsd: 279.27. Anal. calcd for C17H14N2O2: C, 73.37; H, 5.07; N, 10.07. Found: C, 73.29; H, 4.99; N, 10.01.
2.3. Analysis
The receptor could be dissolved in methanol. The stock solution for the different metal salts of Na+, K+, Ca2+, Mg2+, Ba2+, Al3+, Fe3+, Pb2+, Mn2+, Co2+, Ni2+, Sr2+, Zn2+, Sn2+, Cd2+, Hg2+, Cr3+, Ce3+ and Ag+ were prepared in water.
2.4. Job’s plot measurements
Receptor 1 (1.39 mg, 0.005 mmol) was dissolved in methanol (5 mL). 20, 18, 16, 14, 12, 10, 8, 6, 4 and 2 μL of the receptor solution were taken and transferred into vials. Each vial was diluted with methanol to make a total volume of 1.976 mL. AlCl3·6H2O (0.005 mmol) was dissolved in water (1 mL). 0, 2, 4, 6, 8, 10, 12, 14, 16, 18 and 20 μL of the Al3+ solution were added to each diluted receptor solution. Each vial had a total volume of 2 mL. After shaking the vials for a few minutes, fluorescence spectra were taken at room temperature.
2.5. Benesi–Hildebrand plot
The binding constant value was determined from the fluorescence intensity data using a modified Benesi–Hildebrand equation where F0, F and Fmax are the emission intensities of L in the absence of Al3+, intermediate Al3+ concentration and concentration of Al3+ of complete interaction, respectively; the plot of (Fmax − F0)/(F − F0) vs. 1/[Al3+] (Fig. 5) is the Benesi–Hildebrand plot.
2.6. pH study
pH buffer solution (20 mM) preparation: 2.384 g HEPES and 4.25 g NaNO3 were dissolved in pure water (500 mL volumetric flask), then 1 mol L−1 HCl and 1 mol L−1 NaOH were used to adjust the pH value to 7. The compound L (10 μM in methanol) and Al3+ (10 mM in water) were prepared in a cuvette, then the pH values (3.0, 4.0, 5.0, 6.0, 7.0, 8.0, 9.0, 10.0, 11.0, 12.0) were adjusted with 1 mol L−1 HCl and 1 mol L−1 NaOH (2 mL, constant volume with buffer).
2.7. Cell imaging studies
The living HeLa cells were provided by XiangYa Central Experiment Laboratory of Central South University (China).
HeLa cells were incubated with 10 μM of L in the culture medium at 37 °C for 30 min and washed with phosphate-buffered saline (PBS), followed by the addition of (0, 5, 10, 20 and 40 μM) Al3+ ions. Bright field and fluorescent images were captured at 20× magnification using an Olympus microscope (1 × 70) using Camedia software (Chicago, MI, USA) (E-20P 5.0 Megapixel).
3. Results and discussion
3.1. UV-Vis studies of compound L
The UV-Vis absorption spectra of compound L in the presence of various metal ions Na+, K+, Ca2+, Mg2+, Ba2+, Al3+, Fe3+, Pb2+, Mn2+, Co2+, Ni2+, Sr2+, Zn2+, Sn2+, Cd2+, Hg2+, Ag+, Cr3+ and Ce3+ were recorded in an alcoholic medium (CH3OH) using a U-3900 spectrophotometer. As seen in Fig. 1, the absorption spectrum of compound L exhibited two broad absorption bands at 271 nm and 358 nm. The position of absorption bands remained unchanged over the various metal ions except Sn2+ and Al3+ ions. Due to the complexation of Al3+ and L, a new absorption band at 258 nm was formed with Al3+, and the absorption intensity at 358 nm tends to decrease.
