DOI:
10.1039/C5RA16301C
(Paper)
RSC Adv., 2015,
5, 86463-86472
Chromogenic naked-eye detection of copper ion and fluoride†
Received
13th August 2015
, Accepted 8th October 2015
First published on 8th October 2015
Abstract
A bi-functional colorimetric chemosensor 1, based on julolidine moiety and N-(2-aminoethyl)-5-nitropyridin-2-amine, has been synthesized and characterized. The sensor 1 has proven to be highly selective and sensitive to Cu2+ with a color change from colorless to yellow in aqueous solution. The sensing mechanism of 1 for Cu2+ was proposed to be the ligand-to-metal charge-transfer (LMCT), which was explained by theoretical calculations. It was also found that the 1–Cu2+ complex could be recycled simply through treatment with an appropriate reagent such as EDTA. Importantly, the sensor 1 could be used to detect and quantify Cu2+ in water samples. Moreover, 1 showed a selective colorimetric response toward fluoride due to the increase in the intramolecular charge transfer (ICT) band by a deprotonation process without any inhibition from other anions such as CH3COO− and CN−.
Introduction
The design and synthesis of new chemosensors for transition metal ions and anions has been of interest to chemists for many years because these ions play important roles in the fields of biological, environmental and chemical systems.1 Among transition metal ions, copper is the third-most abundant transition metal in human, and it plays an important role as a redox catalyst in biological processes such as electron transfer reactions involving oxidation of various organic substrates.2 However, an excess amount of copper in the body would cause gastrointestinal disturbance, damage to the liver and kidney, Alzheimer's disease, Wilson's disease and Parkinson's disease.3 The maximum permissible levels of Cu2+ in drinking water by World Health Organization (WHO) is 2.0 ppm (31.5 μM).4 Therefore, it is important to design and develop Cu(II) sensors with high selectivity and sensitivity.
Among various important anionic analytes, fluoride (F−) is of particular interest owing to its established role in dental care and clinical treatment for osteoporosis.5 However, an acute intake of a large dose or chronic ingestion of lower doses of fluoride can cause many serious disease such as gastric and kidney disorders, dental and skeletal fluorosis, and urolithiasis in humans, and even death.6 Also, they contribute significantly to environmental pollution.7 For these reasons, the improved chemosensor for the detection and sensing of F− with high selectivity is of current interest. However, many anion sensors are not capable of distinguishing fluoride effectively from anions such as CH3COO− and CN−, because they possess similar basicity to F− and easily form hydrogen bonds.8 Therefore, it is highly desirable to develop selective methods capable of discriminating fluoride, especially from competing anions (CH3COO− and CN−).
Up to now, various methods for detecting cations and anions have been developed in a variety of complex environments, such as chromatographic, fluorometric and colorimetric chemosensor, flow injection and electrochemical analysis.9 Among them, colorimetric chemosensors are the most promising in sensor field as it does not need any expensive instrumentation.10 The analyte determination can be carried out by the naked eye under visible light. Therefore, it is of considerable importance to develop probes with colorimetric sensing ability for detection of both Cu2+ and F−.
Julolidine moiety has been well known as a chromophore and chemosensors with the julolidine moiety often showed a good colorimetric response with target analytes.11 On the other hand, the presence of the electron-withdrawing nitro group on the amino-pyridyl moiety would increase not only the degree of π-conjugation but also the hydrogen bonding ability of the amino NH proton.12 Therefore, we designed and synthesized a new chemosensor 1 based on the combination of the julolidine and nitropyridine moieties, and tested its sensing properties towards various metal ions and anions.
Herein, we report a new bi-functional chemosensor 1 for Cu2+ and F−, which was synthesized in one step by condensation reaction of 8-hydroxyl-julolidine-9-carboxaldehyde and N-(2-aminoethyl)-5-nitropyridin-2-amine (Scheme 1). The sensor 1 can detect Cu2+ by color change from colorless to yellow in aqueous solution. Additionally, 1 showed a distinctly red-shifted absorption spectrum with the intense color change in the presence of F−.
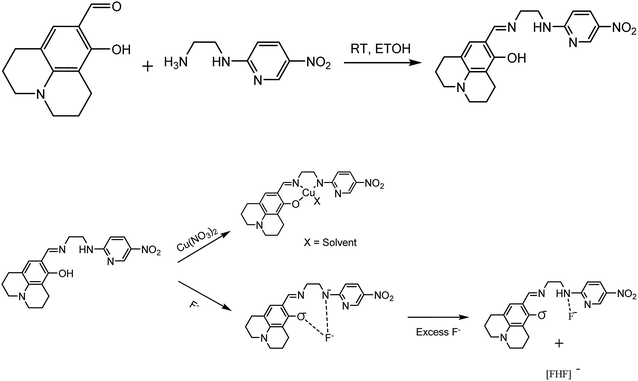 |
| Scheme 1 Synthesis of 1 and sensing mechanisms of Cu2+ and F− by 1. | |
Results and discussion
Synthesis of receptor 1
Receptor 1 was obtained by the combination of 8-hydroxyjulolidine-9-carboxaldehyde and N-(2-aminoethyl)-5-nitropyridine-2-amine with 84% yield in ethanol (Scheme 1), and characterized by 1H NMR and 13C NMR, ESI-mass spectroscopy, and elemental analysis.
Colorimetric signaling of 1 to Cu2+
To verify the selectivity, the absorptive behavior of 1 was investigated upon the addition of various metal ions in acetonitrile/buffer solution (7
:
3; v/v, 10 mM bis–tris, pH 7.0). As shown in Fig. 1, the absorption spectrum of free 1 showed a maximum peak centered at 382 nm. The addition of 12 equiv. of Cu2+ into the 1 solution resulted immediately in a significant enhancement of absorbance at 450 nm with the color change from colorless to yellow (Fig. 1b). Under the identical condition, there were no appreciable changes of the absorption in the presence of Al3+, Zn2+, Cd2+, Mg2+, Cr3+, Co2+, Ni2+, Na+, K+, Ca2+, Mn2+, Pb2+, Fe2+ and Fe3+. Hg2+, Ag+, and Au3+ produced precipitate due to their insolubility in acetonitrile/buffer solution (7
:
3). The results demonstrated that 1 was characteristic of high selectivity toward Cu2+ over other metal ions.
