DOI:
10.1039/C5RA17535F
(Paper)
RSC Adv., 2015,
5, 93850-93857
Enantiomer separation of propranolol and tryptophan using bovine serum albumin functionalized silica nanoparticles as adsorbents†
Received
29th August 2015
, Accepted 26th October 2015
First published on 28th October 2015
Abstract
The immobilization of popular chiral selectors on the surface of nanomaterials to prepare new chiral adsorbents for preparative chiral separation is a research hotspot in separation science nowadays. In this study, bovine serum albumin (BSA) modified silica nanoparticles were prepared by using polydopamine (PDA) as a versatile multifunctional secondary reaction platform. The preparation method was facile, cost-effective and environmentally friendly. The new chiral adsorbents were then investigated for the separation of representative chiral drug enantiomers. For propranolol and tryptophan, the multi-step adsorption enhanced the chiral performance to a great degree. On increasing the starting percent enantiomeric excess (%, e.e.) of the enantiomeric mixtures, the %, e.e. value of the resulting solution increased to almost 100% under the same operating conditions. For simplicity and rapidness, the results of adsorption were measured by capillary electrophoresis (CE) using carboxymethyl-β-cyclodextrin (CM-β-CD) or α-cyclodextrin (α-CD) as additives into the background electrolyte solution. The experimental results also showed that the thus-prepared nanomaterials could be readily recycled at least three times, demonstrating their great stability and possibility in practical use.
1. Introduction
Chirality is a widespread phenomenon in nature and organisms.1 The separation of chiral components to pure enantiomers is of great importance to pharmaceutical, food, toxicological and clinical analysis. In general, a single pure isomer is the active component of one drug and the other isomer may be inactive, or even have dramatic, negative side effects.2 Many methods including HPLC,3 CE,4 membrane-based separation,5 preferential crystallization6 and asymmetric synthesis7 have been applied to separate, identify, and quantify chiral compounds. However, these methods are generally costly and time-consuming, especially when used for preparative purpose.
Nanoparticles (NPs) are referred to the particles that the size between 1 and 100 nm, which have gained significant interest and been applied in various areas such as physics, chemistry, material science, biology, etc. in recent years.8 NPs hold great promise for their small size, large surface area and pore volume when used as the adsorbent materials.9 In comparison with other NPs, such as polymer-based and metal NPs, silica-based NPs (SiNPs) own many advantages such as regular shapes, uniform sizes, good biocompatibility, no swelling in aqueous and organic solvents, and easy post-modification with different functional groups, etc.10
A large number of chiral selectors are currently available, among which cyclodextrins (CDs), proteins, chiral crown ethers, chiral surfactants, ligand-exchange complexes and linear polysaccharides are most popular.11–20 Nowadays, the hyphenation of classical chiral selectors with NPs to prepare new nanomaterials for chiral separation is intriguing, and has attracted much attention.21 Ghosh and coworkers, for example, synthesized magnetic silica nanoparticles, which were grafted with carboxymethyl-β-cyclodextrin (CM-β-CD) via carbodiimide activation. The adsorption behavior of certain chiral aromatic amino acid enantiomers on this material was then detailedly investigated.22 Similarly, Wu et al. fabricated and characterized teicoplanin-conjugated mesoporous silica magnetic nanoparticles (TE-MSMNPs) as novel chiral magnetic nano-selectors. It was experimentally shown that they were effective in the direct chiral separation of five racemic compounds in phosphate buffer.23 Although these materials are successful to some extents for chiral separation, multistep reactions and higher temperature were usually necessary in their preparation processes, which would inevitably result in rising costs and lowered batch to batch reproducibility. As a widely used chiral selector, BSA is known to be able to bind some drugs enantioselectively, due to its specific three-dimensional structure.24–26 Recently, Fu et al.27 reported the immobilization of BSA on the magnetic Fe3O4 nanoparticles through electrostatic adsorption interaction. Although they had proved to be useful for the separation of chiral drug enantiomers, the new materials are susceptive to recycle use due to the instable electrostatic interactions between BSA and NPs. So, the design and facile preparation of NPs-based chiral materials with good stability is still a highly desirable concern.