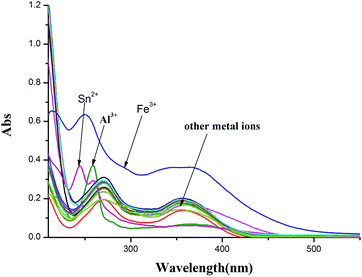 |
| Fig. 1 UV-Vis absorption spectra of compound L (10 μM) in the absence and presence of different metal ions (10 equiv.) such as Al3+, Fe3+, Ba2+, Ag+, Cd2+, Co2+, Sr2+, Hg2+, K+, Pb2+, Mg2+, Na+, Ca2+, Ni2+, Mn2+, Sn2+, Cr3+, Ce3+ and Zn2+ at room temperature. | |
The binding properties of L with Al3+ were studied using UV-Vis titrations in methanol solution (Fig. 2). Upon addition of increasing amounts of Al3+ (0–1.5 equiv.), while the absorption bands at 271 and 358 nm decreased gradually, the absorption band at 271 nm shifted to 258 nm. The blue-shifted absorption indicated that the phenol proton was not deprotonated.30 Moreover, there were clearly two isosbestic points at 256 and 396 nm, and the presence of two different isosbestic points for the sensor L suggested the formation of complex L–Al3+.31
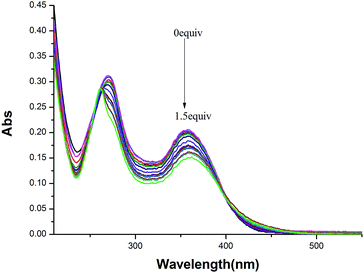 |
| Fig. 2 UV-Vis absorption spectra of compound L (10 μM) upon the titration of Al3+ (0, 0.1, 0.2, 0.3, 0.4, 0.5, 0.6, 0.7, 0.8, 0.9, 1.0, 1.2, 1.4 and 1.5 equiv.) at room temperature. | |
3.2. Fluorescence studies of compound L
3.2.1. Selectivity of compound L for Al3+ over other metal ions. For an excellent chemosensor, high selectivity is a matter of necessity. To confirm the selectivity of sensor L, the fluorescence behavior of (E)-4-methoxy-N-((8-methylquionline-2yl)methylene)aniline (L) upon the addition of various metal ions was investigated using a Hitachi F-4600 spectrophotometer. Quinoline Schiff base L did not show any significant emission band alone at 517 nm when the excitation wavelength was at 373 nm. After the addition of Na+, K+, Ca2+, Mg2+, Ba2+, Fe3+, Pb2+, Mn2+, Co2+, Ni2+, Sr2+, Zn2+, Sn2+, Cd2+, Hg2+, Ag+, Cr3+ and Ce3+ (Fig. 3), compound L exhibited no significant fluorescent enhancement. Only in the case of Al3+ ions compound L exhibited a more than 38-fold fluorescent enhancement. The quantum yields of L and its metal complex L–Al3+ were 0.016 and 0.62, respectively (Fig. 4).
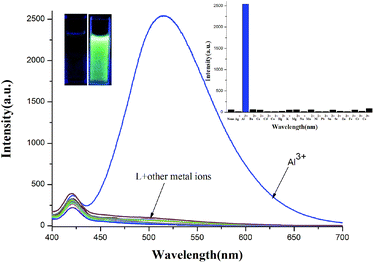 |
| Fig. 3 Fluorescence emission spectra of L (10 μM) in methanol in the presence of different metal ions (10 equiv.) such as Al3+, Fe3+, Ba2+, Ag+, Cd2+, Co2+, Sr2+, Hg2+, K+, Pb2+, Mg2+, Na+, Ca2+, Ni2+, Mn2+, Sn2+, Cr3+, Ce3+ and Zn2+. Excitation wavelength was at 373 nm. Inset (left): fluorescence intensity of compound L with Al3+ at 517 nm. Inset (right): the fluorescence intensity of different metal ions at 517 nm. | |
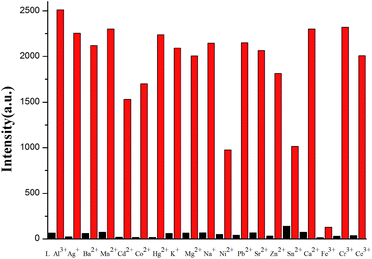 |
| Fig. 4 Selectivity of L for Al3+ ions in the presence of other competitive metal ions (10 equiv.) in methanol. Excitation wavelength and emission maximum are 373 nm and 517 nm, respectively. Black bars represent fluorescent intensity after the addition of 10 equiv. of the appropriate metal ions (Fe3+, Ba2+, Ag+, Cd2+, Co2+, Mn2+, Sn2+, Hg2+, K+, Pb2+, Mg2+, Na+, Ca2+, Ni2+, Sr2+, Cr3+, Ce3+ and Zn2+) in a 10 μM solution of L. Red bars represent the subsequent addition of same equiv. of Al3+ ions in each of the samples. | |
Interferences of cations were studied by treating L with 10 equiv. of Al3+ in the presence of 10 equiv. of other metal ions. As shown in Fig. 4, the response of compound L for Al3+ is not affected by competing metal ions (except Fe3+, as L and Fe3+ could form a stable chelate complex leading to fluorescence quenching), the fluorescent response of L–Al3+ is observed to be relatively reduced in the presence of Ni2+ and Sn2+ ions but is clearly detectable. From the results above, it was concluded that compound L had high selectivity and specificity for Al3+ over other metal ions.
