DOI:
10.1039/C5RA16080D
(Paper)
RSC Adv., 2015,
5, 75711-75721
Effect of reaction temperature on properties of carbon nanodots and their visible-light photocatalytic degradation of tetracyline
Received
10th August 2015
, Accepted 1st September 2015
First published on 1st September 2015
Abstract
Water-soluble carbon nanodots (CNDt) with diverse sizes, crystal structures, surface properties, and characteristic fluorescence spectra were synthesized by the hydrothermal carbonization of larch at different temperatures. The effects of reaction temperature on the diameter distribution, structural components, and fluorescence properties of the CNDt were investigated systematically. The synthesized CNDt were found to be monodispersed spherical polymer nanodots with a low degree of aromatization and abundant oxygen-containing groups on their surface. Increasing the reaction temperature decreased the average size of the nanodots from 20.35 to 6.48 nm, while their quantum yield increased from 13% to 18%. Broader and weaker UV characteristic peaks were detected when the reaction temperature was increased from 200 to 260 °C. All the CNDt samples exhibited excitation and emission-independent properties, and obvious blue shift of the excitation and emission peaks occurred at higher reaction temperatures owing to the smaller size and different surface properties obtained. The CNDt were used as a photosensitizer in a CND/TiO2 system to effectively degrade tetracycline hydrochloride (TCH) under visible-light irradiation. The obvious blue shift exhibited by the smaller CNDt allowed the light to be fully used by the TiO2, resulting in nearly 100% TCH degradation for CND260/TiO2.
1. Introduction
Antibiotics are used extensively worldwide in medical treatment and agriculture but are often poorly absorbed by humans and animals; 50% to 80% of all antibiotics drain out and enter the aquatic environment. These residues may induce soil toxicity, development of antibiotic-resistant pathogens, and other problems that may threaten human health.1,2 For example, tetracyclines are the second most common antibiotics in both production and usage, and have been successfully used for treating various infectious diseases. However, there is still a lack of effective, complete, and systematic technologies for the removal of pollutants like tetracyclines. Compared with other methods such as adsorption and microbial degradation, photocatalytic degradation is a simple and effective way of removing such macromolecular organic compounds in aqueous systems.3,4 Photocatalysts can degrade macromolecules into non-toxic small molecules, inhibiting their toxic properties and restricting their transport in water thoroughly. Traditional semiconductors such as TiO2 and SiO2 are widely used for the photocatalytic removal of environmental pollution because they are good oxidants with high photocatalytic activity, chemical stability, and low toxicity. However, they can only effectively use a narrow spectral range of UV irradiation, which comprises 5% of incident sunlight.
The preparation and applications of quantum dots have attracted much attention because their quantum effects can give them unique properties. When particles are nanoscale, the size limit domain can give rise to size, quantum confinement, macroscopic quantum tunneling, and surface effects, resulting in chemical and physical properties different from those of macroscopic materials. Quantum dots have been widely used in the fields of bio-imaging, photocatalysis, medicine, and functional materials.5–8 The emerging carbon nanodots successfully overcome the drawbacks of traditional semiconductor and metal quantum dots such as poor water-solubility, high toxicity, and incompatibility with biological environments, opening up new directions and broadening the scope in which quantum dots may be used. Carbon nanodots are a new kind of nanoscale particles consisting of carbon existing in the form of a conjugated system of SP2 hybridized and unsaturated SP3 hybridized carbon atoms, which creates obvious responses to light, electricity and magnetism.9,10 Therefore, the search for a simple and environmentally friendly method of preparing carbon nanodots has become a hot topic.
