DOI:
10.1039/C5RA16479F
(Paper)
RSC Adv., 2015,
5, 99253-99269
Performance of hybrid nanostructured conductive cotton threads as LPG sensor at ambient temperature: preparation and analysis†
Received
15th August 2015
, Accepted 4th November 2015
First published on 5th November 2015
Abstract
The present research work explores the feasibility of a novel sensor based on nanostructured cotton threads. These threads were functionalized using carbon nanotubes (CNTs) and PAni/γ-Fe2O3 nanostructures. To begin with, we prepared catalyst nanoparticles by a hydrothermal method. These nanofabricated catalyst particles were used to grow CNTs by a newly designed catalytic chemical vapor deposition set up. The resulting CNTs were subjected to strategic purification steps. Both nanofabricated catalyst and decontaminated CNTs were analysed to study their size, shape, surface morphology and crystalline structure. Pure CNTs were successfully incorporated on permeable cotton threads using basic submersion in a CNT-ink under an ultrasound environment. These profoundly conductive cotton threads were functionalized using PAni/γ-Fe2O3 nanostructures by facile ultrasound assisted in situ polymerization. The hybrid nanostructured cotton threads were prepared at different loadings of both CNTs and PAni/γ-Fe2O3 nanocomposite and their influence was further investigated to study their surface morphology, electrical behavior and sensing performance for the detection of LPG. A quick response time and maximum response value (Rres = 0.91) were observed for low concentration (50 ppm) detection of LPG at ambient temperature. So these hybrid nanostructured cotton based sensing threads could be easily woven into the textile materials which will be useful as wearable gadgets for LPG detection in household kitchens, manufacturing sites and industries. Also this work will boost an essential encouragement for the development of wearable smart sensing gadgets.
1 Introduction
Leakages of explosive gases are a great cause of concern for environmental pollution. As liquefied petroleum gas (LPG) is a composition mix of hydrocarbons (propane and butane) and a highly inflammable gas, its air pollution affects health through irritating the respiratory tract, nose and eyes. LPG is regularly used as a cooking fuel for household purposes, vehicles, agriculture and industrial production sites and thus accidental explosion caused by leakages has sharply increased. These could be very dangerous to property and a threat to human lives. So to overcome these significant issues, it is imperative to develop fast and selective detection with precise monitoring that could detect any LPG leakage at low concentrations (ppm) and thus be beneficial in preventing the occurrence of accidental explosions, fire hazards and losses. The National Institute for Occupational Safety and Health (NIOSH) and Occupational Safety and Health Administration (OSHA) have specified lower explosive limit (LEL) standards for chemical hazards that is 21
000 ppm (2.1% by volume in air) for propane and 19
000 ppm (1.9% by volume in air) for butane. The NIOSH and OSHA have also set permissible exposure limit (PEL) standards for LPG as 1000 ppm (0.1% by volume in air). Levels below this limit can be detected if a safety device like highly sensitive and quick time LPG sensor is put in place. Reliable sensors with improved gas response have been developed to monitor and precisely measure leakages. But, at certain low permissible concentrations of LPG, some metal oxides/sulfides and ceramic based LPG sensors have shown poor performance with respect to the sensitivity, response and recovery times, long term stability, selectivity, high power consumption, complex fabrication and high cost for the detection and generally operate above room temperature. Consequently, there is a need for the development of cost effective sensors to monitor LPG of the lowest possible concentration at room temperature (∼30 °C). The detection of LPG at room temperature will definitely be helpful for households, safety of vehicles, chemical industries and research laboratories. To overcome these draw backs, conducting polymers (CPs) and nanostructures (such as nanoparticles, nanotubes, nanosheets, nanoflowers and nanowalls) have been recently investigated as effective materials for room temperature chemical sensors which have favorable properties such as easy processability and degree of miniaturization.1–20
Sensing technology21–26 has become more significant because of the various sensing methods (based on different materials and techniques) and their widespread smart wearable applications. Xiao et al.22 summarized a comparison of various sensing materials and methods, along with their performance, advantages and disadvantages.22 Potential sensing applications for various forms of nanocarbon have been demonstrated owing to their good electrical conductivity.27,28 Carbon nanotubes (CNTs) possess extraordinary and excellent characteristics29,30 such as electrical, mechanical, thermal, electronic, vibrational, optical and electromechanical properties which corroborate their potential in a variety of applications29,31,32 in flat panel displays, energy storage, field emission devices, electrochemical, electronic gadgets and sensors.30,33–40 CNTs are an intriguing nano-cylindrical form of rolled carbonaceous graphene sheet capped at both ends by a half fullerene molecule. They has also shown potential as a sensing element in various nano-electro mechanical sensors such as chemical sensors, temperature sensors, mass sensors, pressure sensors, flow sensors and biosensors. These CNT based sensors have been investigated, and their properties indicate that they can play a vital role when designing sensors.29,34–52 Hybrid nanocomposites based on chitosan grafted CNTs and graphene oxide have demonstrated their potential for vapor sensing of organic compounds53 and drug delivery,54 while CNT ink can detect hydrogen peroxide and NADH (β-nicotinamide adenine dinucleotide).41 Doped CNTs also have been utilized for nanosensors to detect CO2, CH4, CO and water molecules with good response at room temperature.42,43,55 MWCNTs grown on iron catalyst nanoparticles can be used for sensing applications.56,57 Because of the extremely sensitive perturbations and electric properties of SWCNTs, nanoscopically functionalized hybrid layer (with the transition metals Au, Ag, Co, Fe, Mn, Ni, Pd, Pt and Ti) based sensors were used to detect alcohol vapor, NH3, NO2, CO and C6H6 at room temperature.44–51 In recent years, many efforts have been put forth for metal oxide and sulfide based gas sensors such as Fe2O3,1 Bi2O3 and Y2O3,2 SnO2,2,9 TiO2,4,5,13 ZnO,7,8,14 ZnMoO4,10 CdSe,12 ZnFe2O4,17 CdS,3,16,18,58 PbS.18 Semiconducting materials like Fe2O3 nanostructures1 and nanostructured SnO2 hollow spheres2 have been used for LPG sensing at room temperature. Similarly, CPs such as polypyrrole (PPy),58 nanostructures and fibres of PAni1,3,4,6,7,10–12,14,15,26,59 are promising polymeric materials for sensing applications. PAni–CNT composites are reported as pH sensors for the detection of triethylamine, nitrite vapour, CO2, acetaminophen present in acetate, glucose, ascorbic acid, H2O2, NH3, H2S, acetic acid, hydrazine. These sensors are useful for detection in industrial monitoring, personal safety, and the medical field.37,52,60
Nanocarbon-based gas sensors bear tremendous flexibility that makes them ideal and suitable candidates, e.g. super-stretchable spring-like CNT ropes, for the next generation gas/chemical sensors.61,62 Porous cotton threads are made profoundly conductive using basic submersion of a CNT without affecting its shape because CNTs have solid van der Waals interactions with this sort of poly-D-glucose chain based micro fibrils. Permeable conductive textiles have been receiving immense interest for smart wearable devices. These wearable applications include (i) energy storage device,63 (ii) electronics and yarn based ammonia sensor,23 (iii) multi-grade nanostructured (PPy-MnO2-CNT) cotton thread base wearable supercapacitor,64 (iv) wearable electrochemical devices for detection of K+ and NH4+, pH,24 (v) cable-type polyelectrolyte-wrapped graphene/CNT core-sheath fibre based supercapacitor,65 (vi) metal-free fiber-based generator (FBG) based smart garments,66 (vii) highly sensitive, stretchable and wearable multifunctional sensors (based on conductive electrodes of silver nanowires and dielectric made from Ecoflex) for healthcare and flexible touch panel,67, (viii) triboelectric generator (TEG) for an energy harvesting device interconnected by conductive threads as wearable electronics,68 (ix) stretchable electronic devices made from CNTs for detection of human motion, including typing, body movement, speech and breathing,69 (x) CNT based elastic strain sensors and robust rotational actuators,70 (xi) cotton fiber/textile structures as wearable electronic devices with mechanical functionalities,71 (xii) smart fabric sensor as electronic textiles25 and (xiii) wearable textile-based battery rechargeable by solar energy.72 In situ polymerized PPy/cotton fabric based conducting textiles possess high conductivity, and shallow skin depth.58,73 The flexible sensors were fabricated on 100 μm thick polyimide foils which can withstand mechanical deformations. This beneficial concept can be useful for instance in safety applications for objects located in a dangerous environment to avoid direct access by hand.74
1.1 Aims of this research work
We have reviewed various fabrication techniques, properties and application of hybrid nanostructured conductive cotton threads and reported in detail in the review article of this paper. From the above aforementioned previous research perspectives and motivation (discussed in section S1 of ESI†) from past work, we have demonstrated strategic methods for the development of hybrid nanostructured conductive cotton threads which were investigated for LPG sensing at room temperature. This research work includes the preparation of Fe/MgO catalyst nanoparticles by a hydrothermal method, preparation of CNTs by a newly designed chemical vapor deposition (CVD) set up followed by their successive purification. Characterization and analysis of catalyst nanoparticles and CNTs are also discussed which determine size and shape, growth mechanism and crystalline structure. Pure CNTs were variably incorporated on the surface of a flexible cotton thread by an ultrasound sonication route. An ultrasound assisted in situ chemical polymerization route was preferred for the preparation of PAni/γ-Fe2O3 nanostructures. These polymer nanostructured composites were embedded in variable amounts on conductive threads by an ultrasound assisted dipping and coating method. Pure CNTs, PAni/γ-Fe2O3 nanostructures and CNT/PAni/γ-Fe2O3 nanostructured threads were studied for thermal stability and electrical current–voltage (I–V) characteristics. Meanwhile, the gas sensing performance such as response value, response time and recovery time, selectivity of composition, sensing mechanism were studied to assess the feasibility of hybrid nanostructured cotton threads for the detection of LPG at room temperature.