3.2.2. Fluorescence titration of compound L with Al3+. The sensitivity of L for aluminum ions was examined with the fluorescence titration of different concentrations of Al3+ ions (0.0, 0.1, 0.2, 0.3, 0.4, 0.5, 0.6, 0.7, 0.8, 0.9, 1.0, 1.2 and 1.5 equiv.) at 517 nm (Fig. 5); the point which primarily indicates 1
:
1 stoichiometric complexation between compound L and Al3+ ions. The detection limit of L in recognizing Al3+ was found to be 1.2 × 10−7 M with the fluorescence titration spectra, which was lower than some reported Al3+ selective fluorescent sensors.32–36 It demonstrated that the detection limit was low enough for this sensor to detect and control Al3+ in environmental and biological systems (Table S1†).37–41
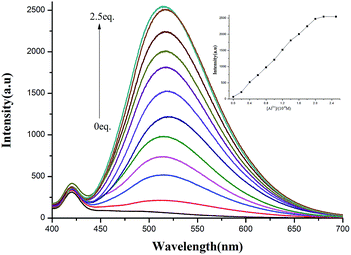 |
| Fig. 5 Fluorescence emission spectra at 517 nm of L upon addition of Al3+ (0, 0.1, 0.2, 0.3, 0.4, 0.5, 0.6, 0.7, 0.8, 0.9, 1.0, 1.2 and 1.5 equiv.) with an excitation of 373 nm, inset: fluorescence emission spectra of L upon addition of Al3+ (0, 0.1, 0.2, 0.3, 0.4, 0.5, 0.6, 0.7, 0.8, 0.9, 1.0, 1.2 and 1.5 equiv.) with an excitation of 373 nm and an emission of 517 nm. | |
3.3. Binding mode of compound L and Al3+
A proposed binding mode of L with Al3+ ions has been shown in Fig. 5 (inset), which primarily demonstrates a 1
:
1 stoichiometric complexation between sensor L and Al3+. These results are further confirmed by applying the emission Job’s plot method. The maximum fluorescence intensity appears at 0.5 mole fraction of Al3+ which indicates a 1
:
1 complexation between quinoline Schiff base L and Al3+ ions (Fig. 6). Furthermore, from the electrospray ionization mass spectra (ESI-MS) (Fig. S4†), a peak was observed at 335.59 [L + Al3+ + CH3OH − 2H+]+, which further supports a 1
:
1 binding stoichiometry between L and Al3+.
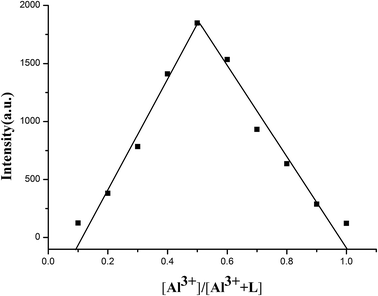 |
| Fig. 6 Job’s plot of L according to the method for continuous variations, indicating the 1 : 1 stoichiometry for L–Al3+ (the total concentration of L and Al3+ is 20 μM). | |
3.3.1. 1H NMR titration. In order to determine the coordination site, 1H NMR spectroscopy experiments were performed in the presence of Al3+ ions, with 0 to 2 equivalents of Al3+ added to compound L. As shown in Fig. 7, chemical shift changes were observed in the spectrum as the HC
N proton signal of L at 8.82 ppm shifted downfield to 8.86 ppm. Meanwhile, the hydroxyl hydrogen at 9.96 ppm shifted downfield to 10.16 ppm with the proton signal almost disappearing. The proton signals of the quinoline and benzene groups have been shifted, while a significant shift has been observed for the para-position hydrogen of nitrogen in the quinoline group. These spectrum changes demonstrated that nitrogen in the imine group, oxygen in the hydroxyl group and nitrogen in the quinoline group participated in the complexation with Al3+ ions.