The northeast of China is rich in larch resources, and a huge amount of sawdust waste is produced and discarded every year. The exploitation of such waste plays a significant role in the development of renewable energy sources.11,12 Hydrothermal carbonization has been proved to be an effective way to convert biomass into useful functional carbon materials. Through this process, biomass can be hydrolyzed to generate various value-added polymer precursors from which carbon materials with controllable shapes and sizes can be obtained.13,14 Carbon nanodots with ordered crystal lattices and frequency up-conversion properties have been successfully prepared via hydrothermal carbonization of biomass.15 Our groups have previously synthesized water-soluble carbon nanodots with substantial fluorescence via hydrothermal carbonization of pentosan extracted from pulp refining, which exhibited good photocatalytic properties under visible light.16
Here, we report the synthesis of water-soluble carbon nanodots with diverse sizes, crystal structures, and optical properties, using larch as the carbon source. The particle size, quantum yield, and characteristic fluorescent peaks of the nanodots were controlled by adjusting the reaction temperature. The resulting CNDt were systematically investigated as a photosensitizer in a TiO2-based photocatalytic system for the degradation of tetracycline hydrochloride.
2. Materials and methods
2.1 Hydrothermal carbonization of larch to produce CNDt
In a typical experiment, larch biomass (1.5 g) was dispersed in distilled water (40 mL) and ultrasonically agitated for 15 min. The suspension was added to a stainless steel autoclave of 55 mL in volume. The autoclave was placed in a furnace at 200 °C, 220 °C, 240 °C, and 260 °C for 12 h, and then allowed to cool to room temperature. The resulting brown suspension was collected and subjected to centrifugation at 12
000 rpm for 20 min. The light yellow supernatant containing the CNDt was subjected to dialysis (1000 Da molecular weight cutoff) for about 72 h before further characterization.
2.2 Preparation of the CND/TiO2 system
CND/TiO2 composites were synthesized using a sol–gel method.17 Tetrabutyl titanate (0.5 mL) was dissolved in ethanol (20 mL) to form aqueous solution A, while water (9 mL) and aqueous ammonia (5 mL, 28%) were mixed to form aqueous solution B. Solution B was added to A in a dropwise manner with continual stirring and the mixture was left to age for 12 h. The resulting precipitate was collected by centrifugation, dried at 80 °C for 6 h, and then calcined at 500 °C for 1 h to yield TiO2. The obtained TiO2 (0.1 g) was added to the CNDt solution (5 mL) with stirring over 10 min, followed by drying before further use.
2.3 Characterization
Transmission electron microscopy (TEM) images of the CNDt samples were obtained on a JEOL 2011 (FEI, Holland) operated at 200 kV. FT-IR spectra were recorded on a Fourier transform infrared (FT-IR) instrument (Perkin Elmer TV1900, Waltham, MA, USA) from 400–4000 cm−1 at a resolution of 4 cm−1. X-ray photoelectron spectra were recorded on a Physical Electronics PHI 5400 spectrometer (Physical Electronics, USA) using Al-Kα radiation (hν = 1486.6 eV). Binding energies were referenced to the C1s line at 284.6 eV. Elemental analysis (C, H, O, and N) was carried out using an EA 3000 analyzer (EuroVector, Milan, Italy). UV-vis absorption spectra were obtained using a TU-1900 UV-vis spectrometer. Fluorescence spectroscopy was performed on a LS-55 fluorescence spectrophotometer (PerkinElmer, USA) equipped with a 120 W xenon lamp as the excitation source. The morphologies and sizes of the CND/TiO2 composites were observed using environmental scanning electron microscopy (SEM; Quanta 200, FEI, Hillsboro, OR, USA).
2.4 Photocatalytic activity and quantum yield test
Photocatalytic activity tests were carried out in a cylindrical quartz photoreactor (275 mL) using TCH as the model compound and a 380 W Xe lamp (510 nm, Shanghai Hualun Lamp Factory, China) positioned inside the reactor as the light source. The photocatalyst powder (0.1 g) was added to 100 mL aqueous solution (50 mg L−1). Before the photocatalytic degradation, the suspension was magnetically stirred in the dark for 20 min to establish TCH adsorption/desorption equilibrium. Aliquots of 5 mL were collected from the suspension and filtrated using a 0.22 μm filter membrane. The concentration of TCH in the reaction solution after illumination was determined spectrophotometrically using a TU-1900 UV-vis spectrometer at 357 nm.