1.2 Importance of the current research work
This research reports the preparation of hybrid nanostructured cotton threads and the feasibility of using them for the detection of 50 ppm LPG at room temperature. This research work is important, because CNT and PAni/γ-Fe2O3 nanostructures were embedded on cotton threads for the first time by an ultrasound assisted dipping and coating method and in situ polymerization under an ultrasound environment. Fast recovery and quick response times have been achieved by functionalizing cotton threads using hybrid (CNT/PAni/γ-Fe2O3) nanostructures owing to their nanoscale morphology and expansive surface. These achieved results are also significant because as wt% loading of CNT increases the conductivity of the hybrid nanostructured thread improves, which is a crucial parameter for higher response value towards low concentration (50 ppm) of LPG detection at ambient temperature. A higher loading of (CNT/PAni/γ-Fe2O3) nanostructures on the surface of cotton threads showed a maximum response value (0.91) towards low concentration (50 ppm) of LPG. These hybrid nanostructured threads could be an efficient sensor for detection of LPG at room temperature. Meanwhile, it can be woven into textile garments and also be useful as wearable gadgets for LPG detection in household kitchens and manufacturing sites.
2 Experimental
2.1 Materials and reagents
Iron(III) nitrate [Fe(NO3)3·9H2O] (Rankem, RFCL Limited, New Delhi, India), MgO (Merck Specialties India Limited, Mumbai, India) and ethanol (Changshu Yangyuan Chemical Cooperation Limited, China) were received and used as such for the synthesis of catalyst nanoparticles. Carbon precursors like ferrocene and xylene (Merck Specialties India Limited, Mumbai, India) were received and used as such for synthesis of CNTs by the CVD method. Argon gas (Bhandari Carbonic, Jalgaon, India) was used as carrier and inert gas. Nitric acid (HNO3, S.D. Fine-Chem limited, Mumbai, India) was used for purification of CNTs. Sulphuric acid (H2SO4) and potassium dichromate (K2CrO7) were also received for cleansing treatment of glass slides. Aniline and ammonium persulphate (APS, Fisher Scientific, Mumbai, India) was received and used as such for the preparation of nanostructured PAni. Sodium dodecyl sulphate (SDS), hydrochloric acid (HCl) and perchloric acid (HClO4) were procured from Himedia Laboratories Private Limited (Mumbai, India), while N-methyl-2-pyrrolidone (NMP) was received from Merck Specialities India Limited (Mumbai, India). Anhydrous ferric chloride (FeCl3) and sodium hydroxide (NaOH) were purchased from RFCL Limited (Mumbai, India) and methanol was received from S.D. Fine-Chem Limited (Mumbai, India). Ultra-pure demineralised (DM) water 18 (MΩ) was prepared using a Smart2Pure system (Thermo Electron LED GmbH, Germany) and used for the preparation of various solutions and washing purposes. Cotton thread, Grifin 40 (Madura Coats Private Limited, Bangalore, India) was used as a stretchable material to prepare conductive cotton threads. Commercial LPG gas (for cooking purpose) was used to study the sensing performance of multigrade hybrid nanostructured conductive threads.
2.2 Synthesis of catalyst nanoparticles
Catalyst nanoparticles of Fe/MgO were synthesized in an autoclave reactor by the hydrothermal method which resulted in nanoscale particles with high specific surface area and active sites. These nanofabricated catalysts were used to synthesize CNTs with good % yield. Details of the steps involved for the preparation of Fe/MgO catalyst nanoparticles (K1, K2 and K3) are discussed in section S2 of ESI,† while Fig. S1† shows its schematic. Table 1 shows the composition of reactants taken for the synthesis of Fe/MgO catalyst nanoparticles.
Table 1 Composition of reactants used for preparation of catalyst nanoparticle
Sample code |
Weight ratio (Fe : MgO) |
Percent weight ratio (%) |
Quantity of Fe(NO3)3·9H2O (g) |
Quantity of MgO (g) |
K1 |
1 : 10 |
0.1 |
4.04 |
4.04 |
K2 |
1.25 : 10 |
0.125 |
5.05 |
K3 |
1.5 : 10 |
0.15 |
6.061 |
2.3 Synthesis and purification of CNTs
CNTs were synthesized using a CVD reactor setup which was designed and supplied by Lelesil Innovative System (Mumbai, India). Construction, design and working of the CVD reactor setup are discussed in section S3 of ESI.† Fig. S2† illustrates the detailed design of the CVD set up, while Table S1(a–c)† shows its dimensions. The details of the steps for the synthesis of CNTs by the CVD method are discussed in section S4 of ESI.† Fig. S3(a)† illustrates the arrangement of the CVD reactor set up, while Fig. S3(b)† shows a graphical presentation of the steps involved in the synthesis of CNTs by the CVD method using carbon precursors. The graphical steps indicate that preheating and cooling periods were kept at 20 min, while vapor deposition/residential time was kept at 20–40 min during which CNT growth took place at a temperature range between 800–1000 °C. The resulting materials were soft and spongy in nature with a carbonaceous texture indicating the vicinity of CNTs.
The purification of CNTs was achieved by a number of strategic steps (in section S5 of ESI†), which involves extraction of impurities, oxidation and acid treatment, separation and drying as shown in Fig. 1. The method was comprised of (i) extraction of soluble impurities using solvent (e.g. toluene), (ii) liquid-phase oxidation of amorphous carbon, (iii) acid treatment for removal of unprotected metallic catalyst particles, (iv) ultracentrifugation step for separation of the CNTs from the unwanted graphitic impurities and protected catalysts and (v) drying to obtain powdered material. This intriguing method is exceptionally useful because it allows for the selective extraction of impurities from CNT samples.
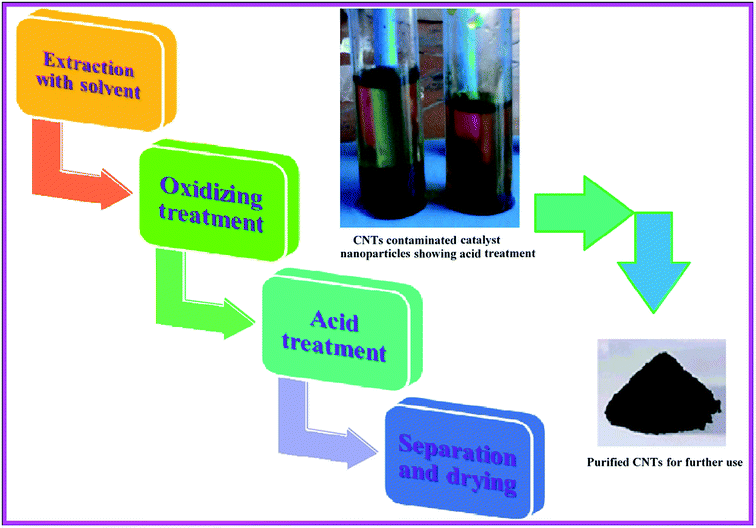 |
| Fig. 1 Illustration of the multi-step strategy for the purification of CNTs. | |
2.4 Synthesis of PAni/γ-Fe2O3 nanostructures
The synthesis of γ-Fe2O3 nanoparticles was carried out as per the earlier approach1 of ultrasound cavitation steps as shown in Fig. 2(a). PAni/γ-Fe2O3 nanostructures were also prepared by the previous approach1 of in situ chemical polymerization as shown in Fig. 2(b). Microscope glass slides (Polar India Corporation, Mumbai) were used for observation of PAni/γ-Fe2O3 nanostructures. These glass slides were cleaned by keeping them in chromic acid (H2CrO4, mixture of H2SO4 and K2CrO7) solution for 30 min. Finally, these clean slides were immersed completely in 0.1 M HCl solution for 60 min. The slides were then progressively sonicated in water and methanol for 15 min each, followed by vacuum drying prior to use for analysis of PAni/γ-Fe2O3 nanostructures.