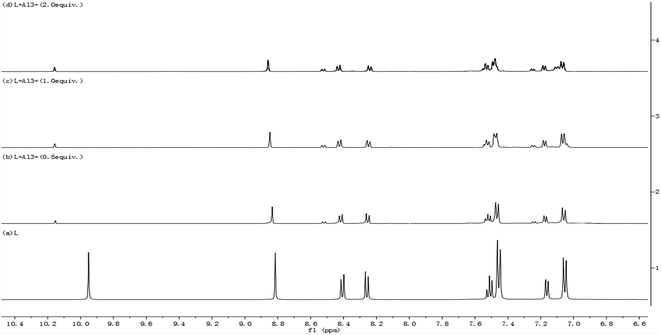 |
| Fig. 7 1H NMR (500 MHz) spectra of L with Al3+ (0.0–2.0 equiv.) in DMSO. | |
3.3.2. Calculations of association constants and detection limit. Association constants of L–Al3+ were calculated with the Benesi–Hildebrand equation through fluorescence titration:42–44 (Fig. S5†). |
 | (1) |
In this equation F is the fluorescence intensity at 517 nm at any given Al3+ concentration, F0 is the fluorescence intensity at 517 nm in the absence of Al3+, and Fmax is the maximal fluorescence intensity at 517 nm in the presence of Al3+ in solution. Data were fitted linearly as shown in Fig. 8. The association constant (Ka) for Al3+ binding in sensor L was determined to be 2.4 × 104 M−1. The detection limit (DL) of Al3+ using L was determined from the following equation: DL = K × SD/S, where K = 3; SD is the standard deviation of the blank solution; S is the slope of the calibration curve. DL = 1.2 × 10−7 M, which exhibits good selectivity of L with aluminum ions.
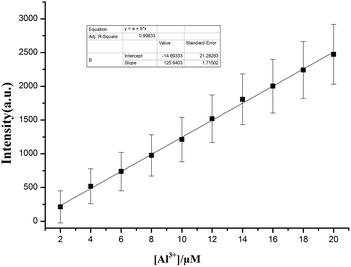 |
| Fig. 8 Plot of fluorescence intensity variation upon varying the concentration of Al3+ ion from 2–20 μM with an excitation of 373 nm and an emission of 517 nm. | |
3.3.3. Reversibility and the influence of solvents. The reversibility of sensor L was measured by the titration of EDTA with (L + Al3+). The fluorescence intensity was quenched thoroughly after the addition of EDTA (1.0 equiv.) (Fig. 9). The fluorescence emission intensity due to the L–Al3+ probe returned to a lower level for sensor L indicating the regeneration of free chemosensors L, quenching in fluorescence intensity.
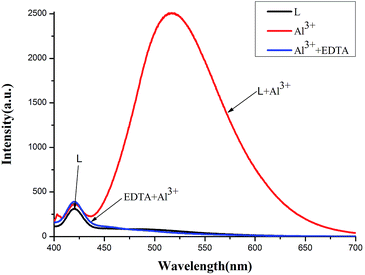 |
| Fig. 9 Fluorescence emission spectra of compound L (10 μM) in the presence of Al3+ ions (1.0 equiv.) or EDTA (1.0 equiv.) in methanol. | |
The effect of different solvents THF, DMSO, DMF, DCM, acetonitrile, ethanol and methanol on the detection of Al3+ by L was explored. It can be seen (Fig. 10) that the fluorescence was changed due to different binding modes with different solvents and the best result was observed with methanol.
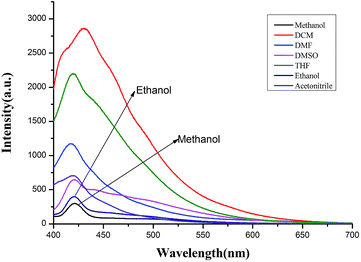 |
| Fig. 10 Effects on fluorescence intensity of L in different solvents methanol, DMSO, DMF, DCM, ethanol, THF and acetonitrile. | |
3.4. Sensing mechanism
As the recognition unit, Schiff bases have been widely used for the development of fluorescent sensors based on various sensing mechanisms. In this case, the Off–On properties of sensor L may operate by a photoinduced electron transfer (PET) mechanism. In the Off state, when the UV-light was created, the lone pair of electrons from the CH
N unit to the quinoline fluorophore quenches the fluorescence. In the presence of Al3+, the lone pair of electrons on the nitrogen atom is perturbed by the coordination of Al3+, which consequently suppresses the quenching process, resulting in fluorescence enhancement (On-state). This suggests that PET is a suitable mechanism for explaining the Off–On fluorescence for L after binding to Al3+ (Scheme 2).