Since its emergence in 2007, polydopamine (PDA) has attracted much attention because it can be used as a versatile coating material on the surface of nearly all kinds of materials (inorganic or organic).28 PDA can also be used to create a variety of ad-layers (additional layer) for secondary reactions through Michael addition or Schiff base reactions.29–31 For example, Cao and coworkers prepared efficient oil/water separation mesh films by conjugating N-dodecyl mercaptan (NDM) with PDA film. The as-prepared PDA–NDM mesh was highly hydrophobic and superoleophilic, which could be used effectively for a large amount of oil/water separation.32 Iqbal et al. synthesized PDA-coated magnetic nanoparticles and polymethacrylic acid-co-ethylene glycol dimethacrylate submicron particles, then investigated their fast binding kinetics with environmentally hazardous compounds such as bisphenol A.33
The objective of this work is to develop a facile and mild way for the stable immobilization of chiral selectors on the surface of nanomaterials, and to realize their repeated use for preparative chiral separation. In the present stage, BSA was used as a model chiral selector. Through utilizing PDA coating as a versatile multifunctional secondary reaction platform, the new enantioselective materials of BSA functionalized SiNPs using PDA coating as a versatile multifunctional secondary reaction platform (SiO2@PDA@BSA) NPs were successfully prepared and used to produce optically pure drug enantiomers. For simplicity and rapidness, the results of adsorption were measured by capillary electrophoresis (CE) using CM-β-CD or α-CD as additives into the background electrolyte solution.34,35 One-step or multi-step operations using the new adsorbents were optimized to obtain high %, e.e. As increasing the adsorption times and the starting %, e.e. of enantiomeric mixtures, the final %, e.e. value of the resulting solution was up to nearly 100%, demonstrating the validity of the new materials in preparative enantioseparation.
2. Experimental
2.1 Reagents and chemicals
All chemicals were analytical grade unless noted otherwise. Double-distilled water (DDW) purified by a Nanopure II system (Barnstead, USA) was used throughout the experiment. Tetraethoxysilane (TEOS) (98%) was purchased from Guotai-Huarong New Chemical Materials (Zhangjiagang, China). Ammonia (25, wt%), methanol ethanol, acetonitrile, thiourea were purchased from Tianda Kewei (Tianjin, China). Phosphoric acid (85%), tris(hydroxymethyl) aminomethane, potassium hydroxide, potassium dihydrogen phosphate and hydrochloric acid were from Tianjin Guangfu Chemicals (Tianjin, China). Dopamine hydrochloride (DA) (99%) was obtained from Tianjin Heowns Biochem LLC (Tianjin, China). Bovine serum albumin (BSA, MW 68
000) was purchased from Solarbio Life Science (Beijing, China) and stored at 4 °C. Carboxymethyl-β-cyclodextrin (CM-β-CD, >98%, molecular weight ≈ 1551, degree of substitution ≈ 7.05) was purchased from Zhiyuan Bio-Technology Co., Ltd. (Binzhou, China). α-Cyclodextrin (α-CD) was purchased from Aladdin Industrial Corporation (Shanghai, China). L-Tryptophan (L-Trp, >98%), D-tryptophan (D-Trp, >98%) were purchased from TCI (Shanghai) Development Co., Ltd. (Shanghai, China). Propranolol tablets were purchased from Tianjin Lisheng Pharmaceutical Co., Ltd. (Tianjin, China). The purity is 10 mg per tablet. Stock solution of propranolol was extracted from tablets with methanol. The concentration of stock solution was 5.8 mg mL−1. Stock solutions were stored in the dark at 4 °C.
2.2 Apparatus
TEM were carried out on a Philips Tecnai G20 at 200 kV (PHG, Amsterdam, Netherlands). The samples were prepared by dipping micro grid membrane coated copper grids (300-mesh) into the dispersion of NPs in ethanol. Elemental analysis was performed by elemental analyzer (vario EL CUBE, Germany) and the zeta potential of the functionalized silica NPs were determined with Zetasizer Nano ZS equipments (Malvern Instruments, Worcestershire, UK). All CE experiments were carried out on a TH-3000 HPCE-HPLC amphibious system equipped with a UV detector and CXTH-3000 data handling software (Tianhui Instruments, Baoding, China). A QL-901 vortex (Haimen Kylin-Bell Lab Instruments Co., Jiangsu, China) were used to disperse NPs in sample solutions. A TG16-WS centrifuge (Hunan Xiangyi Lab Instruments Co., Changsha, China) was used to centrifuge the samples. The optical rotation of samples before and after adsorption process were estimated on an autopol II automatic polarimeter (Rudolph Research Analytical, USA) with a 10 cm sample tube under a sodium lamp (λ = 589 nm). Fused-silica capillaries of 375 μm o.d. and 75 μm i.d., 50 μm i.d. (Yongnian Optic Fiber Co, Hebei, China) were used throughout the experiment.
A capillary with the total length of 38 cm and the effective length of 28 cm was made by scraping off 3–5 mm of the polymer outside the capillary at an appropriate place. Prior to the first use, the capillary was successively rinsed by methanol, DDW, 1 mol L−1 NaOH, DDW, 1 mol L−1 HCl, DDW and phosphate buffer solution (PBS) for 20 min each.