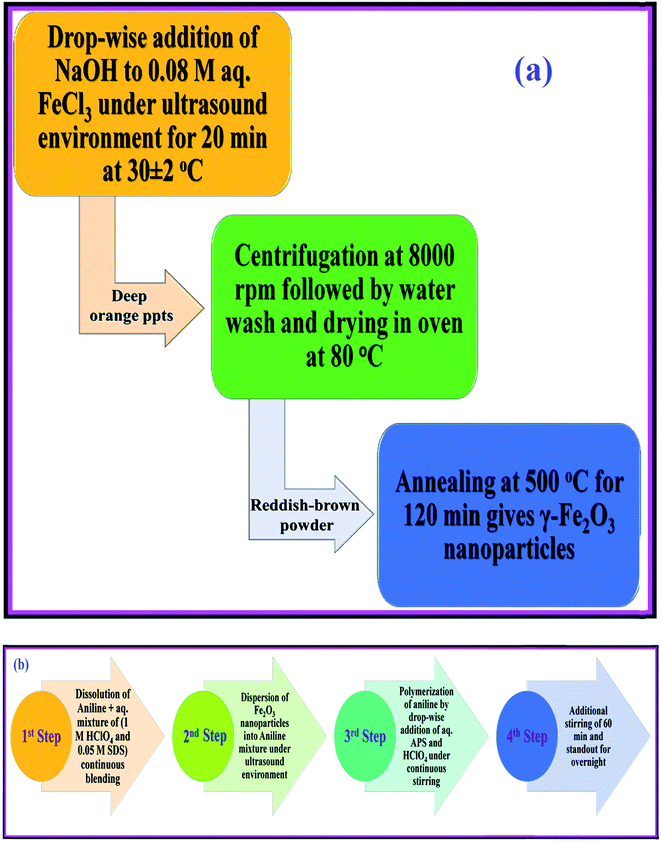 |
| Fig. 2 Steps for the preparation of (a) γ-Fe2O3 nanoparticles and (b) PANi/γ-Fe2O3 composite films. | |
2.5 Preparation of hybrid nanostructured cotton threads
2.5.1 Cleaning treatment and functionalization of the cotton threads using CNT ink. A commercial white cotton thread was used to prepare nanostructured sensing threads. At first, threads were washed with hot water. Since the surfaces of the threads are fibrous, they were also treated with ethanol which eliminated the redundant fibres. Threads were also cleaned three times by acetone and DM water. These clean threads were immersed into 5 M HNO3 solution for 60 min to increase the hydrophilicity. Finally, the threads were rinsed with DM water under ultrasound conditions for 15 min and dried in an oven for 15 min to remove water traces. Pure CNTs were uniformly incorporated onto the surface of the cotton thread using a well-dispersed CNT colloidal solution. First, CNTs were treated with 5 M HNO3 for 30 min and then with 40 mg mL−1 of SDS, and were finally dispersed in DM water. Last, the colloidal mixture of CNT was sonicated for 20 min before functionalizing them on the surface of cotton threads. The pure cotton threads were alternately dipped into the colloidal solution of CNT ink. Finally, the mixture was sonicated for 20 min. Due to the hierarchical and permeable structure of the cotton thread, it quickly swells the large amounts of the CNT from colloidal solution.23,25,61–64,71,72 The conductive cotton threads were dried in an oven at 80 °C for 120 min.
2.5.2 Incorporation of PAni/γ-Fe2O3 nanostructures on conductive threads using strategic protocol. Conductive (CNTs functionalized) cotton threads were immersed in a solution of in situ polymerized PAni/γ-Fe2O3 nanostructures and then uniformly mixed for 15 min under an ultrasound environment. Then it was allowed to stir continuously for 12 h and left to stand overnight. These hybrid nanostructured conductive cotton threads were dried in an oven at 80 °C for 2 h. Table 2 shows the quantity and size of materials and reactants taken during the preparation of hybrid nanostructured cotton threads. Before being used for the first time, each thread sample was dipped in solution and treated for 15 min under ultrasound conditions for uniform distribution of multigraded nanostructures. Subsequently their reinforcement on each cotton thread was carried out successfully under ultrasound conditions.
Table 2 Composition of reactants used for the preparation of hybrid (CNT/PAni/γ-Fe2O3) nanostructured threads
Sr. no. |
Sample code |
Quantity/scale of material |
CNT (mg) |
PAni/γ-Fe2O3 nanostructures (mg) |
Thread (cm) |
1 |
CNT pellet |
100 |
— |
— |
2 |
PANi/γ-Fe2O3 pellet |
— |
100 |
— |
3 |
CNT/thread |
50 |
— |
5 |
4 |
PANi/γ-Fe2O3/thread |
— |
50 |
5 |
5 |
PANi/γ-Fe2O3/CNT/cotton thread (hybrid nanostructured thread) |
25 |
75 |
5 |
6 |
50 |
75 |
5 |
7 |
75 |
75 |
5 |
8 |
100 |
75 |
5 |
9 |
100 |
100 |
5 |
10 |
100 |
125 |
5 |
11 |
100 |
150 |
5 |
2.6 Characterization and measurement techniques
2.6.1 X-ray diffraction (XRD). XRD analysis of nanofabricated catalyst particles, CNTs, γ-Fe2O3 nanoparticles and PAni/γ-Fe2O3 nanostructured composite was conducted on an Advance X-ray diffractometer, (D8, Brukers Germany) with CuKα1 radiation (λ = 1.5404 Å) within the 2θ range of 20–80°.
2.6.2 Field emission-scanning electron microscopy (FE-SEM). An FE-SEM (S-4800, Hitachi, Tokyo, Japan) microscope was used to study the growth mechanism of CNTs, size and shape of γ-Fe2O3 nanoparticles and surface morphology of PAni/γ-Fe2O3 nanostructured composites and multigraded nanostructured threads. The CNTs were dispersed in acetone and subjected to ultrasound treatment for 10 min to obtain a holey carbon film before viewing under the microscope. Hybrid nanostructured cotton threads were gold coated and mounted on a specimen stub prior to being viewed in the microscope.
2.6.3 Tunneling electron microscopy (TEM). The exact size and shape of MWCNTs were studied by a CM200, TEM (Philips, Netherlands) at a resolution of 2.4 Å and operating voltage of 20–200 kV. Pure MWCNTs were dispersed in water and kept in a conventional ultrasonic bath for 10 min. A sonicated crude droplet of colloidal suspension was dried and then put on a holey electron micro copper grid as a microscope specimen.
2.6.4 Current–voltage (I–V) measurements by four probe conductivity meter. The current–voltage (I–V) characteristic values of nanostructured threads were measured by a four-point probe conductivity meter, using a current source (CCS-01) and micro voltmeter (DMV-001, SES Instruments, Scientific Equipments, Roorkee, India). Each sample of nanostructured thread was placed in contact with four-probes and variation in the electrical current was studied. The voltage was applied, at the same time the current was recorded in the bias range of 2 to −2 V by a multi meter using four probes. All the electrical measurements were carried out at an ambient temperature 30 ± 2 °C. The (I–V) characteristic values of each thread sample were recorded in the presence of air at ambient temperature. The conductive/ohmic nature of the nanostructured thread was studied from the (I–V) characteristic graphs.
2.6.5 Gas sensing procedure and characteristic properties. Fig. 3 shows a schematic representation of the two probe gas sensing setup, while the actual arrangement of laboratory set up is shown in Fig. S4.† Construction details of the set up are described in section S6 of the ESI.† Gas sensing experiments were carried out in a sealed quartz glass test chamber (Vijay Scientifics, Aurangabad, India) of 1.5 L volume as shown in Fig. S4.† The samples of hybrid nanostructured cotton threads were attached on a glass substrate and both ends were contacted between two conducting probes. At first, the chamber was evacuated and purged continuously with pure argon gas. Then, 50 ppm of commercial grade (household purpose) LPG gas was injected using a syringe into the glass chamber. Both ends of the nanostructured cotton threads were contacted with the help of the two probes connected to a programmable 4.5 digital multimeter (SM 5015, Scientific MES-Technik Private Limited, Indore, India). This multimeter was attached to a computer (QT035AV, Hewlett Packard, Banglore, India) using a RS232C interface that was fully automated and logged by a program. The (I–V) characteristic values were recorded in the bias range of 0.15 to −0.15 V at intervals of 1 s. The change in current was deduced from the (I–V) characteristic plot; and the gas response value was calculated from the change in current and current measured in the presence of air. The response of the sensor was calculated from the (I–V) characteristics. All the (I–V) characteristic values were measured at ambient temperature (30 ± 2 °C) in the presence of air as well as 50 ppm of LPG. Eqn (1) shows the gas response (Rresp) value which is defined as the ratio of change in current to the current of the sensing thread measured in the vicinity of air. |
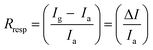 | (1) |
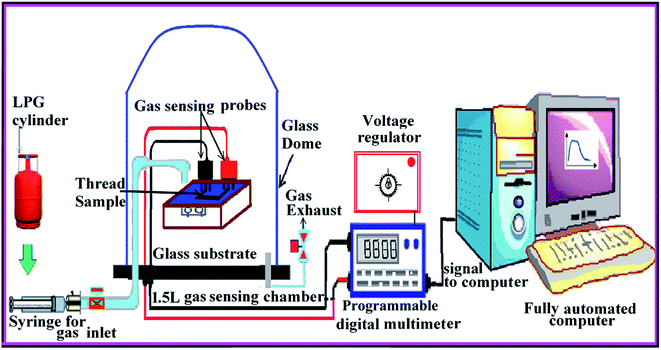 |
| Fig. 3 Schematic representation of a two-probe gas sensing setup. | |
In eqn (1), Ig is the current of the sensing thread measured on exposure to LPG gas, while Ia is the current of the sensing thread measured in the presence of air, and ΔI is the change in current. The response time (tresp) is defined as the time needed for a sensor to attain a 90% of change in current on exposure to the test gas, while the recovery time (treco) is defined as the time taken by the sample to get back to 90% of the original current in the vicinity of the air. The selectivity is defined as the ability of an optimum value of nanostructures in the sensing threads to respond to a certain ppm of gas, while in earlier work selectivity is defined with respect to a particular gas.9,14 The response and recovery time periods of the junction were determined by holding the junction at a potential range (0.15 to −0.15 V) and recording the current with respect to time.3,4
3 Results and discussions
3.1 Crystallinity studies
3.1.1 Crystalline behavior of catalyst nanoparticles. XRD patterns of the nanofabricated Fe/MgO catalyst particles are shown in Fig. 4. Nanoparticles of Fe/MgO catalyst exhibit characteristic peaks of MgO support and oxides of Fe metal. The crystal plane for MgO was observed at (2 2 0), which is at a distance from the main peak of Fe (1 0 0) in Fig. 4. The peak observed at 2θ = 43° was assigned to the Fe (1 0 0) plane, while the peak at 2θ = 67° was allocated to MgO (2 2 0).