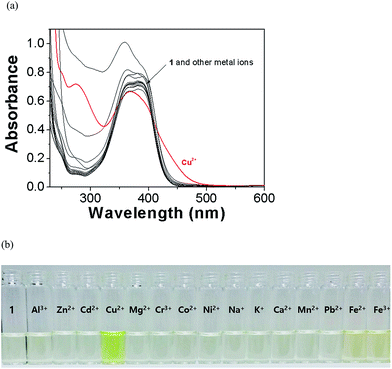 |
| Fig. 1 (a) UV-vis spectral changes of 1 (20 μM) upon addition of 12 equiv. of various metal ions in acetonitrile–water (7 : 3, v/v). (b) Colorimetric changes of 1 (20 μM) upon the addition of various metal ions (12 equiv.). | |
An UV-vis titration experiment was performed to investigate the interaction of Cu2+ and 1 (Fig. 2). Upon the gradual addition of Cu2+ to a solution of 1, the absorbance peak at 382 nm steadily decreased and two new absorption bands at 275 and 450 nm appeared concomitantly, resulting in a color change from colorless to yellow. The isosbestic point at 350 nm was clearly observed, indicating the formation of a single species between 1 and Cu2+. Moreover, the new peak at 450 nm in the visible region with molar extinction coefficient in the thousands, 7.32 × 103 M−1 cm−1, is too large to be Cu-based d–d transitions and thus must be ligand-based transitions.13 Therefore, we proposed that the new peak at 450 nm might be attributed to a ligand-to-metal charge-transfer (LMCT).
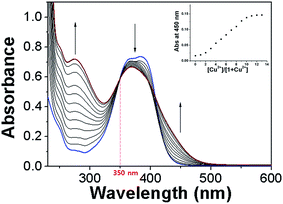 |
| Fig. 2 Absorption spectral changes of 1 (20 μM) after addition of increasing amounts of Cu2+ in acetonitrile–water (7 : 3, v/v). Inset: absorption at 450 nm versus the number of equiv. of Cu2+ added. | |
A Job plot analysis showed a 1
:
1 stoichiometry for the 1–Cu2+ complex (Fig. S1†), which was further confirmed by ESI-mass spectrometry analysis (Fig. S2†). The positive ion mass spectrum of ESI-mass indicated that a peak at m/z = 520.73 was assignable to [1(–H+) + Cu2+ + DMSO]+ [cald, m/z = 521.12]. Based on Job plot, ESI-mass spectrometry and the crystal structures of similar type of Cu complexes reported in the literature,14 we proposed the structure of 1
:
1 complex of 1 and Cu2+ as shown in Scheme 1.
From UV-vis titration data, the association constant for 1–Cu2+ complex was determined to be 1.5 × 103 M−1 using Benesi–Hildebrand equation15 (Fig. S3†). This value was within the range of those (103 to 1012) reported for Cu2+ sensing chemosensors.16 The detection limit (3σ/K)17 of receptor 1 for the analysis of Cu2+ was calculated to be 23.5 μM (Fig. S4†). The detection limit of 1 for Cu2+ is lower than that (31.5 μM) recommended by World Health Organization (WHO) in drinking water.4 Therefore, 1 could be a good indicator for the detection of copper ions in drinking water.
To explore the anti-disturbance of 1 as the Cu2+-selective sensor, competition experiments were performed (Fig. 3). For this purpose, 1 was treated with 12 equiv. of Cu2+ in the presence of the same concentration of other metal ions. The absorbance at 450 nm caused by Cu2+ was retained with Zn2+, Cd2+, Mg2+, Cr3+, Co2+, Ni2+, Na+, K+, Ca2+, Mn2+, Fe3+ and Pb2+. Instead, the absorbance of 1–Cu2+ complex in the presence of Al3+ and Fe2+ was relatively low. In order to overcome the inhibitions, we have added iodide to 1–Cu2+–Al3+ solution and fluoride to 1–Cu2+–Fe2+ one. The resulting absorbance of 1–Cu2+ complex in the presence of Al3+ and Fe2+ were recovered to 89% and 84%, respectively (Fig. S5†). Thus 1 can be used as a selective colorimetric sensor for Cu2+ detection in the presence of most competing metal ions.
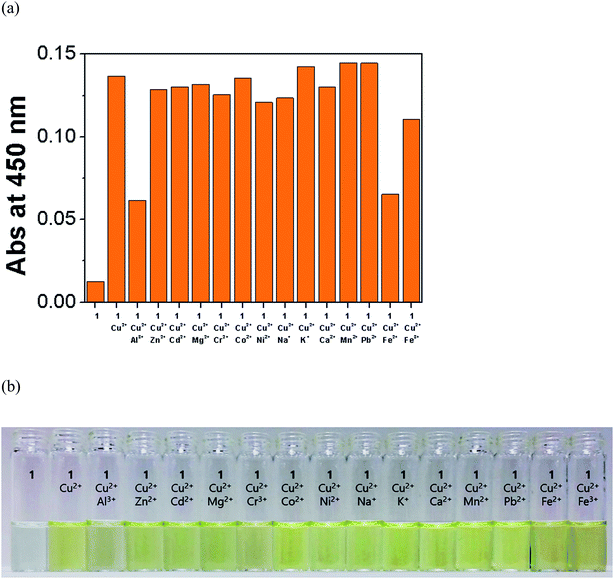 |
| Fig. 3 (a) Competitive selectivity of 1 (20 μM) toward Cu2+ (12 equiv.) in the presence of other metal ions (12 equiv.) in acetonitrile–water (7 : 3, v/v). (b) Colorimetric changes of 1 (20 μM) in the presence of Cu2+ (12 equiv.) and other metal ions (12 equiv.). | |
To study the practical applicability, the colorimetric responses of 1 in the absence and presence of Cu2+ in different pH values were evaluated. As shown in Fig. 4, the receptor 1 itself was stable from pH 2 to 12. Upon the addition of Cu2+, there was an apparent increase of absorbance (450 nm) at the pH range of 7–12. These results indicated that Cu2+ could be clearly detected by the naked eye or UV-vis absorption measurements using 1 over the wide pH range.