2.3 Synthesis of the functionalized SiNPs
2.3.1 Preparation of SiNPs. SiNPs were prepared by the modified Stöber method.36 Briefly, solution consisting of 2.0 mL TEOS (∼9 mmol) in 50 mL of ethanol was firstly prepared. To this solution at 40 °C, a mixture of NH4OH (2.0 mL) and DDW (1.4 mL) was added dropwise with vigorous stirring. After 18 h of reaction, the particles thus formed were centrifuged and washed with ethanol and DDW repeatedly, and then vacuum-dried at 70 °C for 3 h.
2.3.2 Synthesis of PDA coated SiNPs (SiO2@PDA). The prepared SiNPs (∼0.5 g) were added to a conical flask in the presence of 36 mL of 10 mM tris–HCl buffer solution (pH 8.5) by slow stirring at ambient temperature. Then 80.0 mg of DA dissolved into 4 mL 90% ethanol was slowly dropped into the above solution. After 3 h of reaction, the particles thus formed were centrifuged and washed with tris–HCl buffer solution, DDW and ethanol for several times to remove the physically attached DA and residual unattached DA, and then vacuum-dried at 70 °C for 3 h.
2.3.3 Synthesis of BSA functionalized SiNPs (SiO2@PDA@BSA). BSA was grafted onto the PDA-coated SiNPs surfaces via Michael addition or Schiff base reactions.37 Briefly, 0.4 g SiO2@PDA nanoparticles and 5 mg mL−1 BSA were added to a conical flask in the presence of 40 mL of 20 mM phosphate buffer solution with different pH (7, 7.17 and 7.4) by slow stirring at 35 °C for 24 h. The obtained nanoparticles were centrifuged and washed with PBS and DDW for several times to remove the unbound residues, and then freeze-dried for 24 h and stored at 4 °C.
2.4 Direct enantiomer separation of chiral compounds by SiO2@PDA@BSA
Direct enantiomer separations of propranolol and tryptophan were carried out by using SiO2@PDA@BSA NPs as the enantioselective adsorbents. For one-step operation, a certain amount of SiO2@PDA@BSA NPs were mixed with propranolol (or tryptophan solution) in PBS buffer. The mixture was ultrasonicated and vortexed for 5 min each, and then centrifuged (10
000 rpm) for 10 min, the supernatant solution thus obtained was analyzed by chiral CE using CM-β-CD (for propranolol) and α-CD (for tryptophan) as chiral additives. For the multi-step operation, SiO2@PDA@BSA NPs were used in batches, and the supernatants for each step were saved and used as the feed solution for the next step. The final solution was collected in a sample tube for subsequent CE analysis. The results of adsorption could be expressed by enantiomeric excess (%, e.e.),
where %, e.e. refers to enantiomeric excess, [R], [S] is the concentration of R-enantiomer and S-enantiomer, respectively.27 Since chiral CE is used for the analysis, peak areas can be directly used to calculate %, e.e. instead of concentration.
3. Results and discussion
3.1 Synthesis of BSA functionalized nanoparticles
3.1.1 Optimization of synthesis conditions. In this study, we used a new way to synthesize SiO2@PDA NPs. The conventional method is simple immersion of substrates in a dilute aqueous solution of DA, and then buffered to a pH typical of marine environments (2 mg of DA per milliliter of 10 mM tris–HCl, pH 8.5), resulting in spontaneous deposition of a thin adherent polymer film.28 By contrast, in our method the DA was firstly dissolved into 90% ethanol, then it is slowly dropped into tris buffer. The resulting PDA coatings are much more homogeneous and more likely to be coated on the surface of SiNPs instead of forming a sheet precipitate of PDA self-polymerization. The reason is that adding organic solvents may slow down the speed of DA polymerization, so that PDA could be better coated on the surface of SiNPs.The pH of buffer solution is an important factor on the synthetic progress of NPs. The obtained SiO2@PDA@BSA NPs from different pH (7.00, 7.17 and 7.40) were used for multi-step adsorption of propranolol (three times), respectively. The supernatants for each step were saved and used as the feed solution for the next step. The results are as shown in Fig. 1. From this figure, we can see that the NPs synthesized in pH 7.17 showed the best results. Optimum synthetic condition of pH 7.17 is so selected for the further work.
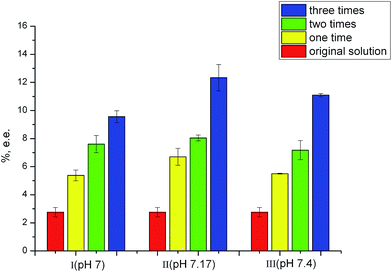 |
| Fig. 1 The effect of buffer solution pH on synthesis SiO2@PDA@BSA NPs. (I) pH 7 (II) pH 7.17 (III) pH 7.4. The other synthesis conditions are mentioned in Section 2.3.2. CE conditions: 30 mmol L−1 phosphate background electrolyte (pH 6.0) containing 1.2 mmol L−1 CM-β-CD, 5% (v : v) acetonitrile. Detection wavelength, 214 nm; 75 μm i.d.; injection, 6 kV × 3 s for propranolol; separated voltage, +6 kV. | |
3.1.2 Characterization of functionalized SiNPs. TEM images of three kinds of NPs (SiNPs, SiO2@PDA NPs, SiO2@PDA@BSA NPs) are shown in Fig. 2. It can be observed that these nanoparticles are sphere-like and uniform in shape with the size of approximately 150 nm, although having different functional groups on the surfaces.