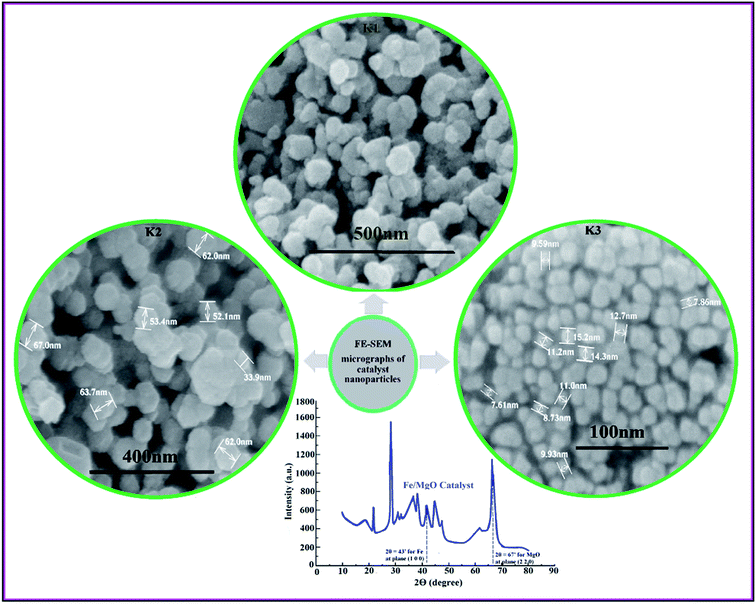 |
| Fig. 4 FE-SEM micrographs [K1 (1 wt%), K2 (1.25 wt%), K3 (1.5 wt%)] and XRD pattern of catalyst (Fe/MgO) nanoparticles. | |
3.1.2 Crystalline nature of CNTs. Fig. 5 also shows X-ray diffractograms of the CNTs grown over the catalyst K1, K2, and K3. The XRD pattern clearly shows that the CNTs were pure and well graphitized. The entire XRD pattern of the CNTs shows four major peaks at 2θ = 26°, 44°, 56° and 62° for all catalysts obtained from Fe/MgO. Clear reflections similar to those of graphite are observed above the primary peaks (0 0 2), (1 0 0), (0 0 4) and (1 1 0) in case of all CNT samples. The peak at 2θ = 26° confirms the presence of more crystalline graphitic carbon in the CNTs synthesized by catalysts K1 and K3. It is observed that the peak for catalyst K2 is slightly visible at 2θ = 26°. Table 3 shows yield and crystallinity of CNTs synthesized using different catalysts K1, K2 and K3. When CNTs were synthesized using catalyst K2, greater crystallinity was observed relative to those synthesized using catalyst K1 and K3 owing to their different electronic structure.
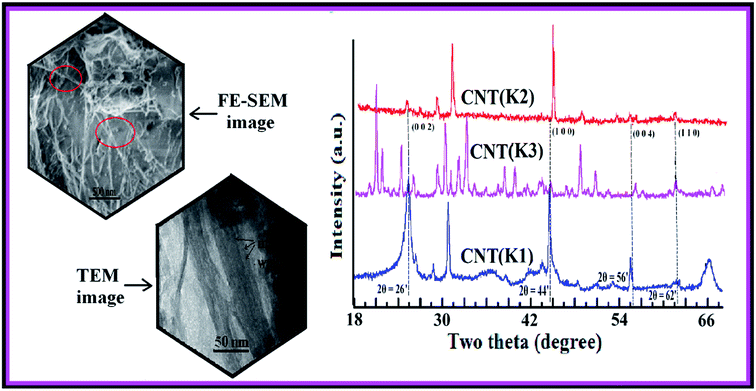 |
| Fig. 5 FE-SEM micrographs of CNTs showing growth at 1000 °C and XRD pattern of CNTs showing crystalline behavior. | |
Table 3 Yield and crystallinity of CNTs
Sample code |
Catalyst code |
Weight of catalyst (g) |
Weight of product + catalyst (g) |
Weight of pure CNT purified (g) |
% of crystallinity (from XRD) |
CNT1 |
K1 |
0.2 |
0.361 |
0.310 |
63.7 |
CNT2 |
K2 |
0.451 |
0.394 |
76.8 |
CNT3 |
K3 |
0.396 |
0.352 |
63.6 |
3.1.3 Crystal structure of γ-Fe2O3 nanoparticles and PAni/γ-Fe2O3 nanocomposites. X-ray diffractograms of PANi and its nanocomposites were studied earlier.1 From observed peaks and d-values in the range of 0.12–0.30 nm, it is confirmed that the γ-Fe2O3 nanoparticles were cubic.1 The crystallite size of these γ-Fe2O3 nanoparticles was calculated by Scherrer’s equation, and was found to be 29.5 nm, while the crystallinity was 55%. The crystallinity of PAni/γ-Fe2O3 (3 wt%) nanostructures was reported to be 63%.1
3.2 Surface morphology
3.2.1 Shape and size of catalyst nanoparticles. Fig. 4 shows FE-SEM micrographs indicating size, shape and definite crystalline morphology of nanofabricated catalyst particles. It can be seen from Fig. 4 that the catalyst particles possess nano-spherical shape with a smooth surface. The size of the K1 catalyst nanoparticle was reported to be 33–67 nm and size of the K3 catalyst nanoparticle was observed to be small of 5.5–14 nm. Uniform particles size was observed in the case of K2 catalyst nanoparticles, which also shows an increment in % yield of CNT produced.
3.2.2 Growth and morphology of CNTs. The growth of CNTs was observed at different temperatures ranging from 500 to 1000 °C, which is shown in Fig. S5(a–e)† and discussed in section S7 of the ESI.† The TEM and FE-SEM cross-section micrographs in Fig. 5(a) show the growth of MWCNTs at 900 °C using K2 catalyst after a chemical vapor deposition time of 20 min. Fig. 5(a) demonstrates that MWCNTs having small diameters might exist at 1000 °C or even SWCNTs. In general, CNTs grow on catalyst nanoparticles and might have an almost smaller or similar diameter to catalyst nanoparticles. These results are in close agreement with the consequences of XRD patterns which are demonstrated and discussed in an earlier section. The TEM micrograph in Fig. 5 shows the multiwall of CNTs. The TEM study reveals that most of the multi-layered CNTs have diameters in the range 30–5 nm. Hence the MWCNTs are crystalline in nature with the least defects due to the effect of ultrasonication and centrifugation steps during purification.28
3.2.3 Nanostructures of PAni/γ-Fe2O3 composites. Fig. 6(a) and (b) elaborate the FE-SEM micrographs of γ-Fe2O3 nanoparticles and PAni/γ-Fe2O3 (3 wt%) nanocomposites. Uniform nanofibers of PAni/γ-Fe2O3 (indicated by blue arrows) with a diameter of ∼72 nm are observed in Fig. 6(a). This shows the adequacy of ultrasound in producing non-aggregated nanofibres in the absence of an encapsulating agent. Fig. 6(b) shows the uniform dispersion of γ-Fe2O3 nanospheres (indicated by white circles) in the PAni matrix. Nanofibrillar morphology of PAni having a diameter of about 70–74 nm can be clearly seen. It appears that increasing the amount of γ-Fe2O3 nanoparticles can result1 in a larger diameter of PAni nanofibers. From the FE-SEM results shown in Fig. 6(b), it is evident that ultrasound induced cavitation can produce non-aggregated, nanostructured γ-Fe2O3 particles, and also it can aid uniform dispersion of the nanoparticles into the polymer matrix.