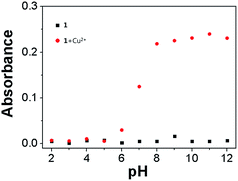 |
| Fig. 4 Absorbance of 1 upon addition of 12 equiv. of Cu2+ at various range of pH. | |
We subsequently studied the binding reversibility of Cu2+ to 1 in acetonitrile–water solution. Due to the high stability constant for the EDTA–Cu2+ complex, it was expected that EDTA would chelate Cu2+ from the 1–Cu2+ complex, liberating 1. As shown in Fig. 5, the absorbance of 1–Cu2+ was quenched and the color changed from yellow to colorless upon the addition of 12 equiv. EDTA. Introduction of an additional Cu2+ resulted in the recovery of absorbance and color, indicating that the binding of 1 with Cu2+ is chemically reversible. The absorbance (Fig. 5a) and color changes (Fig. 5b) were almost reversible even after several cycles with the sequentially alternative addition of Cu2+ and EDTA.
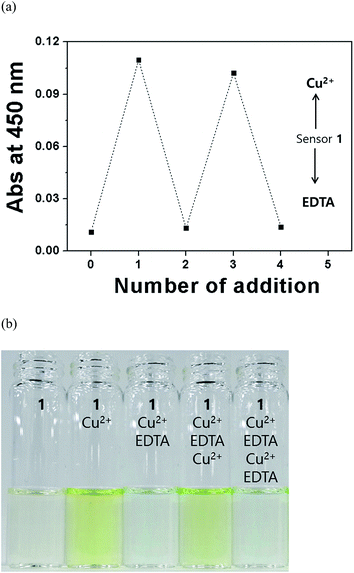 |
| Fig. 5 (a) Absorbance of 1 (20 μM) after the sequential addition of Cu2+ and EDTA. (b) The color changes of 1 (20 μM) after the sequential addition of Cu2+ and EDTA. | |
In order to study the applicability of the sensor 1 in environmental water samples, we constructed a calibration curve for the determination of Cu2+ by 1 (Fig. S6†). It exhibited a good linear relationship between the absorbance of 1 and Cu2+ concentrations (0–10 μM) with a correlation coefficient of R2 = 0.9912 (n = 3), which means that 1 could be suitable for the quantitative detection of Cu2+. Then, the sensor was applied for the determination of Cu2+ in tap water samples. The results were summarized in Table 1, which exhibited a satisfactory recovery and R.S.D. values for the tap water samples.
Table 1 Determination of Cu(II) in water samplesa
Sample |
Cu(II) added (μmol L−1) |
Cu(II) found (μmol L−1) |
Recovery (%) |
R.S.D (n = 3) (%) |
Conditions: [1] = 10 μmol L−1, water–acetonitrile (3 : 7, v/v) at pH 7.0 buffered by 10 mmol L−1 bis–tris. |
Tap water |
0.00 |
0.23 |
|
0.06 |
|
6.00 |
6.24 |
100.2 |
0.01 |
To understand the sensing mechanisms of 1 toward Cu2+, theoretical calculations were performed with the 1
:
1 stoichiometry, based on Job plot and ESI-mass spectrometry analysis. To get the energy-minimized structures of 1 and 1–Cu2+ complex, the geometric optimizations were performed by DFT/B3LYP level. Especially, 1–Cu2+ complex was considered by a paramagnetic character (S = 1/2, DFT/uB3LYP/main group atom: 6-31G** and Cu: Lanl2DZ/ECP). The significant structural properties of the energy-minimized structures were shown in Fig. 6. The energy minimized structure of 1 showed a V-type structure with the dihedral angle of 1N, 2N, 3C, 4O = 37.664°, and the hydrogen bond was observed between 2N and 6H (Fig. 6a). 1–Cu2+ complex exhibited a square planar structure (dihedral angle (1N, 2N, 3C, 4O) = 8.738°) with the coordination of N, N and O atoms of 1 (Fig. 6b).
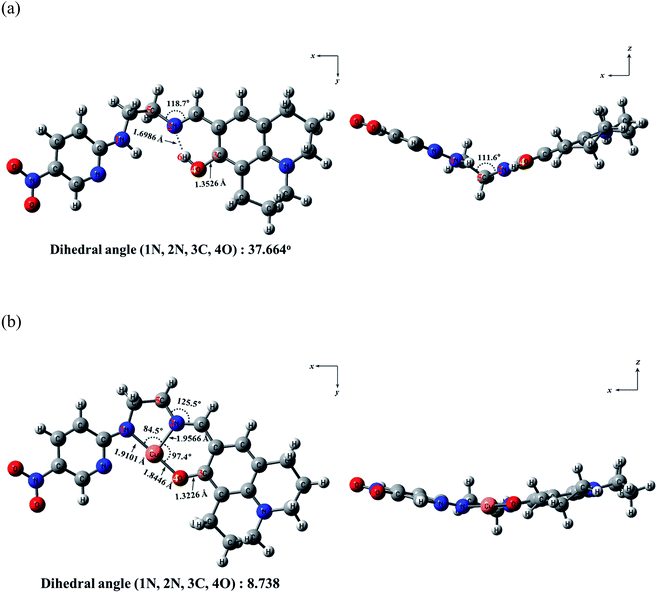 |
| Fig. 6 The energy-minimized structures of (a) 1 and (b) 1–Cu2+ complex. | |
We also investigated the absorption to the singlet excited states of 1 and 1–Cu2+ species via TDDFT calculations. In case of 1, the main molecular orbital (MO) contribution of the first lowest excited state was determined for HOMO−2 → LUMO and HOMO → LUMO+1 transitions (341.34 nm, Fig. S7†), which indicated ICT bands. 1–Cu2+ complex showed that the excited states of 4th, 9th and 12th (497.47, 403.68 and 377.69 nm) were relevant to the color change (colorless to yellow) with predominant ICT and LMCT characters (Fig. S8–S10†). Thus, the chelation of Cu2+ with 1 mainly showed the ICT and LMCT, which induced the different color change of 1 in the presence of Cu2+.