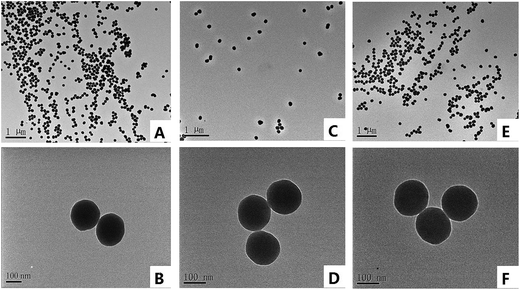 |
| Fig. 2 TEM images of SiO2 NPs (A and B), SiO2@PDA NPs (C and D) and SiO2@PDA@BSA NPs (E and F). | |
The results of elementary analysis of pure silica NPs and two functionalized silica NPs are summarized in Table 1. In comparison with pure silica NPs, the contents of three elements (C, H, N) in SiO2@PDA NPs and SiO2@PDA@BSA NPs are all increased, indicating that amino and alkyl groups have been successfully bonded onto silica NPs during the synthesis process. There is a little S element in BSA, from the content of S in SiO2@PDA@BSA NPs, it is easy to see that BSA were successfully modified on the silica NPs.
Table 1 Elementary analysis of pure silica NPs and two functionalized silica NPs
NPs |
C%/RSD% (n = 3) |
H%/RSD% (n = 3) |
N%/RSD% (n = 3) |
S%/RSD% (n = 3) |
SiO2 |
1.74/0.036 |
1.49/0.081 |
0.61/0.028 |
— |
SiO2@PDA |
2.46/0.180 |
1.50/0.053 |
0.66/0.017 |
— |
SiO2@PDA@BSA |
3.66/0.021 |
1.62/0.007 |
0.74/0.014 |
0.18/0.014 |
The measurement of the zeta potential of the aqueous suspension of NPs is an indirect but useful way to confirm the existence of functional groups on the surface of NPs. Zeta potential of the aqueous suspensions of NPs can be affected by a number of factors including pH, ion concentration and charges of ions. Fig. S1† shows the curves of the zeta potential vs. pH. In the pH range of 2.0–8.0, SiO2@PDA@BSA NPs have more positive zeta potentials than those of pure SiO2 NPs and SiO2@PDA NPs. The positive surface charge provided by amino group also results in a shift of the isoelectric point (IEP).
3.2 Optimization of direct enantiomer separation of chiral compounds
3.2.1 Effect of sample solution (pH and concentration of buffer). The acidity of the sample solution is a critical parameter affecting adsorption efficiency, since it determines the analytes ionic state as well as the surface charge of the adsorbent. In the case of propranolol, we mixed 3 mg mL−1 BSA functionalized NPs and propranolol solution using different pH. To investigate the influence of pH on adsorption, considering the pH-dependent stereoselectivity and activity of the immobilized BSA on NPs, pH values ranging from 6 to 8 were examined in this work and the results are as shown in Fig. 3. From Fig. 3, we can see that after adsorption by NPs at pH 7, the enantiomeric excess of the resulting solutions is 6.70% in R-propranolol, which is higher than other pHs. This might be due to well-maintained BSA conformation which is required for chiral discriminations. Optimum pH of 7 was so selected for the further work. The concentrations of PBS buffer were investigated in the range of 10–50 mmol L−1 (Fig. S2†). 30 mmoL L−1 PBS buffer was chosen as the optimal condition under which the highest %, e.e. was obtained.