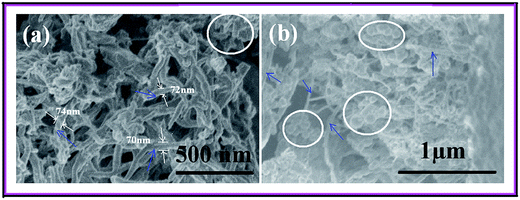 |
| Fig. 6 Nano-fibrous morphology of PAni/γ-Fe2O3 composite films. | |
3.2.4 Hybrid nanostructured surface of conductive cotton threads. Fig. 7 shows schematic, graphical and actual FE-SEM views of hybrid nanostructured conductive threads. The schematic view in Fig. 7 indicates that CNTs were successfully incorporated into individual micro fibrils of cotton threads. The graphical view of the Fig. 7 demonstrates that electrical conductivity increases as mass loading of CNTs increases. The amount of CNT coating can be easily controlled by changing the concentration of CNT ink and dipping time. Considering mechanical flexibility and strength for the conductive threads, it is not preferred to increase the mass loading of CNTs.64
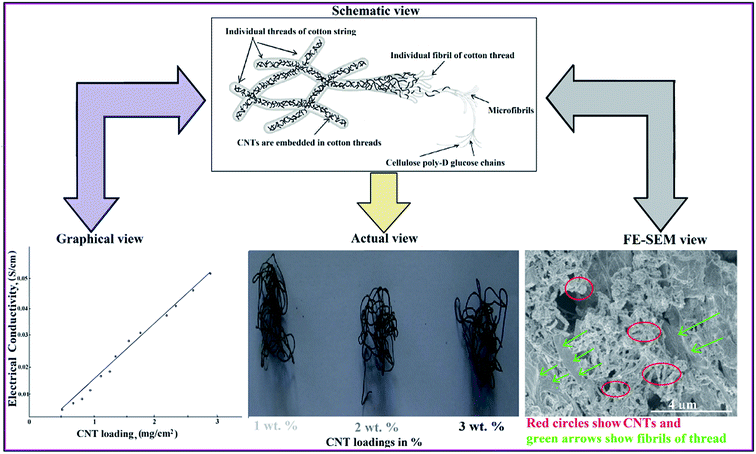 |
| Fig. 7 Schematic view [Reprinted with permission from ref. 63. Copyright (2010) American Chemical Society], graphical view, photographic view and FE-SEM view of conductive cotton thread functionalized using CNTs. | |
The genuine photographic FE-SEM view in Fig. 7 shows flexible cotton threads coated with various loading of CNTs. The incorporated CNT/PAni/γ-Fe2O3 (3 wt%) hybrid nanostructures (red circles) can be visualized on the surface of cotton threads (green arrows) as shown in the FE-SEM view of Fig. 7.
Fig. 8(a)–(f) shows FE-SEM micrographs indicating the presence of PAni/γ-Fe2O3 nanostructures on the conductive cotton threads. Due to the ultrasound effect, the PAni/γ-Fe2O3 nanostructures get uniformly distributed over the surface of the conductive threads without affecting its diameter and length. It can be said that when the amount of γ-Fe2O3 nanoparticles was increased, embedment of PAni/γ-Fe2O3 nanostructures resulted in a larger diameter. The blue coloured circle area shows the vicinity of PAni/γ-Fe2O3 nanostructures on the surface of conductive threads and it was also reported that increasing the amount of PAni/γ-Fe2O3 nanostructures can lead to effective sensing and response.1 The LPG sensing response of the hybrid nanostructured conductive thread can be increased by increasing the amount both of CNTs as well as PAni/γ-Fe2O3 nanostructures. The large surface area of γ-Fe2O3 nanoparticles can adequately provide greater adsorption sites for detection of gas molecules which may enhance the response at higher γ-Fe2O3 nanoparticle content. On the other hand an increase in loading of CNTs provides high conductivity and strength to the cotton thread. Fig. 9(a) and (b) clearly show the loading of PAni/γ-Fe2O3 (3 wt%) nanostructures incorporated at different wt%. Green circles show evidence of CNTs functionalized on cotton threads (blue arrows) shown in Fig. 9(c).
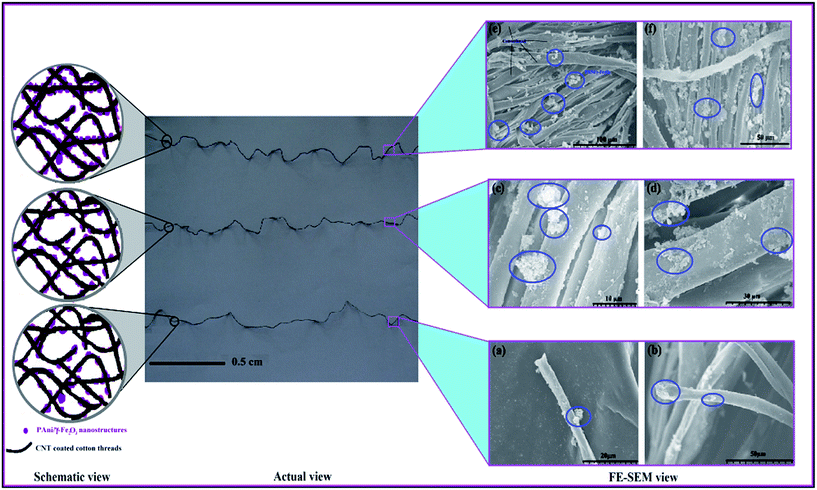 |
| Fig. 8 FE-SEM micrographs of multi-grade nanostructured thread [(a and b) 1 wt%, (c and d) 2 wt% and (e and f) 3 wt% loading of PAni/γ-Fe2O3 nanostructures]. | |
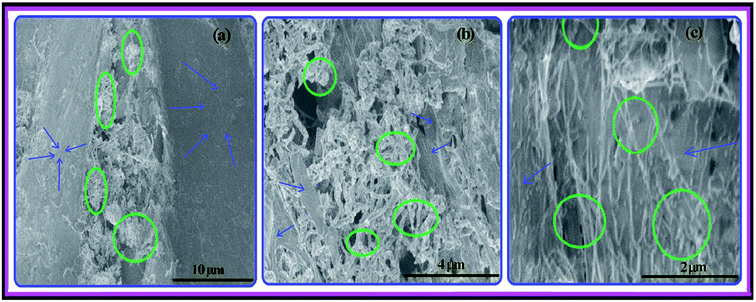 |
| Fig. 9 FE-SEM micrograph of conductive thread embedded with 3 wt% PAni/γ-Fe2O3 nanostructures [(a) 3 wt%, (b) 5 wt% and (c) 10 wt% loading of CNT]. | |
3.3 Electrical (I–V) characteristics of hybrid nanostructured cotton threads
Fig. 10(a)–(c) illustrate (I–V) characteristics of conductive cotton threads functionalized by CNTs as well as PAni/γ-Fe2O3 nanostructures. Earlier, the semi conducting nature of PAni/γ-Fe2O3 nanocomposites was reported from the non-linear nature of (I–V) characteristics.1 Fig. 10(a) shows (I–V) characteristics of pure thread, Fig. 10(b) shows the effect of various CNT loadings and Fig. 10(c) shows the effect of various loadings of PAni/γ-Fe2O3 nanostructure on (I–V) characteristics. It is clear from all symmetrical (I–V) characteristics that the electrical contacts on the films show ohmic nature. CNTs exhibit higher electrical conductivity than that of PAni/γ-Fe2O3 nanostructures as shown in Fig. 10(a) and here both show a linear nature. A higher electrical conductivity of the thread was observed on increasing the amount of CNT nanostructures and can be confirmed from Fig. 10(b), while PAni/γ-Fe2O3 nanostructure loadings may decrease the electrical conductivity and this can be confirmed from the nanostructures shown in Fig. 10(c). Meanwhile, Fig. 10(b) and (c) shows the current–voltage (I–V) characteristics of hybrid nanostructured threads.