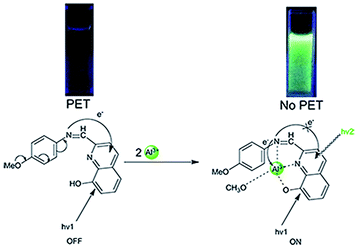 |
| Scheme 2 Proposed scheme of the fluorescence enhancement mechanism of compound L and Al3+. | |
3.5. Fluorescence imaging of intracellular L and Al3+
The effect of pH, in the range of pH 3.0–12.0, on the emission intensity of L was examined both in the absence and presence of Al3+ ions. It can be clearly seen from Fig. 11, that the emission intensity of L was significantly high in the pH region of 6.0–10.0 compared to that of L in the absence of Al3+ ions. Thus, L may be a suitable sensor for imaging Al3+ in living cells under physiological conditions. To further demonstrate the potential of L in monitoring Al3+ in living matrices, the Al3+ imaging behavior of the sensor L was studied using fluorescence microscopy using HeLa cells. HeLa cells were first incubated with various concentrations of Al3+ (0, 5, 10, 20 and 40 μM) and then exposed to L (10 μM) for 30 min at 37 °C, and images were taken (Fig. 12). The fluorescence emission might be due to the complex formation between Al3+ and the sensor L. These results confirm that L can be a suitable and biocompatible sensor to detect Al3+ in living cells.
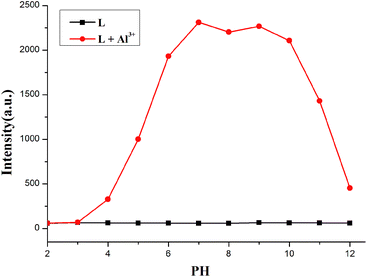 |
| Fig. 11 Fluorescence intensity of L, and L in the presence of Al3+ at various pH values. | |
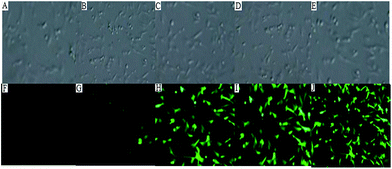 |
| Fig. 12 Representative fluorescence images of HeLa cells incubated with 10 μM L and 0 μM (A and F), 5 μM (B and G), 10 μM (C and H), 20 μM (D and I), and 30 μM (E and J) Al3+. The top images (A, B, C, D, and E) were observed using a light microscope and the bottom images were taken using a fluorescence microscope. The scale bar is 20 μm. | |
3.6. Determination of the fluorescence quantum yield
The quantum yield ϕ of sensor L and L + Al3+ complex45,46 were calculated by |
ϕx = ϕs (Fx/Fs)(As/Ax)(ηx/ηs)
| (2) |
Here, x and s indicate the unknown and standard samples, ϕ = quantum yield, F = integrated fluorescence intensity, A = absorbance, and η = refractive index of the solvent (rhodamine B is used as a standard in ethanol [ϕ = 0.69]). The quantum yield of L is 0.016, and on complexation with Al3+, the value significantly increases to 0.62.
4. Conclusion
In conclusion, we have synthesized a highly selective and sensitive Al3+ fluorescent sensor (E)-4-methoxy-N-((8-methylquionline-2yl)methylene)aniline (L). The fluorescence emission intensity of sensor L is remarkably enhanced by more than 38-fold after the addition of aluminum ions, and the association constant (Ka) and the detection limit (DL) were calculated to be 2.4 × 104 M−1 and 1.2 × 10−7 M, respectively. Moreover, the sensor L is attributed to the formation of a 1
:
1 complex, according to Job’s plot and electrospray ionization mass spectrometry (ESI-MS). The outstanding property of L is that the selectivity to Al3+ ions is not subject to interference by other mixed metal ions and the fluorescent nature of the L–Al3+ complex goes to “turn-On” in the presence of Al3+. It has been applied in a biological system to study living cell imaging. Therefore, Al3+ ions can be easily detected by fluorescent sensor L using fluorescence spectroscopy in biological systems.