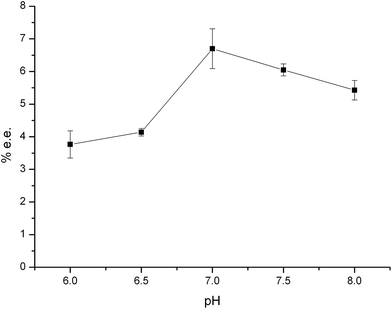 |
| Fig. 3 The effect of sample solution pH on adsorption of propranolol. Experimental conditions: 0.58 mg mL−1 racemate propranolol, 30 mmol L−1 phosphate buffer with 3 mg mL−1 SiO2@PDA@BSA NPs, ultrasonication for 5 min and vortex for 5 min. CE conditions are the same as in Fig. 1. | |
3.2.2 Effect of adsorption time and mode. The effect of adsorption time on the enantioseparation efficiency for propranolol was studied from 5 to 25 min. As shown in Fig. S3,† results indicated that enantioseparation efficiency increased when the adsorption time increased from 5 to 10 min. However, when time was longer than 10 min, the enantioseparation efficiency decreased slightly. After 25 min adsorption, enantioseparation efficiency increased slightly again. It seems that the analytes bind strongly with the sorbent and the distribution equilibrium was easy to achieve in 10 min. However, the analytes could not be pulled down easily from the sorbent by desorption solvent with the further increase in adsorption time. Therefore, the adsorption time was selected as 10 min.Three different processes for sample agitation during the adsorption step were evaluated. As shown in Fig. S4,† experimental results showed that adsorption efficiency obtained only by ultrasonication or vortex was lower than that by a mix of them. Ultrasonication is beneficial for the homogeneous dispersion of NPs in solution, which enables the analytes to interact fully with the sorbent. However, too long ultrasonication will result in the increase in temperature of sample solution, and so the desorption of analytes from sorbents. Only vortex was disadvantageous to the dispersion of NPs. Ultimately, a procedure of ultrasonication for 5 min and then vortex for 5 min was selected.
3.2.3 Direct enantiomeric separation of chiral compounds with one-step or multi-step operations. Direct chiral separation of propranolol and tryptophan was carried out to investigate the chiral recognition abilities of the BSA functionalized NPs with one-step or multi-step operations. In one-step operation, we mixed 0.58 mg mL−1 propranolol solution or 0.1 mg mL−1 tryptophan solution using different amount of chiral selectors at pH 7.0. As shown in Fig. 4A and B, after adsorption, the enantiomeric excess of the two compounds improved to different degrees.
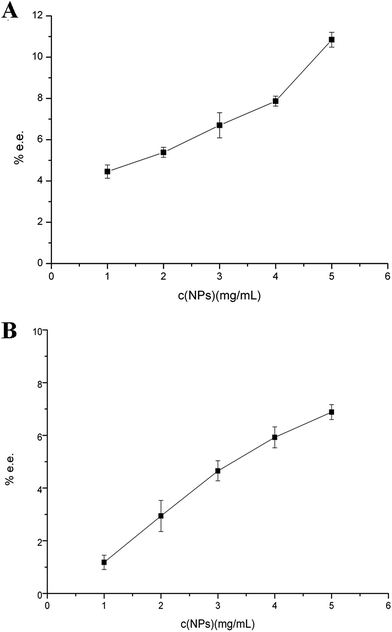 |
| Fig. 4 Direct enantiomer separation of compounds (A) 0.58 mg mL−1 propranolol (B) 0.1 mg mL−1 tryptophan with one-step operation at pH 7.0 (30 mmol L−1 phosphate buffer) by different amount of SiO2@PDA@BSA NPs, ultrasonication for 5 min and vortex for 5 min. CE conditions of propranolol are the same as in Fig. 1. CE conditions of tryptophan: 20 mmol L−1 phosphate background electrolyte (pH 2.5) containing 40 mmol L−1 α-CD, 2% (v : v) methanol. Detection wavelength, 254 nm; 50 μm i.d.; injection, 15 kV × 3 s for propranolol; separated voltage, +15 kV. | |
To improve the enantioseparation efficiency, we performed the stereoselective adsorption process in a mode of multi-step operation. As shown in Fig. 5A and B, in the multi-step operation, the chiral performance of tryptophan is significantly enhanced with increasing the number of operations and the enantiomeric excess is up to 14.50% and with five operational steps. Moreover, the increase in the enantioselectivity with the number of operations is also observed for propranolol to give enantiomeric excess up to 11.71% with five-step adsorption. The electropherograms of above resulting solution is shown in Fig. S5.†
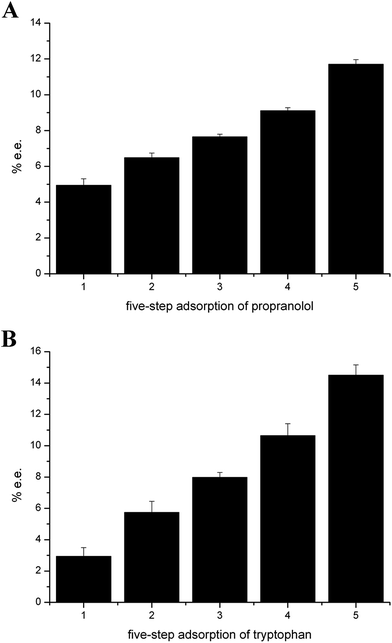 |
| Fig. 5 Direct enantiomer separation of chiral compounds (A) 0.58 mg mL−1 propranolol (B) 0.1 mg mL−1 tryptophan with multi-step operation at pH 7.0 (30 mmol L−1 phosphate buffer) by 2 mg mL−1 SiO2@PDA@BSA NPs, ultrasonication for 5 min and vortex for 5 min. CE conditions are the same as in Fig. 4. | |
Then, to establish enantioseparation using BSA functionalized NPs, we utilized multi-step adsorption to achieve enantiomeric enrichment of D-tryptophan starting from high initial %, e.e. (75.55%) as shown in Fig. 6. We used 4 mg mL−1 NPs mixed with ∼0.1 mg mL−1 tryptophan solution (L-tryptophan 0.015 mg mL−1 and D-tryptophan 0.09 mg mL−1), after adsorption, %, e.e. of the resulting solution is up to almost 100%. That is to say, the sample was purified.