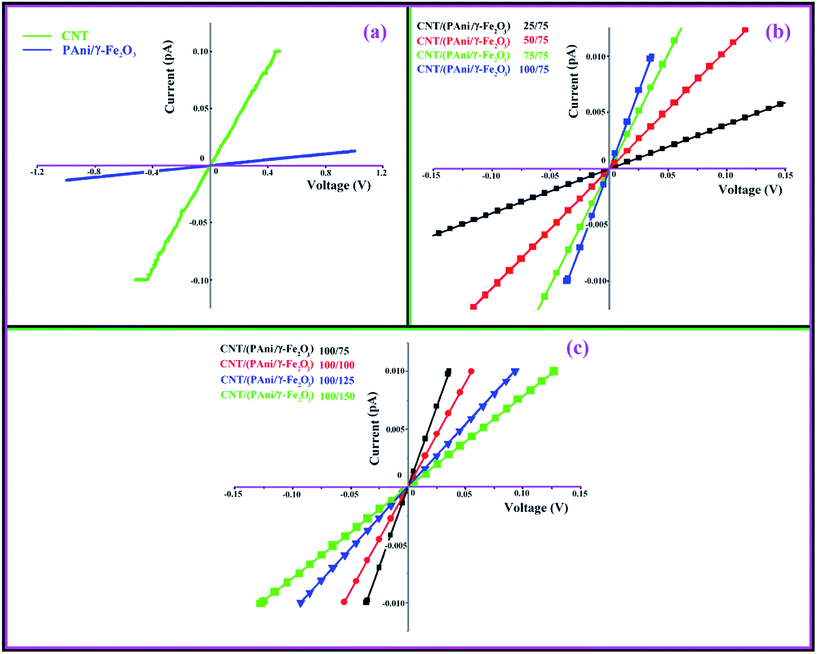 |
| Fig. 10 Current–voltage (I–V) characteristics of multi-grade nanostructured thread (a) virgin samples, (b) variation in CNT loading (c) variation in PAni/γ-Fe2O3 nanostructure loading. | |
3.4 Gas sensing performance of hybrid nanostructured cotton threads
3.4.1 Gas sensing characteristics. Fig. 11(a)–(e) illustrates gas sensing characteristics of hybrid nanostructured threads which can detect 50 ppm LPG at ambient temperature. The conducting nature of the PAni/γ-Fe2O3 nanocomposite was confirmed because of adsorption of LPG molecules. As shown in Fig. 11(a), no significant change in electrical resistance was observed when the conductive thread was exposed to LPG, i.e. conductive threads do not render the sensing response value to LPG as compared to PAni/γ-Fe2O3 nanostructures. PAni/γ-Fe2O3 nanostructures showed a significant reduction in current due to the adsorption of LPG molecules over their surface and also PAni/γ-Fe2O3 nanocomposites were semiconducting in nature.1 However, with incorporation of γ-Fe2O3 nanoparticles can pronounce the increment in resistance of PAni/γ-Fe2O3 nanocomposite when exposed to a low concentration (50 ppm) of LPG. This increment in resistance on exposure to LPG becomes more significant with increasing content of γ-Fe2O3 nanoparticles.1
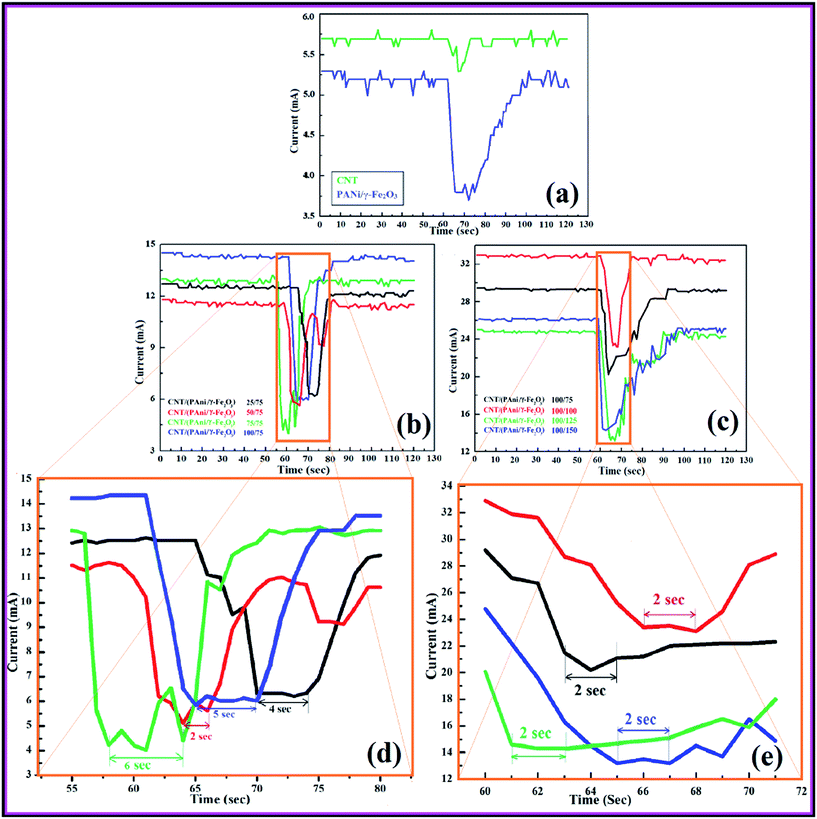 |
| Fig. 11 Current–time characteristics of (a) virgin samples, multi-grade nanostructured thread, (b) loaded with various wt% of CNT at constant wt% of PAni/γ-Fe2O3 nanostructures, (c) loaded with various wt% of PAni/γ-Fe2O3 nanostructures at constant wt% of CNT, (d) magnification of image (b) showing response time of multi-grade nanostructured threads loaded with various wt% of CNT, (e) magnification of image (c) showing response time of multi-grade nanostructured threads loaded with various wt% of PAni/γ-Fe2O3 nanostructures. | |
3.4.2 Selectivity of composition. Fig. 11(b) and (c) depict the current–time characteristics indicating the variation in loading of CNTs and PAni/γ-Fe2O3 nanostructures over the surface of cotton threads. Fig. 11(b) shows current–time characteristics of sensing threads incorporated with various loadings of CNTs by keeping constant loading of PAni/γ-Fe2O3 nanostructures. Fig. 11(b) also shows that selective composition of CNT/(PAni/γ-Fe2O3) nanostructure loading was 75/75. Similarly Fig. 11(c) shows current–time characteristics of the hybrid nanostructured thread incorporated with various loadings of PAni/γ-Fe2O3 nanostructures by keeping constant loading of CNTs. The selective and optimum composition ratio of CNT/(PAni/γ-Fe2O3) nanostructures loading was 100/125. Optimum and selective loading of CNTs shows a better sensing response towards LPG detection owing to the higher loading of CNTs, superior conductivity of CNTs and higher loading of PAni/γ-Fe2O3 nanostructures. It is clear from the characteristics that both optimum and selective composition ratio of CNT/(PAni/γ-Fe2O3) nanostructures at 100/125 was a selective ratio for hybrid nanostructured sensing threads for detection of 50 ppm LPG.
3.4.3 Response and recovery times. A very short, i.e., quick response time (tresp) of hybrid nanostructured conductive threads should be understood in the framework of rapid gas diffusion over the sensing surface due to the nano porous shell structures. This obviously confirms that the multigrade hybrid CNT/(PAni/γ-Fe2O3) nanostructures are promising materials for the development of a highly sensitive and fast responding gas sensor. As shown in Fig. 11(d) and (e), the response time (tresp) of hybrid nanostructured sensing threads was observed to be ∼20–25 s and the minimum recuperation/recovery time (treco) was observed to be ∼35–40 s. It can be said that hybrid nanostructured sensing threads showed quick response and fast recovery time, which may be due to its crystalline nature, nanoscale morphology, high LPG adsorption capability on the expansive surface of γ-Fe2O3 activator molecules and therefore fast reduction of exposed gas.
3.4.5 LPG sensing phenomena and reaction mechanism. The LPG sensing mechanism of the sensor is a surface controlled phenomenon i.e., it is based on the surface area of the nanostructured materials. Initially, oxygen from the atmosphere gets adsorbed on the surface of the film and picks up electrons from its conduction band. The sensitivity of a sensor is mainly determined by the interactions between the gases and the surface of a sensor. The enhanced sensitivity of the hybrid nanostructured thread based sensor may be attributed to its surface related factors (1D surface morphological structure); such as high surface area, a large space charge layer change and small crystallite size. The specific surface area of nanocrystallites is larger. Further, the small crystallites of γ-Fe2O3 provide more adsorption–desorption sites, as a result of the increased amounts of surface atoms and grain boundaries, which will also give a contribution to increment in the sensitivity of the sensor.1,17 It was already demonstrated in previous work1 that the response value was 0.5 for detection of 50 ppm LPG and it was 1.3 for detection of 200 ppm LPG. In this research work, the gas response value of hybrid nanostructured is reported to be 0.91 for detecting the low concentration (50 ppm) of LPG. Therefore, a larger number of LPG molecules could be adsorbed so a higher gas response is possible.In fact, during adsorption of gas molecules on the surface of a thread, a potential barrier to charge transport is developed. This may significantly increase the concentration of electrons due to the sensing phenomena, which promotes an increment in the sensor’s response. Also, the adsorption of gas molecules depends on the distribution of hybrid nanostructures over the surface of threads. The LPG sensing response of the multigrade nanostructured thread is restricted by the speed of the chemical reaction because the gas molecules have enough thermal energy to react with the surface (Fig. 13). The high sensing of LPG molecules may be due to the nanospherical γ-Fe2O3 particles being uniformly distributed on the surface of the CNT functionalized conductive thread. Adsorption of LPG molecules plays a vital role in the electrical and sensing properties of the hybrid nanostructured thread. An adsorption of LPG molecules removes the conduction electrons and enhances the resistance of thread sensor. The LPG molecule is conceivably converted at the iron oxide surface (in presence of adsorbed oxygen ions) by a dehydration reaction2 as shown below.
CnH2n+2 + 2O− → H2O + CnH2n:O + e− (dehydration) |
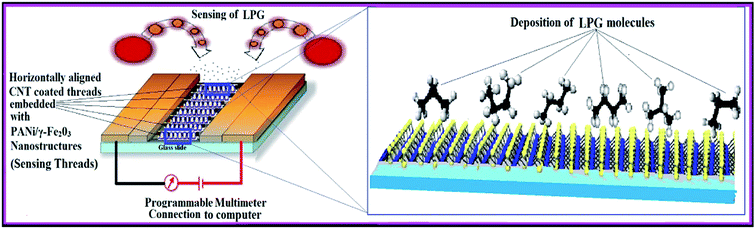 |
| Fig. 13 LPG sensing phenomena. | |
Here, LPG (CnH2n+2) represents CH4, C3H8, C4H10, while CnH2n:O represents molecules which are partially oxidized intermediates on the surface of γ-Fe2O3 nanospheres. Thus, during the oxidizing phenomena, the LPG molecule generally liberates electrons into the conduction band. This addition of electrons into the conduction band of the sensing surface on threads is a result of decreases in resistance of the sensor.13,19 As was discussed earlier1 PAni/γ-Fe2O3 nanostructures show a high sensing response to LPG gas due to the presence of the adsorption centre of Fe(III). Moreover, high dispersity of PAni/γ-Fe2O3 nanostructures on the conductive threads provides efficient electron exchange between the cations: Fe(III) ↔ Fe(II). All this produces a more prominent conductivity drop of the active layer and consequently enhances the sensor performance.