Acknowledgements
We are grateful to the Nature Science Foundation of China for financial support (No. 21265010).
References
- D. P. Perl, D. C. Gajdusek, R. M. Garruto, R. T. Yanagihara and C. J. Gibbs, Intraneuronal aluminium accumulation in amyotrophic lateral sclerosis and Parkinsonism-dementia of Guam, Science, 1982, 217, 103–1055 Search PubMed.
- Y. W. Liu, C. H. Chen and A. T. Wu, A turn-on and reversible fluorescence sensor for Al3+ ion, Analyst, 2012, 137, 5201–5203 RSC.
- J. H. Lee, H. Y. Kim, S. J. Kim, J. Y. Noh, E. J. Song, C. Kim and J. H. Kim, Fluorescent dye containing phenol-pyridyl for selective detection of aluminum ions, Dyes Pigm., 2013, 96, 590–594 CrossRef CAS.
- O. Tavakoli and H. Yoshida, Effective recovery of harmful metal ions from squid wastes using subcritical and supercritical water treatments, Environ. Sci. Technol., 2005, 39, 2357–2363 CrossRef CAS PubMed.
- D. P. Perl and A. R. Brody, Alzheimer’s disease: X-ray spectrometric evidence of aluminum accumulation in neurofibrillary tangle bearing neurons, Science, 1980, 208, 297–299 CAS.
- G. D. Fasman, Aluminium and Alzheimer’s disease: model studies, Coord. Chem. Rev., 1996, 149, 125–165 CrossRef CAS.
- Y. Liu, P. Liang and L. Guo, Nanometer titanium dioxide immobilized on silica gel as sorbent for preconcentration of metal ions prior to their determination by inductively coupled plasma atomic emission spectrometry, Talanta, 2005, 68, 25–30 CrossRef CAS PubMed.
- J. S. Becker, A. Matusch, C. Depboylu, J. Dobrowolska and M. V. Zoriy, Quantitative imaging of selenium, copper, and zinc in thin sections of biological tissues (slugs–genus arion) measured by laser ablation inductively coupled plasma mass spectrometry, Anal. Chem., 2007, 79, 6074–6080 CrossRef CAS PubMed.
- A. P. S. Gonzales, M. A. Firmino, C. S. Nomure, F. R. P. Rocha, P. V. Oliveira and I. Gaubeur, Peat as a natural solid-phase for copper preconcentration and determination in a multicommuted flow system coupled to flame atomic absorption spectrometry, Anal. Chim. Acta, 2009, 636, 198–204 CrossRef CAS PubMed.
- D. G. Weller, A. J. Gutierrez, C. Rubio, C. Revert and A. Hardisson, Dietary intake of aluminum in a Spanish population (Canary Islands), J. Agric. Food Chem., 2010, 58, 10452–10457 CrossRef PubMed.
- B. Valeur and I. Leray, Design principles of fluorescent molecular sensors for cation recognition, Coord. Chem. Rev., 2000, 205, 3–40 CrossRef CAS.
- R. Sladek, A genome-wide association study identifies novel risk loci for type diabetes, Nature, 2007, 445, 881–885 CrossRef CAS PubMed.
- M. Arduini and P. Tecilla, Aluminium fluorescence detection with a FRET amplified chemosensor, Chem. Commun., 2003, 9, 1606–1607 RSC.
- Y. J. Lee, C. Lim, H. Suh, E. J. Song and C. Kim, A multifunctional sensor: chromogenic sensing for Mn2+ and fluorescent sensing for Zn2+ and Al3+, Sens. Actuators, B, 2014, 201, 535–544 CrossRef CAS.
- T. Mistri, R. Alam, R. Bhowmick, S. K. Mandal, M. Dolai, A. R. Khuda-Bukhsh and M. Ali, A simple rhodamine-based dual signalling reversible molecular switch for recognition of Al(III) with promising applications for advanced logic operations – ‘OR’, ‘Keypad Lock’ & ‘INHIBIT’ logic function and cell-imaging studies, Analyst, 2014 10.1039/c3an02255b.