Colorimetric signaling of 1 to F−
The colorimetric sensing properties of 1 were also investigated towards various anions (CN−, OAc−, F−, Cl−, Br−, I−, H2PO4−, BzO−, N3− and SCN−) in DMSO as shown in Fig. 7. Upon the addition of 30 equiv. of various anions to 1, there was no significant UV-vis spectral change in the nature of spectra except for F− (Fig. 7a). In the presence of F−, the receptor 1 showed a distinct spectral change and a color change from colorless to orange with an immediate response time, which was sufficiently distinct to be discriminated from other anions through naked-eye (Fig. 7b).
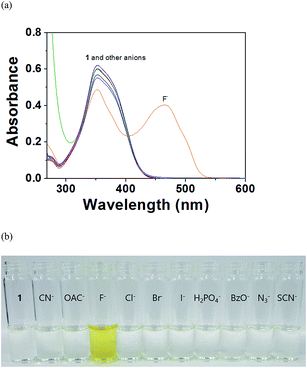 |
| Fig. 7 (a) UV-vis spectral changes of 1 (20 μM) upon addition of 30 equiv. of various anions in DMSO. (b) Colorimetric changes of 1 (20 μM) upon the addition of various anions (30 equiv.). | |
The interaction of receptor 1 with F− was studied in detail by UV-vis spectroscopic titration, as shown in Fig. 8. On gradual addition of F−, the band at 350 nm decreased, and a new band at 465 nm increased drastically. The absorbance reached a maximum at 30 equiv. of fluoride with a clear isosbestic point observed at 406 nm, indicating the formation of the only one species between 1 and F−. The color change of 1 from colorless to orange might be due to the deprotonation of phenol of 1 by F− (Scheme 1). To further confirm the sensing mechanism between 1 and F−, the interaction between 1 and OH− was also investigated (Fig. S11†). UV-vis spectral change of 1 upon addition of OH− was nearly identical to that of 1 obtained from the addition of F−, which indicated the deprotonation between 1 and F−. The resulting negative charge on the phenol is introduced in the receptor which causes intramolecular charge transfer (ICT) between the electron deficient –NO2 group and the electron rich –O− to show the UV-vis and colorimetric changes.18
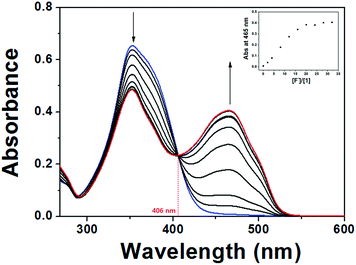 |
| Fig. 8 Absorption spectral changes of 1 (20 μM) after addition of increasing amounts of F− in DMSO. Inset: absorption at 465 nm versus the number of equiv of F− added. | |
The Job plot for the binding between 1 and F− exhibited a 1
:
1 stoichiometry (Fig. S12†), which was further supported by ESI-mass spectrometry analysis (Fig. S13†). The negative ion mass spectrum of ESI-mass indicated that a peak at m/z = 380.67 was assignable to [1–H+]− [calcd, 380.17], which is corresponding to the receptor 1 deprotonated by fluoride. From the UV-vis titration data, the association constant for 1 with F− was determined as 5.9 × 102 M−1 using the Benesi–Hildebrand equation15 (Fig. S14†). The detection limit (3σ/K)17 of receptor 1 for the analysis of F− was calculated to be 19.4 μM (Fig. S15†).
To study the interaction between 1 and F−, the 1H NMR titrations of 1 were measured with different amounts of F− (Fig. 9). In the absence of F−, the phenolic OH proton (H12) and the amine NH proton (H8) appeared as a singlet at 13.56 and 8.25 ppm, respectively. Upon the gradual addition of F−, the singlets of H12 and H8 were broadened and finally disappeared due to hydrogen bonding with F−. Simultaneously, the H6,7 protons and the protons of nitropyridine moiety (H9–11) were shifted upfield. These results suggested that the –NH and –OH protons might hydrogen bond to F−, and eventually undergo the deprotonation (Scheme 1).
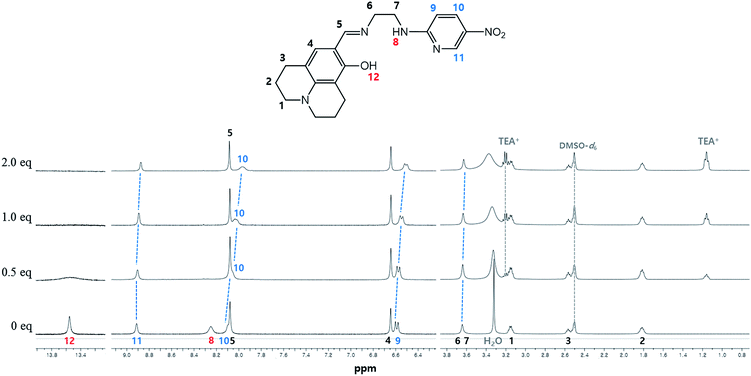 |
| Fig. 9 1H NMR titration of 1 with F−. | |
To test whether 1 can detect F− selectively even in the presence of other anions, competitive experiment was conducted (Fig. 10). 1 was treated with 30 equiv. of F− in the presence of several anions. The results indicated that the competitive anions did not lead to any significant spectral (Fig. 10a) and color change (Fig. 10b), and that F− ions still resulted in the similar absorbance and color in the presence of competitive anions. Thus, 1 could be used as a selective chemosensor for F− by the colorimetric mode.