The fluorescence quantum yields of the CNDt were calculated using 5 μg mL−1 Rhodamine B in ethanol as a standard (quantum yield 90%). The absorbance of Rhodamine B at 543 nm was less than 0.05. The calculation was performed according to the following equation: Φu = Φs (Yu/Ys)(As/Au), where u and s represent the CNDt and Rhodamine B, respectively, Φ is the quantum yield, and Y is the integrated fluorescence area. A is the absorption at fluorescence excitation.
3. Results and discussion
3.1 Morphology of the CNDt
Changing the reaction temperature had a direct effect on the average diameter of the obtained CNDt, as shown in Table 1. TEM images and histograms of the size distribution of CND200 and CND260 are presented in Fig. 1, and clearly show that the CNDt were well dispersed spherical particles with different ordered lattice structures. CND200 exhibited a mean diameter of 20.35 nm (100 particles measured), and an obvious stripe-like hexagonal structure was detected on the surface. For CND260, the average diameter was only 6.48 nm, and an ordered cubic structure was clearly seen. These results suggest that increased reaction temperature led to a decrease in size and change in lattice structure of the CNDt. As the hydrothermal process mainly involved dehydration, polymerization, aromatization and carbonization,18 various water-soluble organic products were also produced in the HTC process, including sugars and organic acids, maximum sugar and organic acids concentrations were obtained under low-temperature conditions but degraded considerably at higher temperatures,19 besides, higher reaction temperature favor carbonization,20 which means reaction was more completely at higher temperature with more condensed carbon structure, leading to the size of carbon dots decreased as temperature increased.
Table 1 Average diameters and XPS analysis (wt%) of CNDt
Sample |
Average diameter (nm) |
C (wt%) |
O (wt%) |
N (wt%) |
Others (wt%) |
O/C (%) |
CND200 |
20.35 |
77.31 |
20.17 |
0.21 |
2.31 |
26.09 |
CND220 |
16.4 |
78.35 |
19.80 |
0.16 |
1.69 |
25.22 |
CND240 |
11.9 |
77.92 |
20.37 |
0.41 |
1.30 |
26.14 |
CND260 |
6.48 |
72.38 |
25.85 |
0.27 |
1.50 |
35.71 |
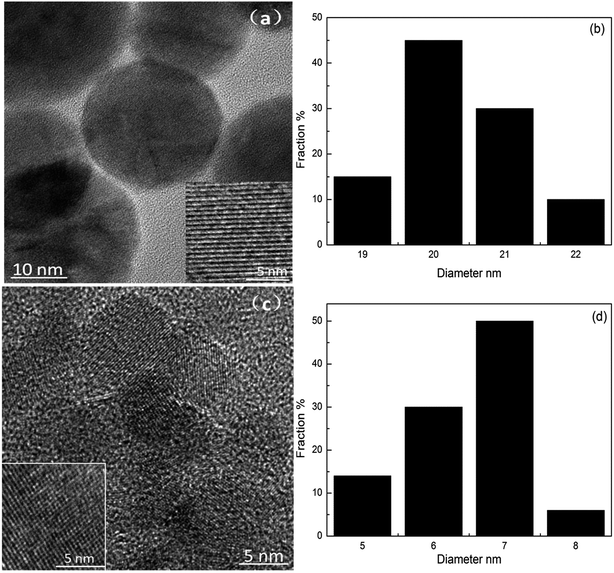 |
| Fig. 1 TEM images and corresponding diameter distributions of CND200 (a and c) and CND260 (b and d). | |
3.2 Surface properties of the CNDt
FT-IR analysis (Fig. 2(a)) was used to reveal the functional groups on the surface of the raw material and CNDt samples. Larch wood is predominantly composed of cellulose, hemicelluloses, and lignin, which have large amounts of oxygen-containing surface groups such as hydroxyls, carbonyls, and carboxyls. The FT-IR peaks observed for CND200 were similar to those of the raw materials, while the CND220, CND240 and CND260 samples showed peaks with obvious changes, in accordance with previous studies showing that 200 °C is too low for the wood structure to be broken down completely.