- A. Kumar Neeraj, V. Kumar, R. Prajapati, S. K. Asthana, K. K. Upadhyay and J. Zhao, A remarkable effect of N,N-diethylamino functionality on the optoelectronic properties of a salicylimine-based probe for Al3+, Dalton Trans., 2014, 43, 5831–5839 RSC.
- S. Das, A. Sahana, A. Banerjee, S. Lohar, D. A. Safin, M. G. Babashkina, M. Bolte, Y. Garcia, I. Hauli, S. K. Mukhopadhyay and D. Das, Ratiometric fluorescence sensing and intracellular imaging of Al3+ ions driven by an intramolecular excimer formation of a pyrimidine–pyrene scaffold, Dalton Trans., 2013, 42, 4757–4763 RSC.
- S. Guha, S. Lohar, A. Sahana, A. Banerjee, D. A. Safin, M. G. Babashkina, M. P. Mitoraj, M. Bolte, Y. Garcia, S. K. Mukhopadhyay and D. Das, A coumarin-based “turn-on” fluorescent sensor for the determination of Al3+: single crystal X-ray structure and cell staining properties, Dalton Trans., 2013, 42, 10198–10207 RSC.
- S. Kim, J. Y. Noh, K. Y. Kim, J. H. Kim, H. K. Kang, S. W. Nam, S. H. Kim, S. Park, C. Kim and J. Kim, Salicylimine-based fluorescent chemosensor for aluminum ions and application to bioimaging, Inorg. Chem., 2012, 51, 3597–3602 CrossRef CAS PubMed.
- L. Fan, J. C. Qin, T. R. Li, B. D. Wang and Z. Y. Yang, A novel rhodamine chromone-based “Off–On” chemosensor for the differential detection of Al(III) and Zn(II) in aqueous solutions, Sens. Actuators, B, 2014, 203, 550–556 CrossRef CAS.
- L. Fan, T. R. Li, B. D. Wang, Z. Y. Yang and C. J. Liu, A colorimetric and turn-on fluorescent chemosensor for Al(III) based on a chromone Schiff-base, Spectrochim. Acta, Part A, 2014, 118, 760–764 CrossRef CAS PubMed.
- Y. W. Liu, C. H. Chen and A. T. Wu, A turn-on and reversible fluorescence sensor for Al3+ ion, Analyst, 2012, 137, 5201–5203 RSC.
- A. Kumar, V. Kumar and K. K. Upadhyay, An Al3+ and H2PO4−/HSO4− selective conformational arrest and bail to a pyrimidine–naphthalene anchored molecular switch, Analyst, 2013, 138, 1891–1897 RSC.
- H. Xiao, K. Chen, N. Jiang, D. Cui, G. Yin, J. Wang and R. Wang, A highly selective turn-on fluorescent probe for Al(III) based on coumarin and its application in vivo, Analyst, 2014, 139, 1980 RSC.
- X. Li, J. Chen and E. Wang, A highly selective and sensitive fluorescent chemosensor for detection of Al3+ in totally aqueous media, Chin. J. Chem., 2014, 32, 429–433 CrossRef CAS.
- R. Azadbakht, T. Almasi, H. Keypour and M. Rezaeivala, A new asymmetric Schiff base system fluorescent chemosensor for Al3+ ion, Inorg. Chem. Commun., 2013, 33, 63–67 CrossRef CAS.
- Y. J. Chang, P. J. Hung, C. F. Wan and A. T. Wu, A highly selective fluorescence turn-on and reversible sensor for Al3+ ion, Inorg. Chem. Commun., 2014, 39, 122–125 CrossRef CAS.
- Z. Liu, Y. Li, Y. Ding, Z. Yang, B. Wang, Y. Lic, T. Li, W. Luo, W. Zhu, J. Xie and C. Wang, Water-soluble and highly selective fluorescent sensor from naphthol aldehyde-tris derivate for aluminium ion detection, Sens. Actuators, B, 2014, 197, 200–205 CrossRef CAS.
- L. Chen, et al., Linear and nonlinear optical properties of Ln–Zn heteronuclear complexes from a Schiff base ligand containing 8-hydroxyquinoline moiety, Inorg. Chem. Commun., 2014, 47, 13–16 CrossRef CAS.