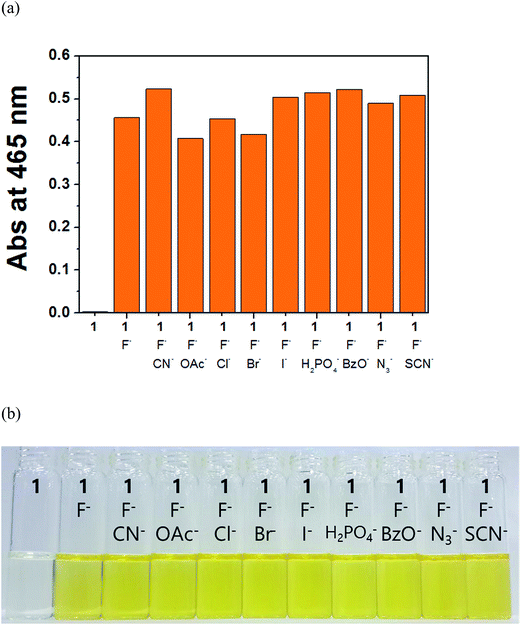 |
| Fig. 10 (a) Competitive selectivity of 1 (20 μM) toward F− (30 equiv.) in the presence of other anions (30 equiv.) in DMSO. (b) Colorimetric changes of 1 (20 μM) in the presence of F− (30 equiv.) and other anions (30 equiv.). | |
Conclusion
We synthesized a bi-functional colorimetric chemosensor based on the combination of 8-hydroxyljulolidine-9-carboxaldehyde and N-(2-aminoethyl)-5-nitropyridin-2-amine. The sensor 1 exhibited a colorimetric response from colorless to yellow upon binding to Cu2+ in aqueous solution. The binding of the sensor 1 and Cu2+ was also chemically reversible with EDTA. The recovery studies of the water samples added with Cu2+ showed a satisfactory recovery and R.S.D. values. Additionally, the sensing mechanism of 1 toward Cu2+ was explained by theoretical calculations. Furthermore, 1 can selectively detect F− among the various anions with the colorimetric change from colorless to orange. In particular, 1 can distinguish F− in the presence of other anions such as CH3COO− and CN−. These results demonstrated that chemosensor 1 will be useful for development of new chemosensor for recognizing both cations and anions.
Experimental
Materials and equipment
All the solvents and reagents (analytical and spectroscopic grade) were purchased from Sigma-Aldrich. 1H and 13C NMR spectra were recorded on a Varian 400 MHz and 100 MHz spectrometer and chemical shifts were recorded in ppm. Electro spray ionization mass spectra (ESI-MS) were collected on a Thermo Finnigan (San Jose, CA, USA) LCQTM Advantage MAX quadrupole ion trap instrument by infusing samples directly into the source using a manual method. Spray voltage was set at 4.2 kV, and the capillary temperature was at 80 °C. Absorption spectra were recorded at room temperature using a Perkin Elmer model Lambda 25 UV/Vis spectrometer. Elemental analysis for carbon, nitrogen and hydrogen was carried out using a Flash EA 1112 elemental analyzer (thermo) at the Organic Chemistry Research Center of Sogang University, Korea.
Synthesis of receptor 1
An ethanolic solution of 8-hydroxyjulolidine-9-carboxaldehyde (0.23 g, 1 mmol) was added to N-(2-aminoethyl)-5-nitropyridine-2-amine (0.22 g, 1.2 mmol) in ethanol (10 mL). The reaction solution was stirred for 2 d at room temperature. The yellow powder was filtered and washed with ethanol and diethyl ether. The yield: 0.32 g (84%); 1H NMR (400 MHz DMSO-d6, ppm): δ 13.54 (s, 1H), 8.90 (s, 1H), 8.22 (s, 1H), 8.09 (s, 1H), 8.08 (s, 1H), 6.64 (s, 1H), 6.60 (d, J = 8 MHz, 1H), 3.64 (s, 4H), 3.17 (m, J = 6 MHz, 4H), 2.58 (t, J = 6 MHz), 1.84 (m, J = 6 MHz, 4H); 13C NMR (100 MHz DMSO-d6, ppm): 165.14, 161.48, 159.33, 146.79, 145.84, 134.26, 131.79, 128.83, 111.54, 108.60, 107.29, 105.59, 56.03, 49.29, 48.91, 42.00, 26.61, 21.60, 20.68, 20.07. ESI-MS m/z (M + H+): calcd, 382.19; found, 382.33. Anal. Calc. for C20H23N5O3: C, 62.98; H, 6.08; N, 18.36. Found: C, 63.21; H, 5.93; N, 18.27%.
UV-vis titration
For Cu2+, the UV-vis titration experiment of 1 was carried out by adding aliquots of 3–39 μL of Cu(NO3)2·2.5H2O solution (20 mM) to 3 mL of 1 solution (20 μM, 7
:
3, acetonitrile/bis–tris buffer v/v; 10 mM bis–tris, pH 7.0). The spectra were recorded a minute after the solution was completely mixed.
For F−, the UV-vis titration experiment of 1 was carried out by adding aliquots of 6–60 μL of tetraethyl ammonium fluoride (TEAF) solution (100 mM) to 3 mL of 1 solution (20 μM in DMSO). The spectra were recorded a minute after the solution was completely mixed.
Job plot measurements
For Cu2+, stock solution of receptor 1 (10 mM) was prepared in 1 mL of acetonitrile/bis–tris buffer (7
:
3; v/v). Cu2+ solution (10 mM) was prepared by dissolving nitrate salts in acetonitrile/bis–tris buffer (7
:
3; v/v, 1 mL). 30, 27, 24, 21, 18, 15, 12, 9, 6 and 3 μL of the 1 solution were taken and transferred to vials. Each vial was diluted with acetonitrile/bis–tris buffer (7
:
3; v/v) to make a total volume of 2.97 mL. Then, 0, 3, 6, 9, 12, 15, 18, 21, 24, 27 and 30 μL of the Cu2+ solution were added to each diluted 1 solution. Each vial had a total volume of 3 mL. After shaking the vials for a minute, UV-vis spectra were taken at room temperature.