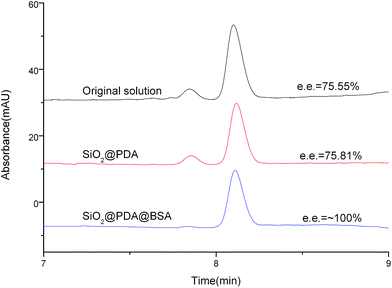 |
| Fig. 6 CE analysis of the resulting solutions of 0.1 mg mL−1 tryptophan after three-step operation at pH 7.0 (30 mmol L−1 phosphate buffer) by 4 mg mL−1 SiO2@PDA@BSA NPs or SiO2@PDA NPs, ultrasonication for 5 min and vortex for 5 min. CE conditions are the same as in Fig. 4. | |
The chiral recognition abilities of the BSA functionalized NPs were further confirmed by polarimetry. The resulting solutions in Fig. 6 were also carried out by an automatic digital polarimeter. As shown in Table 2, these results were in agreement with those obtained by the measurement of CE.
Table 2 Chiral separation of tryptophan
Samples |
The specific rotation ([α]25D, 0.1 mg mL−1 in 3 mL of solvents) |
Original solution |
+0.216 |
SiO2@PDA adsorption |
+0.216 |
SiO2@PDA@BSA adsorption |
+0.286 |
SiO2@PDA@BSA adsorption (blank solution) |
+0.000 |
3.3 Recycle of BSA functionalized NPs
In practical applications, reusability is a very important property for adsorbent. The adsorption and regeneration cycle was repeated for four times for the same batch of BSA functionalized NPs to test their reusability. Collection of the analytes after centrifugation and then washing 15 min with PBS (pH 7.0, 30 mM) by ultrasonication, the NPs could be recycled. As shown in Fig. S6,† the chiral recognition abilities of these BSA functionalized NPs remained almost constant and with little loss of activity after they were reused at least three times.
4. Conclusions
In this study, SiO2@PDA@BSA NPs were facilely prepared by using PDA coating as a versatile multifunctional secondary reaction platform. The use of these BSA modified NPs as adsorbents for the preparative enantioseparation of drugs was then presented with satisfactory results. As increasing the starting percent enantiomeric excess (%, e.e.) of enantiomeric mixtures, the final %, e.e. value of the resulting solution was up to nearly 100% by using a multi-step adsorption strategy, demonstrating its validity in preparative enantioseparation, especially for the products from asymmetric synthesis. Comparing with other nanoparticles-based chiral adsorbents,22,23,27 besides simplicity and environment friendness, another advantage of the preparation process is the stability of the final materials, which had exhibited the ability for recycling use for at least three times.
Further work will be focused on the use of the strategy to prepare other chiral selectors-modified nanomaterials and use them as adsorbents for the preparative separation of chiral drugs of interest. To further simplify the adsorption process, superparamagnetic character will be incorporated in the preparation process simultaneously.
Conflict of interest
The authors have declared no conflict of interest.
Acknowledgements
Financial support from National Natural Science Foundation of China (21275081) and National Basic Research Program of China (2011CB707703) is gratefully acknowledged.
References
- S. Kröger, Y. F. Wong, S. T. Chin, J. Grant, D. Lupton and P. J. Marriott, Evaluation of reversible interconversion in comprehensive two-dimensional gas chromatography using enantioselective columns in first and second dimensions, J. Chromatogr. A, 2015, 1404, 104–114 CrossRef PubMed.
- T. Duerinck and J. F. M. Denayer, Metal–organic frameworks as stationary phases for chiral chromatographic and membrane separations, Chem. Eng. Sci., 2015, 124, 179–187 CrossRef CAS.
- Q. Zhang, H. F. Zou, H. L. Wang and J. Y. Ni, Synthesis of a silica-bonded bovine serum albumin s-triazine chiral stationary phase for high-performance liquid chromatographic resolution of enantiomers, J. Chromatogr. A, 2000, 866, 173–181 CrossRef CAS PubMed.
- H. F. Li, H. L. Zeng, Z. F. Chen and J. M. Lin, Chip-based enantioselective open-tubular capillary electrochromatography using bovine serum albumin–gold nanoparticle conjugates as the stationary phase, Electrophoresis, 2009, 30, 1022–1029 CrossRef CAS PubMed.