- V. K. Gupta, et al., A turn-on fluorescent chemosensor for Zn2+ ions based on antipyrine Schiff base, Sens. Actuators, B, 2014, 204, 507–514 CrossRef CAS.
- L. Yang, et al., Synthesis and spectral investigation of a turn-on fluorescence sensor with high affinity to Cu2+, Sens. Actuators, B, 2013, 176, 181–185 CrossRef CAS.
- H. S. Jung and J. S. Kim, Rationally designed fluorescence turn-on sensors: a new design strategy based on orbital control, Inorg. Chem., 2010, 49, 8552–8557 CrossRef CAS PubMed.
- M. Mameli, M. C. Aragoni and V. Lippolis, Synthesis and coordination properties of quinoline pendant arm derivatives of [9]aneN(3) and [9]aneN(2) S as fluorescent zinc sensors, Inorg. Chem., 2009, 48, 9236–9249 CrossRef CAS PubMed.
- D. Zhou, C. Y. Sun, C. Chen, X. N. Cui and W. J. Li, Research of a highly selective fluorescent chemosensor
for aluminum(III) ions based on photoinduced electron transfer, J. Mol. Struct., 2015, 1079, 315–320 CrossRef CAS.
- H. M. Park, B. N. Oh, J. H. Kim, W. Qiong, I. H. Hwang, K. D. Jung, C. Kim and J. Kim, Fluorescent chemosensor based-on naphthol–quinoline for selective detection of aluminum ions, Tetrahedron Lett., 2011, 52, 5581–5584 CrossRef CAS.
- W. H. Ding, D. Wang, X. J. Zheng, W. J. Ding, J. Q. Zheng, W. H. Mu, W. Cao and L. P. Jin, Sens. Actuators, B, 2015, 209, 359 CrossRef CAS.
- X. Sun, Ya-W. Wang and Y. Peng, A Selective and Ratiometric Bifunctional Fluorescent Probe for Al3+ Ion and Proton, Org. Lett., 2012, 14, 3420–3423 CrossRef CAS PubMed.
- V. D. Suryawanshi, et al., A novel pyrimidine derivative as a fluorescent chemosensor for highly selective detection of aluminum(III) in aqueous media, Spectrochim. Acta, Part A, 2013, 114, 681–686 CrossRef CAS PubMed.
- V. K. Gupta, et al., New “on–off” optical probe based on Schiff base responding to Al3+ ions: logic gate application, Sens. Actuators, B, 2015, 219, 218–231 CrossRef CAS.
- J. Lee, et al., Fluorescent dye containing phenol-pyridyl for selective detection of aluminum ions, Dyes Pigm., 2013, 96, 590–594 CrossRef CAS.
- Y. W. Choi, et al., A single Schiff base molecule for recognizing multiple metal ions: a fluorescence sensor for Zn(II) and Al(III) and colorimetric sensor for Fe(II) and Fe(III), Sens. Actuators, B, 2014, 194, 343–352 Search PubMed.
- K. B. Kim, D. M. You, J. H. Jeon, Y. H. Yeon, J. H. Kim and C. Kim, A fluorescent and colorimetric chemosensor for selective detection of aluminum in aqueous solution, Tetrahedron Lett., 2014, 55, 1347–1352 CrossRef CAS.
- H. A. Benesi and J. H. Hildebrand, A Spectrophotometric Investigation of the Interaction of Iodine With Aromatic Hydrocarbons, J. Am. Chem. Soc., 1949, 71, 2703–2707 CrossRef CAS.
- S.-P. Wu, T.-H. Wang and S.-R. Liu, A highly selective turn-on fluorescent chemosensor for copper(II) ion, Tetrahedron Lett., 2010, 66, 9655–9658 CrossRef CAS.
- J. N. Demas and G. A. Crosby, The measurement of photoluminescence quantum yields, J. Phys. Chem., 1971, 75, 991–1024 CrossRef.
- C. S. Choi, M. K. Kim, K. S. Jeon and K. H. Lee, A functionalized dianthryl tetraaza macrocycle having the recognizing and switching ability, J. Lumin., 2004, 109, 121–128 CrossRef CAS.
Footnote |
† Electronic supplementary information (ESI) available. See DOI: 10.1039/c5ra17557g |
|
This journal is © The Royal Society of Chemistry 2015 |