For F−, stock solution of receptor 1 (10 mM) was prepared in 1 mL of DMSO. F− solution (10 mM) was prepared by dissolving TEAF salt in DMSO (1 mL). 15, 13.5, 12, 10.5, 9, 7.5, 6, 4.5, 3 and 1.5 μL of the 1 solution were taken and transferred to vials. Each vial was diluted with DMSO to make a total volume of 2.985 mL. Then, 0, 1.5, 3, 4.5, 6, 7.5, 9, 10.5, 12, 13.5 and 15 μL of the F− solution were added to each diluted 1 solution. Each vial had a total volume of 3 mL. After shaking the vials for a minute, UV-vis spectra were taken at room temperature.
Competition experiments
For Cu2+, stock solution of receptor 1 (5 mM) was prepared in 1 mL of acetonitrile/bis–tris buffer (7
:
3; v/v). Solution samples (20 mM) of metal ions were prepared by dissolving the corresponding salts in a mixture of acetonitrile/bis–tris buffer (7
:
3; v/v, 1 mL). 36 μL aliquot of each metal-ion stock solution and 36 μL of Cu2+ solution were diluted into a 3 mL of mixture of acetonitrile/bis–tris buffer (7
:
3; v/v). Then, 12 μL of the 1 solution was added into the mixed solution to make 20 μM. After shaking the vials for a minute, UV-vis spectra were taken at room temperature.
For F−, stock solution of receptor 1 (5 mM) was prepared in 1 mL of DMSO. Solution samples (20 mM) of anions were prepared by dissolving the corresponding salts in DMSO (1 mL). 48 μL aliquot of each anion stock solution and 48 μL of F− solution were diluted into a 3 mL of DMSO. Then, 12 μL of the 1 solution was added into the mixed solution to make 20 μM. After shaking the vials for a minute, UV-vis spectra were taken at room temperature.
Theoretical calculation methods of 1 with Cu2+
All DFT/TDDFT calculations based on the hybrid exchange-correlation functional B3LYP19 were carried out using Gaussian 03 program.20 The 6-31G** basis set21 was used for the main group elements, whereas the Lanl2DZ effective core potential (ECP)22 was employed for Cu. In vibrational frequency calculations, there was no imaginary frequency for the optimized geometries of 1 and 1–Cu2+ complex, suggesting that these geometries represented local minima. For all calculations, the solvent effect of water was considered by using the Cossi and Barone's CPCM (conductor-like polarizable continuum model).23 To investigate the electronic properties of singlet excited states, time-dependent DFT (TDDFT) was performed in the ground state geometries of 1 and 1–Cu2+. The 35 singlet–singlet excitations were calculated and analyzed. The GaussSum 2.1 (ref. 24) was used to calculate the contributions of molecular orbitals in electronic transitions.
1H NMR titration of 1 with F−
For 1H NMR titration of receptor 1 with F−, four NMR tubes of receptor 1 (3.81 mg, 0.01 mmol) dissolved in DMSO-d6 (700 μL) were prepared and then four different concentrations (0, 0.005, 0.01 and 0.02 mmol) of TEAF dissolved in DMSO-d6 were added to each solution of receptor 1. After shaking them for a minute, 1H NMR spectra were obtained at room temperature.
Acknowledgements
Basic Science Research Program through the National Research Foundation of Korea (NRF) funded by the Ministry of Education, Science and Technology (NRF-2014R1A2A1A11051794 and NRF-2015R1A2A2A09001301) is gratefully acknowledged. We thank Nano-Inorganic Laboratory, Department of Nano & Bio Chemistry, Kookmin University to access the Gaussian 03 program packages.
References
-
(a) M. Kumar, N. Kumar and V. Bhalla, Dalton Trans., 2012, 41, 10189–10193 RSC;
(b) D. Karak, S. Lohar, A. Banerjee, A. Sahana, I. Hauli, S. K. Mukhopadhyay, J. S. Matalobos and D. Das, RSC Adv., 2012, 2, 12447–12454 RSC;
(c) Y. Peng, Y.-M. Dong, M. Dong and Y.-M. Wang, J. Org. Chem., 2012, 77, 9072–9080 CrossRef CAS PubMed;
(d) X. Chen, S. Nam, G. Kim, N. Song, Y. Jeong, I. Shin, S. K. Kim, J. Kim, S. Park and J. Yoon, Chem. Commun., 2010, 46, 8953–8955 RSC;
(e) S. Devaraj, V. S. Elanchezhian and M. Kandaswamy, Inorg. Chem. Commun., 2011, 14, 1596–1601 CrossRef CAS PubMed.
-
(a) E. Gaggelli, H. Kozlowski, D. Valensin and G. Valensin, Chem. Rev., 2006, 106, 1995–2044 CrossRef CAS PubMed;
(b) I. A. Koval, P. Gamez, C. Belle, K. Selmeczi and J. Reedijk, Chem. Soc. Rev., 2006, 35, 814–840 RSC;
(c) D. Maiti, H. R. Lucas, A. A. N. Sarjeant and K. D. Karlin, J. Am. Chem. Soc., 2007, 129, 6998–6999 CrossRef CAS PubMed;
(d) Y. J. Na, Y. W. Choi, J. Y. Yun, K.-M. Park, P.-S. Chang and C. Kim, Spectrochim. Acta, Part A, 2015, 136, 1649–2165 CrossRef CAS PubMed.
-
(a) R. Krämer, Angew. Chem., Int. Ed., 1998, 37, 723–772 CrossRef;
(b) Y. K. Jang, U. C. Nam, H. L. Kwon, I. H. Hwang and C. Kim, Dyes Pigm., 2013, 99, 6–13 CrossRef CAS PubMed;
(c) J. Y. Noh, G. J. Park, Y. J. Na, H. Y. Jo, S. A. Lee and C. Kim, Dalton Trans., 2014, 43, 5652–5656 RSC;
(d) H. Kim, Y. J. Na, E. J. Song, K. B. Kim, J. M. Bae and C. Kim, RSC Adv., 2014, 4, 22463–22469 RSC;
(e) S. Wu, T. Wang and S. Liu, Tetrahedron, 2010, 66, 9655–9658 CrossRef CAS PubMed;
(f) J. Yeh, W. Chen, S. Liu and S. Wu, New J. Chem., 2014, 38, 4434–4439 RSC;
(g) H. Yang, H. Song, Y. Zhu and S. Yang, Tetrahedron Lett., 2012, 53, 2026–2029 CrossRef CAS PubMed.