- M. Nakamura, S. Kiyohara, K. Salto, K. Sugita and T. Sugo, Chiral separation of DL-tryptophan using porous membranes containing multilayered bovine serum albumin crosslinked with glutaraldehyde, J. Chromatogr. A, 1998, 822, 53–58 CrossRef CAS PubMed.
- M. Monier, D. A. Abdel-Latif and H. M. Nassef, Preparation of L-tryptophan imprinted microspheres based on carboxylic acid functionalized polystyrene, J. Colloid Interface Sci., 2015, 445, 371–379 CrossRef CAS PubMed.
- F. F. Zheng, Q. Y. Zhang, J. Y. Li, J. J. Suo, C. C. Wu, Y. M. Zhou, X. Q. Liu and L. Xu, Machine learning induction of chemically intuitive rules for the prediction of enantioselectivity in the asymmetric syntheses of alcohols, Chemom. Intell. Lab. Syst., 2015, 145, 39–47 CrossRef CAS.
- P. Foroozandeh and A. A. Aziz, Merging Worlds of Nanomaterials and Biological Environment: Factors Governing Protein Corona Formation on Nanoparticles and Its Biological Consequences, Nanoscale Res. Lett., 2015, 10, 221–232 CrossRef PubMed.
- H. F. Liang and Z. C. Wang, Adsorption of bovine serum albumin on functionalized silica-coated magnetic MnFe2O4 nanoparticles, Mater. Chem. Phys., 2010, 124, 964–969 CrossRef CAS.
- S. Fanali, P. Catarcini, G. Blaschke and B. Chankvetadze, Enantioseparations by capillary electrochromatography, Electrophoresis, 2011, 22, 3131–3151 CrossRef.
- A. Z. M. Badruddoza, K. Hidajat and M. S. Uddin, Synthesis and characterization of β-cyclodextrin-conjugated magnetic nanoparticles and their uses as solid-phase artificial chaperones in refolding of carbonic anhydrase bovine, J. Colloid Interface Sci., 2010, 346, 337–346 CrossRef CAS PubMed.
- R. Chalasani and S. Vasudevan, Cyclodextrin functionalized magnetic iron oxide nanocrystals: a host-carrier for magnetic separation of non-polar molecules and arsenic from aqueous media, J. Mater. Chem., 2012, 22, 14925–14931 RSC.
- Y. Xiao, S. C. Ng, T. T. Y. Tan and Y. Wang, Recent development of cyclodextrin chiral stationary phases and their applications in chromatography, J. Chromatogr. A, 2012, 1269, 52–68 CrossRef CAS PubMed.
- T. T. Hong, C. J. Chi and Y. B. Ji, Pepsin-modified chiral monolithic column for affinity capillary electrochromatography, J. Sep. Sci., 2014, 37, 3377–3383 CrossRef CAS PubMed.
- L. Z. Liu, C. H. He, L. Yang, Y. Huang, Q. Wu, W. G. Duan, H. S. Wang and Y. Y. Pan, Novel C1-symmetric chiral crown ethers bearing rosin acids groups: synthesis and enantiomeric recognition for ammonium salts, Tetrahedron, 2014, 70, 9545–9553 CrossRef CAS.
- O. Koji and T. Shigeru, Enantiomer separation of drugs by micellar electrokinetic chromatography using chiral surfactants, J. Chromatogr. A, 2000, 875, 163–178 CrossRef.
- S. Vidyasankar, M. Ru and F. H. Arnold, Molecularly imprinted ligand-exchange adsorbents for the chiral separation of underivatized amino acids, J. Chromatogr. A, 1997, 775, 51–63 CrossRef CAS.
- B. Chankvetadze, Recent developments on polysaccharide-based chiral stationary phases for liquid-phase separation of enantiomers, J. Chromatogr. A, 2012, 1269, 26–51 CrossRef CAS PubMed.
- J. Lee, J. T. Lee, W. L. Watts, J. Barendt, T. Q. Yan, Y. Huang, F. Riley, M. Hardink, J. Bradow and P. Franco, On the method development of immobilized polysaccharide chiral stationary phases in supercritical fluid chromatography using an extended range of modifiers, J. Chromatogr. A, 2014, 1374, 238–246 CrossRef CAS PubMed.
- X. Y. Yang, L. Su, X. B. Hou, S. Y. Ding, W. F. Xu, B. H. Wang and H. Fang, High-performance liquid chromatographic enantioseparation of 3,5-disubstituted hydantoins analogs and temperature-induced reversals of elution orders on a polysaccharide-based chiral stationary phase, J. Chromatogr. A, 2014, 1355, 291–295 CrossRef CAS PubMed.