4 Conclusions
In summary, flexible conductive cotton threads were used as a platform to prepare a cable-type hybrid nanostructured sensor using CNTs and PAni/γ-Fe2O3 multi-grade nanostructures via a facile strategic synthesis under an ultrasound environment. The study shows that CNTs were optimally grown on Fe/MgO nanoparticles (10–60 nm) by the CVD method at 900 °C and purified using a combination of several chemical methods. On the other hand, sonochemically synthesized γ-Fe2O3 nanoparticles (3 wt%) were decorated and found to be disseminated uniformly into the in situ polymerized nanofibrous PAni matrix (60–75 nm) due to effect of ultrasound. These hybrid nanostructures were successfully incorporated over the surface of the conductive cotton threads which were then investigated for LPG detection at ambient temperature. The response values were found to be increased with increasing amount of CNTs. The large surface area of γ-Fe2O3 nanoparticle provides greater adsorption sites for the gas molecules, which resulted in a high sensing response value. The interaction between the gas molecules and the hybrid nanostructures induces a potential causing an increase in the depletion area of the PAni/γ-Fe2O3 nanostructures. As a result, the resistance of the hybrid nanostructured thread increases upon exposure to LPG molecules. The nanoscale morphology of hybrid conductive threads gives an expansive surface for adsorption of gas molecules which enhances the gas response value. The high response values of the multigrade hybrid nanostructured thread may be due to the combined effects of (i) low Fe–O bonding energy and smaller electro negativity, (ii) the presence of nanostructured γ-Fe2O3 spheres and (iii) the high adsorption capability of gas.
These cable type flexible sensors of multi-grade dynamic nanostructures of CNT/PAni/γ-Fe2O3 functionalized threads can be useful in the development of wearable sensing device at an ambient temperature. Here, a uniquely simple yet remarkably functional solution is offered for the development of wearable smart textiles, which considers many parameters which exceed current technological solutions, including those using carbon materials. One of the most attractive features of this approach is the simple steps involved in both the preparation and operation of the sensor. Furthermore, since a commodity such as cotton is used as a substrate and CNTs are used in a very low amount, mass production of disposable wearable sensors should be conceivable. From the technological point of view, sensors could be integrated in connection with wearable data-reading devices owing to their conductive nature. The as-made hybrid nanostructured cotton threads are flexible and robust enough to be intertwined, knotted and woven in a cloth making a smart garment possible. And this highly bendable cloth based sensor can be woven from cm-long coaxial fibres, promising application in the next generation of smart, safe and flexible garment devices or intelligent sensors.
Acknowledgements
Authors are thankful to Council of Scientific and Industrial Research (CSIR), New Delhi, India (Project no. 02(0023)/11/EMR-II) for providing financial assistance to carry out the major research project. Authors are also thankful to Dr Tanushree Sen for providing samples of polymer nanocomposites and necessary guidance. The authors (D. P. Hansora and R. Yadav) have contributed equally as first authors in this manuscript.
References
- T. Sen, N. G. Shimpi, S. Mishra and R. Sharma, Sens. Actuators, B, 2014, 190, 120–126, DOI:10.1016/j.snb.2013.07.091.
- L. A. Patil, M. D. Shinde, A. R. Bari, V. V. Deo, D. M. Patil and M. P. Kaushik, Sens. Actuators, B, 2011, 155, 174–182, DOI:10.1016/j.snb.2010.11.043.
- D. S. Dhawale, D. P. Dubal, V. S. Jamadade, R. R. Salunkhe, S. S. Joshi and C. D. Lokhande, Sens. Actuators, B, 2010, 145, 205–210, DOI:10.1016/j.snb.2009.11.063.
- D. S. Dhawale, R. R. Salunkhe, U. M. Patil, K. V. Gurav, A. M. More and C. D. Lokhande, Sens. Actuators, B, 2008, 134, 988–992, DOI:10.1016/j.snb.2008.07.003.
- D. S. Dhawale, R. R. Salunkhe, V. J. Fulari, M. C. Rath, S. N. Sawantc and C. D. Lokhande, Sens. Actuators, B, 2009, 141, 58–64, DOI:10.1016/j.snb.2009.06.025.
- K. R. Nemade and S. A. Waghuley, J. Mater. Sci. Eng. B, 2013, 3(5), 310–313 CAS . http://www.davidpublishing.com/davidpublishing/Upfile/8/19/2013/2013081906899848.pdf.
- S. B. Kulkarni, B. B. Raje Shaikh, C. D. Lokhande and S. S. Joshi, J. Shivaji Univ.: Sci., 2014, 41(2), 1–2 Search PubMed . ISSN-Science-0250-5347.
- S. Sarkar and D. Basak, Sens. Actuators, B, 2013, 176, 374–378, DOI:10.1016/j.snb.2012.10.095.
- S. S. Barkade, D. V. Pinjari, U. T. Nakate, A. K. Singh, P. R. Gogate, J. B. Naik, S. H. Sonawane and A. B. Pandit, Chem. Eng. Process., 2013, 74, 115–123, DOI:10.1016/j.cep.2013.09.005.
- B. A. Bhanvase, N. S. Darda, N. C. Veerkar, A. S. Shende, S. R. Satpute and S. H. Sonawane, Ultrason. Sonochem., 2015, 24, 87–97, DOI:10.1016/j.ultsonch.2014.11.009.
- R. B. Birajadara, A. Ghosh, A. Ghule, F. Singh and R. Sharma, Sens. Actuators, B, 2011, 160, 1050–1055, DOI:10.1016/10.1016/j.snb.2011.09.024.
- S. S. Joshi, C. D. Lokhand and S. H. Han, Sens. Actuators, B, 2007, 123, 240–245, DOI:10.1016/j.snb.2006.08.023.
- K. R. Nemade, R. V. Barde and S. A. Waghuley, J. Taibah Univ. Sci., 2015 DOI:10.1016/j.jtusci.2015.03.003.
- P. T. Patil, R. S. Anwane and S. B. Kondawar, Procedia Mater. Sci., 2015, 10, 195–204, DOI:10.1016/j.mspro.2015.06.041.
- Y. T. Ravikiran, S. Kotresh, S. C. Vijayakumari and S. Thomas, Curr. Appl. Phys., 2014, 14(7), 960–964, DOI:10.1016/j.cap.2014.04.015.
- S. Singh, M. Singh, B. C. Yadav, P. Tandon, S. I. Pomogailo, G. I. Dzhardimalieva and A. D. Pomogailo, Sens. Actuators, B, 2011, 160, 826–834, DOI:10.1016/j.snb.2011.08.068.
- A. Singh, A. Singh, S. Singh, P. Tandon, B. C. Yadav and R. R. Yadav, J. Alloys Compd., 2015, 618, 475–483, DOI:10.1016/j.jallcom.2014.08.190.
- N. B. Sonawane, K. V. Gurav, R. R. Ahire, J. H. Kim and B. R. Sankapale, Sens. Actuators, A, 2014, 216, 78–83, DOI:10.1016/j.sna.2014.05.012.
- V. R. Shinde, T. P. Gujar and C. D. Lokhande, Sens. Actuators, B, 2007, 123, 701–706, DOI:10.1016/j.snb.2006.10.003.
- K. V. Gurav, P. R. Deshmukh and C. D. Lokhande, Sens. Actuators, B, 2011, 151, 365–369, DOI:10.1016/j.snb.2010.08.012.
- G. Sberveglieri, Sens. Actuators, B, 1995, 23, 103–109, DOI:10.1016/0925-4005(94)01278-P.
- X. Liu, S. Cheng, H. Liu, S. Hu, D. Zhang and H. Ning, J. Sens., 2012, 12, 9635–9665, DOI:10.3390/s120709635.
- J. W. Han, B. Kim, J. Li and M. Meyyappan, Appl. Phys. Lett., 2013, 102, 1–4, DOI:10.1063/1.4805025.
- T. Guinovart, M. Parrilla, G. A. Crespo, F. X. Rius and F. J. Andrade, The Analyst, 2013, 138, 5208–5215, 10.1039/c3an00710c.
- L. M. Castano and A. B. Flatau, Smart Mater. Struct., 2014, 23, 1–27, DOI:10.1088/0964-1726/23/5/053001.
- S. Mishra, N. G. Shimpi and T. Sen, J. Polym. Res., 2013, 20, 49, DOI:10.1007/s10965-012-0049-5.
- N. G. Shimpi, S. Mishra, D. P. Hansora and U. Savdekar, Indian Patent 3179/MUM/2013, 2013, http://ipindia.nic.in/ipr/patent/journal_archieve/journal_2013/pat_arch_102013/official_journal_25102013_part_i.pdf.
- D. P. Hansora, N. G. Shimpi and S. Mishra, JOM, 2015, 1–14, DOI:10.1007/s11837-015-1522-5.
- V. N. Popov, Mater. Sci. Eng., R, 2004, 43, 61–102, DOI:10.1016/j.mser.2003.10.001.
- B. Mahar, C. Laslau, R. Yip and Y. Sun, IEEE Sens. J., 2007, 7, 266–284, DOI:10.1109/jsen.2006.886863.
- H. Dai, Surf. Sci., 2002, 500, 218–241, DOI:10.1016/S0039-6028(01)01558-8.
- K. Matzinger, Ph.D. theses, Universität Freiburg, 2006, http://doc.rero.ch/record/6666/files/MatzingerK.pdf.