- WHO, WHO guidelines values for chemicals that are of health significance in drinking water, Guidelines for Drinking Water Quality, Geneva, 3rd edn, 2008 Search PubMed.
-
(a) J. H. Kim, J. Vicens and J. S. Kim, Tetrahedron Lett., 2009, 50, 983–987 CrossRef PubMed;
(b) J. Shao, H. Lin and H. K. Lin, Talanta, 2008, 77, 273–277 CrossRef CAS PubMed;
(c) D. A. Jose, D. K. Kumar, B. Ganguly and A. Das, Org. Lett., 2004, 6, 3445–3448 CrossRef CAS PubMed;
(d) R. G. Schamschula, Annu. Rev. Nutr., 1981, 1, 427–435 CrossRef CAS PubMed;
(e) H. S. Horowitz, J. Publ. Health Dent., 2003, 63, 3–81 CrossRef PubMed;
(f) M. Kleerekoper, Endocrinol. Metab. Clin. North Am., 1998, 27, 441 CrossRef CAS;
(g) G. J. Park, H. Y. Jo, K. Y. Ryu and C. Kim, RSC. Adv., 2014, 4, 63882–63890 RSC.
-
(a) Y. Kim and F. P. Gabbaï, J. Am. Chem. Soc., 2009, 131, 3363–3369 CrossRef CAS PubMed;
(b) K. Tayade, S. K. Sahoo, A. Singh, N. Singh, P. Mahulikar, S. Attarde and A. Kuwar, Sens. Actuators, B, 2014, 202, 1333–1337 CrossRef CAS PubMed;
(c) A. Bamesberger, C. Schwartz, Q. Song, W. Han, Z. Wang and H. Cao, New J. Chem., 2014, 38, 884–888 RSC;
(d) P. G. Sutariya, N. R. Modi, A. Pandya, B. K. Joshi, K. V. Joshia and S. K. Menon, Analyst, 2012, 137, 5491–5494 RSC;
(e) L. Zang, D. Wei, S. Wang and S. Jiang, Tetrahedron, 2012, 68, 636–641 CrossRef CAS PubMed;
(f) S. Sharma, M. S. Hundal and G. Hundal, Tetrahedron Lett., 2013, 54, 2423–2427 CrossRef CAS PubMed.
-
(a) B. Moss, Chem. Ind., 1996, 11, 407–411 Search PubMed;
(b) J. M. Tomich, D. Wallace, K. Henderson, K. E. Mitchell, G. Radke, R. Brandt, C. Ambler, A. J. Scott, J. Grantham, L. Sullivan and T. Iwamoto, Biophys. J., 1998, 74, 256–267 CrossRef CAS;
(c) S. Matsuo, K. Kiyomiya and M. Kurebe, Arch. Toxicol., 1998, 72, 798–806 CrossRef CAS;
(d) D. Briancon, Rev. Rhum., 1997, 64, 78–81 CAS.
- T. G. Jo, Y. J. Na, J. J. Lee, M. M. Lee, S. Y. Lee and C. Kim, New J. Chem., 2015, 39, 2580–2587 RSC.
-
(a) V. A. Fassel, Science, 1978, 202, 183–191 CAS;
(b) W. Yang, D. Jaramillo, J. J. Gooding, D. B. Hibbert, R. Zhang, G. D. Willet and K. J. Fishera, Chem. Commun., 2001, 19, 1982–1983 RSC;
(c) A. P. S. Gonsales, M. A. Firmino, C. S. Nomura, F. R. P. Rocha, P. V. Olivera and I. Gaubeur, Anal. Chim. Acta, 2009, 636, 198–204 CrossRef PubMed;
(d) J. S. Becker, A. Matusch, C. Depboylu, J. Dobrowolska and M. V. Zoriy, Anal. Chem., 2007, 79, 6074–6080 CrossRef CAS PubMed.
-
(a) J. Xu, K. Liu, D. Di, S. Shao and Y. Guo, Inorg. Chem. Commun., 2007, 10, 681–684 CrossRef CAS PubMed;
(b) C. Suksai and T. Tuntulani, Chem. Soc. Rev., 2003, 32, 192–202 RSC;
(c) C. Liu, J. Xu, F. Yang, W. Zhou, Z. Li, L. Wei and M. Yu, Sens. Actuators, B, 2015, 212, 364–370 CrossRef CAS PubMed.
-
(a) N. Narayanaswamy and T. Govindaraju, Sens. Actuators, B, 2012, 161, 304–310 CrossRef CAS PubMed;
(b) D. Maity, A. K. Manna, D. Karthigeyan, T. K. Kundu, S. K. Pati and T. Govindaraju, Chem.–Eur. J., 2011, 17, 11152–11161 CrossRef CAS PubMed;
(c) Y. J. Na, I. H. Hwang, H. Y. Jo, S. A. Lee, G. J. Park and C. Kim, Inorg. Chem. Commun., 2013, 35, 342–345 CrossRef CAS PubMed;
(d) J. Y. Noh, S. Kim, I. H. Hwang, G. Y. Lee, J. Kang, S. H. Kim, J. Min, S. Park and C. Kim, Dyes Pigm., 2013, 99, 1016–1021 CrossRef CAS PubMed.
- M. Iniya, D. Jeyanthi, K. Krishnaveni and D. Chellappa, J. Lumin., 2015, 157, 383–389 CrossRef CAS PubMed.
-
(a) E. J. Song, J. Kang, G. R. You, G. J. Park, Y. Kim, S. J. Kim, C. Kim and R. G. Harrison, Dalton Trans., 2013, 42, 15514–15520 RSC;
(b) G. R. You, G. J. Park, J. J. Lee and C. Kim, Dalton Trans., 2015, 44, 9120–9129 RSC.