- M. J. Paik, J. S. Kang, B. S. Huang, J. R. Carey and W. Lee, Development and application of chiral crown ethers as selectors for chiral separation in high-performance liquid chromatography and nuclear magnetic resonance spectroscopy, J. Chromatogr. A, 2013, 1274, 1–5 CrossRef CAS PubMed.
- S. Ghosh, A. Z. M. Badruddoza, M. S. Uddin and K. Hidajat, Adsorption of chiral aromatic amino acids onto carboxymethyl-β-cyclodextrin bonded Fe3O4/SiO2 core–shell nanoparticles, J. Colloid Interface Sci., 2011, 354, 483–492 CrossRef CAS PubMed.
- J. W. Wu, P. Su, J. Huang, S. M. Wang and Y. Yang, Synthesis of teicoplanin-modified hybrid magnetic mesoporous silica nanoparticles and their application in chiral separation of racemic compounds, J. Colloid Interface Sci., 2013, 399, 107–114 CrossRef CAS PubMed.
- T. T. Hong, Y. Zheng, W. W. Hu and Y. B. Ji, Preparation and evaluation of bovine serum albumin immobilized chiralmonolithic column for affinity capillary electrochromatography, Anal. Biochem., 2014, 464, 43–50 CrossRef CAS PubMed.
- G. Gübitz and M. G. Schmid, Chiral separation by capillary electromigration techniques, J. Chromatogr. A, 2008, 1204, 140–156 CrossRef PubMed.
- G. Gübitz and M. G. Schmid, Chiral Separation Principles in Chromatographic and Electromigration Techniques, Mol. Biotechnol., 2006, 32, 1073–6085 Search PubMed.
- Y. Fu, T. T. Huang, B. Chen, J. Shen, X. L. Duan, J. L. Zhang and W. Li, Enantioselective resolution of chiral drugs using BSA functionalized magnetic nanoparticles, Sep. Purif. Technol., 2013, 107, 11–18 CrossRef CAS.
- H. Lee, S. M. Dellatore, W. M. Miller and P. B. Messersmith, Mussel-Inspired Surface Chemistry for Multifunctional Coatings, Science, 2007, 318, 426–430 CrossRef CAS PubMed.
- R. P. Liang, X. N. Wang, C. M. Liu, X. Y. Meng and J. D. Qiu, Facile preparation of protein stationary phase based on polydopamine/graphene oxide platform for chip-based open tubular capillary electrochromatography enantioseparation, J. Chromatogr. A, 2014, 1323, 135–142 CrossRef CAS PubMed.
- Y. L. Liu, K. L. Ai and L. H. Lu, Polydopamine and Its Derivative Materials: Synthesis and Promising Applications in Energy, Environmental, and Biomedical Fields, Chem. Rev., 2014, 114, 5057–5115 CrossRef CAS PubMed.
- X. B. Yin and D. Y. Liu, Polydopamine-based permanent coating capillary electrochromatography for auxin determination, J. Chromatogr. A, 2008, 1212, 130–136 CrossRef CAS PubMed.
- Y. Z. Cao, X. Y. Zhang, L. Tao, K. Li, Z. X. Xue, L. Feng and Y. Wei, Mussel-Inspired Chemistry and Michael Addition Reaction for Efficient Oil/Water Separation, ACS Appl. Mater. Interfaces, 2013, 5, 4438–4442 CAS.
- Z. Iqbal, S. Alsudir, M. Miah and E. P. C. Lai, Rapid CE-UV binding tests of environmentally hazardous compounds with polymer-modified magnetic nanoparticles, Electrophoresis, 2011, 5, 2181–2187 CrossRef PubMed.
- Z. S. Gong, L. P. Duan and A. N. Tang, Amino-functionalized silica nanoparticles for improved enantiomeric separation in capillary electrophoresis using carboxymethyl-β-cyclodextrin (CM-β-CD) as a chiral selector, Microchim. Acta, 2015, 182, 1297–1304 CrossRef CAS.
- S. Fanali, Chiral separations by CE employing CDs, Electrophoresis, 2009, 30, S203–S210 CrossRef PubMed.
- C. Y. Yue, G. S. Ding, F. J. Liu and A. N. Tang, Water-compatible surface molecularly imprinted silica nanoparticleas pseudostationary phase in electrokinetic chromatography for the enantioseparation of tryptophan, J. Chromatogr. A, 2013, 1311, 176–182 CrossRef CAS PubMed.
- C. M. Liu, R. P. Liang, X. N. Wang, J. W. Wang and J. D. Qiu, A versatile polydopamine platform for facile preparation of protein stationary phase for chip-based open tubular capillary electrochromatography enantioseparation, J. Chromatogr. A, 2013, 1294, 145–151 CrossRef CAS PubMed.
Footnote |
† Electronic supplementary information (ESI) available. See DOI: 10.1039/c5ra17535f |
|
This journal is © The Royal Society of Chemistry 2015 |