- N. Gupta, S. Sharma, I. A. Mir and D. Kumar, J. Sci. Ind. Res., 2006, 65, 549–557 CAS . http://nopr.niscair.res.in/bitstream/123456789/4862/1/JSIR%2065(7)%20549-557.pdf.
- B. Crawford, D. Esposito, V. Jain, D. Pelletier, C. Mavroidis, Y. J. Jung and A. Khanicheh, Paper 55, Mechanical Engineering Undergraduate Capstone Projects, Final Report, Northeastern University, 2007, http://iris.lib.neu.edu/cgi/viewcontent.cgi?article=1054%26context=mech_eng_capstone Search PubMed.
- A. Abbaspourrad, C. Verissimo, R. V. Gelamo, M. M. Silva, A. R. Vaz, F. P. M. Rouxinol, O. L. Alves and S. A. Moshkalev, in Smart Nanocomposites Research Compendium, ed. K. Levine, Nova Publishers, USA, 1st edn, 2013, vol. 1, ch. 2, pp. 13–20, https://www.novapublishers.com/catalog/product_info.php?products_id=25809 Search PubMed.
- M. Ding, Ph.D. theses, University of Pittsburgh, 2013, http://d-scholarship.pitt.edu/19257/4/Mengning_Ding_ETD_2013.pdf.
- Z. Chen and K. Saito, UCR Review Meeting, University of Kentucky, 2007, http://seca.doe.gov/publications/proceedings/05/UCR_HBCU/pdf/papers/Zhi_Chen.pdf Search PubMed.
- M. D. Volder, D. Reynaerts and C. V. Hoof, Proceeding of the Conference on IEEE Sensors, 2010, 2369–2372 Search PubMed . http://mechanosynthesis.mit.edu/conferences/026_devolder_sensors10_bridgetempsensor.pdf.
- E. Llobet, Sens. Actuators, B, 2013, 179, 32–45, DOI:10.1016/j.snb.2012.11.014.
- A. Misra, Special section: Carbon Technology, Curr. Sci., 2014, 107, 419–429 CAS . http://www.currentscience.ac.in/Volumes/107/03/0419.pdf.
- J. Wang and M. Musameh, Analyst, 2004, 129, 1–2, 10.1039/b313431h.
- D. Jariwala, V. K. Sangwan, L. J. Lauhon, T. J. Marksa and M. C. Hersam, Chem. Soc. Rev., 2013, 42, 2824–2860, 10.1039/c2cs35335k.
- S. Peng and K. Cho, Nano Lett., 2003, 3, 513–517, DOI:10.1021/nl034064u.
- S. Brahim, S. Colbern, R. Gump, A. Moser and L. Grigorian, J. Nanotechnol., 2009, 20, 1–7, DOI:10.1088/0957-4484/20/23/235502.
- Q. Zhao, M. B. Nardelli, W. Lu and J. Bernholc, Nano Lett., 2005, 5, 847–851, DOI:10.1021/nl050167w.
- A. Abdelhalim, A. Abdellah, G. Scarpa and P. Lugli, J. Nanotechnol., 2014, 25, 1–10, DOI:10.1088/0957-4484/25/5/055208.
- Z. Zanolli, R. Leghrib, A. Felten, J. J. Pireaux, E. Llobet and J. C. Charlier, ACS Nano, 2011, 5, 4592–4599, DOI:10.1021/nn200294h.
- I. V. Anoshkin, A. G. Nasibulin, P. R. Mudimela, M. He, V. Ermolov and E. I. Kauppinen, Nano Res., 2013, 6, 77–86, DOI:10.1007/s12274-012-0282-6.
- M. Ding, Y. Tang, P. Gou, M. J. Reber and A. Star, Adv. Mater., 2011, 23, 536–540, DOI:10.1002/adma.201003304.
- D. R. Kauffman, D. C. Sorescu, D. P. Schofield, B. L. Allen, K. D. Jordan and A. Star, Nano Lett., 2010, 10, 958–963, DOI:10.1021/nl903888c.
- X. Mao, G. C. Rutledge and T. A. Hatton, Nanotoday, 2014, 9, 405–432, DOI:10.1016/j.nantod.2014.06.011.
- P. Gajendran and R. Saraswathi, Pure Appl. Chem., 2008, 80, 2377–2395, DOI:10.1351/pac200880112377.
- V. K. Rana, S. Akhtar, S. Chatterjee, S. Mishra, R. P. Singh and C. S. Ha, J. Nanosci. Nanotechnol., 2014, 14, 2425–2435, DOI:10.1166/jnn.2014.8498.
- V. K. Rana, M. C. Choi, J. Y. Kong, G. Y. Kim, M. J. Kim, S. H. Kim, S. Mishra, R. P. Singh and C. S. Ha, Macromol. Mater. Eng., 2011, 296, 131–140, DOI:10.1002/mame.201000307.
- A. Firouzi, S. Sobri, F. M. Yasin and F. L. Ahmadun, Proceeding of the International Conference on Nanotechnology and Biosensors, Singapore, 2011, http://www.ipcbee.com/vol2/39-Y40002.pdf Search PubMed.
- H. Ago, N. Uehara, N. Yoshihara, M. Tsuji, M. Yumura, N. Tomonaga and T. Setoguchi, Carbon, 2006, 44, 2912–2918, DOI:10.1016/j.carbon.2006.05.049.
- T. Yamada, T. Namai, K. Hata, D. N. Futaba, K. Mizuno, J. Fan, M. Yudasaka, M. Yumura and S. Iijima, Nat. Nanotechnol., 2006, 1, 131–136, DOI:10.1038/nnano.2006.95.
- B. Yeole, T. Sen, D. P. Hansora and S. Mishra, J. Appl. Polym. Sci., 2015, 132, 1–9, DOI:10.1002/app.42379.
- J. Huang and R.
B. Kaner, Chem. Commun., 2006, 4, 367–376, 10.1039/b510956f.
- Z. Zanolli and J. C. Charlier, ACS Nano, 2012, 6, 10786–10791, DOI:10.1021/nn304111a.
- Y. Shang, X. He, Y. Li, L. Zhang, Z. Li, C. Ji, E. Shi, P. Li, K. Zhu, Q. Peng, C. Wang, X. Zhang, R. Wang, J. Wei, K. Wang, H. Zhu, D. Wu and A. Cao, Adv. Mater., 2012, 24, 2896–2900, DOI:10.1002/adma.201200576.
- S. Mao, G. Lu and J. Chen, J. Mater. Chem. A, 2014, 2, 5573–5579, 10.1039/c3ta13823b.
- L. Hu, M. Pasta, F. L. Mantia, L. Cui, S. Jeong, H. Dawn, D. Jang, W. Choi, S. M. Han and Y. Cui, Nano Lett., 2010, 10, 708–714, DOI:10.1021/nl903949m.
- N. Liu, W. Ma, J. Tao, X. Zhang, J. Su, L. Li, C. Yang, Y. Gao, D. Golberg and Y. Bando, Adv. Mater., 2013, 25, 4925–4931, DOI:10.1002/adma.201301311.
- L. Kou, T. Huang, B. Zheng, Y. Han, X. Zhao, K. Gopalsamy, H. Sun and C. Gao, Nat. Commun., 2014, 5, 1–10, DOI:10.1038/ncomms4754.
- J. Zhong, Y. Zhang, Q. Zhong, Q. Hu, B. Hu, Z. L. Wang and J. Zhou, ACS Nano, 2014, 8, 6273–6280, DOI:10.1021/nn501732z.
- S. Yao and Y. Zhu, Nanoscale, 2014, 6, 2345–2352, 10.1039/C3NR05496A.
- S. Jung, J. Lee, T. Hyeon, M. Lee and D. H. Kim, Adv. Mater., 2014, 26, 6329–6334, DOI:10.1002/adma.201402439.
- T. Yamada, Y. Hayamizu, Y. Yamamoto, Y. Yomogida, A. I. Najafabadi, D. N. Futaba and K. Hata, Nat. Nanotechnol., 2011, 6, 296–301, DOI:10.1038/nnano.2011.36.
- Y. Li, Y. Shang, X. He, Q. Peng, S. Du, E. Shi, S. Wu, Z. Li, P. Li and A. Cao, ACS Nano, 2013, 7, 8128–8135, DOI:10.1021/nn403400c.
- W. Zeng, L. Shu, Q. Li, S. Chen, F. Wang and X. M. Tao, Adv. Mater., 2014, 26, 5310–5336, DOI:10.1002/adma.201400633.
- Y. H. Lee, J. S. Kim, J. Noh, I. Lee, H. J. Kim, S. Choi, J. Seo, S. Jeon, T. S. Kim, J. Y. Lee and J. W. Choi, Nano Lett., 2013, 13, 5753–5761, DOI:10.1021/nl403860k.
- P. Saini and V. Choudhary, J. Appl. Polym. Sci., 2013, 129, 2832–2839, DOI:10.1002/APP.38994.
- M. Melzer, J. I. Mönch, D. Makarov, Y. Zabila, G. Santiago, C. Bermúdez, D. Karnaushenko, S. Baunack, F. Bahr, C. Yan, M. Kaltenbrunner and O. G. Schmidt, Adv. Mater., 2014, 27, 1274–1280, DOI:10.1002/adma.201405027.
Footnote |
† Electronic supplementary information (ESI) available. See DOI: 10.1039/c5ra16479f |
|
This journal is © The Royal Society of Chemistry 2015 |