- S. S. Qian, X. S. Cheng, Z. L. You and H. L. Zhu, Russ. J. Coord. Chem., 2013, 39, 704–709 CrossRef CAS.
- H. A. Benesi and J. H. Hildebrand, J. Am. Chem. Soc., 1949, 71, 2703–2707 CrossRef CAS.
-
(a) F. Yu, W. Zhang, P. Li, Y. Xing, L. Tong, J. Ma and B. Tang, Analyst, 2009, 134, 1826–1833 RSC;
(b) Y. Xiang, A. Tong, P. Jin and Y. Ju, Org. Lett., 2006, 8, 2863–2866 CrossRef CAS PubMed;
(c) S. Wu, K. Du and Y. Sung, Dalton Trans., 2010, 39, 4363–4368 RSC;
(d) G. H. Wu, D. X. Wang, D. Y. Wu, Y. Gao and Z. Q. Wang, J. Chem. Sci., 2009, 121, 543–548 CrossRef CAS;
(e) D. Udhayakumari, S. Velmathi, W.-C. Chen and S.-P. Wu, Sens. Actuators, B, 2014, 204, 375–381 CrossRef CAS PubMed;
(f) G. Li, F. Tao, H. Wang, Y. Li and L. Wang, Sens. Actuators, B, 2015, 211, 325–331 CrossRef CAS PubMed;
(g) G. Li, F. Tao, H. Wang, L. Wang, J. Zhang, P. Ge, L. Liu, T. Tong and S. Sun, RSC Adv., 2015, 5, 18983–18989 RSC;
(h) L. Liu, A. Wang, G. Wang, J. Li and Y. Zhou, Sens. Actuators, B, 2015, 215, 388–395 CrossRef CAS PubMed;
(i) S. Hu, J. Song, F. Zhao, X. Meng and G. Wu, Sens. Actuators, B, 2015, 215, 241–248 CrossRef CAS PubMed;
(j) J. J. Lee, Y. W. Choi, G. R. You, S. Y. Lee and C. Kim, Dalton Trans., 2015, 44, 13305–13314 RSC.
- Y. K. Tsui, S. Devaraj and Y. P. Yen, Sens. Actuators, B, 2012, 161, 510–519 CrossRef CAS PubMed.
-
(a) M. A. Kaloo and J. Sankar, Analyst, 2013, 138, 4760–4763 RSC;
(b) R. Sheng, P. Wang, Y. Gao, Y. Wu, W. Liu, J. Ma, H. Li and S. Wu, Org. Lett., 2008, 10, 5015–5018 CrossRef CAS PubMed;
(c) Y. Jiang, H. Zhao, Y. Q. Lin, N. N. Zhu, Y. R. Ma and L. Q. Mao, Angew. Chem., Int. Ed., 2010, 122, 4910–4914 CrossRef PubMed;
(d) S. Devaraj, D. Saravanakumar and M. Kandaswamy, Sens. Actuators, B, 2009, 136, 13–19 CrossRef CAS PubMed.
-
(a) A. D. Becke, J. Chem. Phys., 1993, 98, 5648–5652 CrossRef CAS PubMed;
(b) C. Lee, W. Yang and R. G. Parr, Phys. Rev. B: Condens. Matter Mater. Phys., 1988, 37, 785–789 CrossRef CAS.
- M. J. Frisch, G. W. Trucks, H. B. Schlegel, G. E. Scuseria, M. A. Robb, J. R. Cheeseman, J. A. Montgomery Jr, T. Vreven, K. N. Kudin, J. C. Burant, J. M. Millam, S. S. Iyengar, J. Tomasi, V. Barone, B. Mennucci, M. Cossi, G. Scalmani, N. Rega, G. A. Petersson, H. Nakatsuji, M. Hada, M. Ehara, K. Toyota, R. Fukuda, J. Hasegawa, M. Ishida, T. Nakajima, Y. Honda, O. Kitao, H. Nakai, M. Klene, X. Li, J. E. Knox, H. P. Hratchian, J. B. Cross, V. Bakken, C. Adamo, J. Jaramillo, R. Gomperts, R. E. Stratmann, O. Yazyev, A. J. Austin, R. Cammi, C. Pomelli, J. W. Ochterski, P. Y. Ayala, K. Morokuma, G. A. Voth, P. Salvador, J. J. Dannenberg, V. G. Zakrzewski, S. Dapprich, A. D. Daniels, M. C. Strain, O. Farkas, D. K. Malick, A. D. Rabuck, K. Raghavachari, J. B. Foresman, J. V. Ortiz, Q. Cui, A. G. Baboul, S. Clifford, J. Cioslowski, B. B. Stefanov, G. Liu, A. Liashenko, P. Piskorz, I. Komaromi, R. L. Martin, D. J. Fox, T. Keith, M. A. Al-Laham, C. Y. Peng, A. Nanayakkara, M. Challacombe, P. M. W. Gill, B. Johnson, W. Chen, M. W. Wong, C. Gonzalez and J. A. Pople, Gaussian 03, Revision D.01, Gaussian, Inc., Wallingford CT, 2004 Search PubMed.
-
(a) P. J. Hay and W. R. Wadt, J. Chem. Phys., 1985, 82, 270–283 CrossRef CAS PubMed;
(b) P. J. Hay and W. R. Wadt, J. Chem. Phys., 1985, 82, 284–298 CrossRef PubMed.
- P. J. Hay and W. R. Wadt, J. Chem. Phys., 1985, 82, 299–310 CrossRef CAS PubMed.
-
(a) V. Barone and M. Cossi, J. Phys. Chem. A, 1998, 102, 1995–2001 CrossRef CAS;
(b) M. Cossi and V. Barone, J. Chem. Phys., 2001, 115, 4708–4717 CrossRef CAS PubMed.
- M. M. O’Boyle, A. L. Tenderholt and K. M. Langner, J. Comput. Chem., 2008, 29, 839–845 CrossRef PubMed.
Footnote |
† Electronic supplementary information (ESI) available. See DOI: 10.1039/c5ra16301c |
|
This journal is © The Royal Society of Chemistry 2015 |