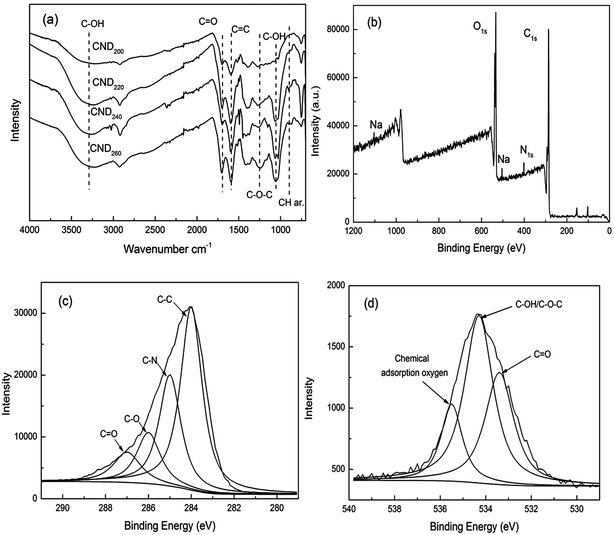 |
| Fig. 2 FT-IR spectra (a) and XPS survey spectrum (b), high-resolution C1s peaks and the fitting curves (c) and high-resolution O1s peaks and fitting curves (d). | |
The bands observed at 3300–3500 cm−1 and 1028 cm−1 were assigned to C–OH groups. Compared with those observed for the raw materials, the intensity of these peaks was much lower for the samples prepared at high temperature, because such groups are easily degraded to intermediate products during hydrothermal carbonization. The new peaks appearing at 1000–1460 cm−1 corresponded to the C–O stretching vibration, confirming that hydroxyl, ester, and ether groups had been generated.21 The new peaks at 1625 and 875–750 cm−1 were attributed to the stretching vibration of C
C and the out-of-plane bending vibration of aromatic C–H, respectively, confirming the formation of a large amount of aromatic structure.22 Finally, the adsorption band at 1700 cm−1 corresponded to carboxylate groups. These results indicate that the structure of the larch wood had been completely destroyed to form carbon material containing aromatic structures rich in surface functional groups including hydroxyls, esters, ethers, carbonyls, and carboxylic groups.
The elemental compositions (measured by XPS) of the surface of the raw materials and CNDt samples are summarized in Table 1. The samples mainly contained C, O, and N elements, with trace amounts of Na, Si, Ca, and S. When the reaction temperature was increased from 200 °C to 260 °C, the content of C on the surface declined from 77.3% to 72.4%, while the O content increased from 20.2% to 25.9%. This further confirmed that the CNDt were condensed carbon materials with a large amount of oxygen-containing groups. In detail, the ratio of O and C exhibited an obvious rising trend with reaction temperature, different from that observed for previously obtained solid products.20 This was caused by the increased number of molecules available for polymerization, confirming that the structure of the CNDt samples may have been different from those of CSs. The present CNDt may have been polymer nanodots rich in oxygen-containing groups with a low degree of aromatization.23
XPS was conducted to analyze the chemical composition of CND260. C1s, O1s, N1s, and Na peaks were observed, as shown in Fig. 2(b). The XPS results indicated that these nanodots were mainly composed of C, O, and N, as well as a limited amount of Na element. The high C and O content confirmed that a condensed material with abundant oxygen-containing groups was produced at 260 °C. The C1s spectrum was fit to four curves and attributed to aliphatic groups C–C (283.9 eV), C–N (285.2), C–O (286.1), and C
O/C
N (287.3).18,22,24 The O1s spectrum exhibited three peaks at 533.4, 534.3, and 535.5 eV, which were attributed to O–C
O, C–OH/C–O–C groups, and chemically adsorbed oxygen, respectively.23 These results indicate the presence of an aromatic structure and abundant oxygen-containing groups on the surface of CND260.
3.3 Optical properties of the CNDt
UV-vis and PL spectra were measured to investigate the optical properties of the CNDt samples. All obtained samples were water-soluble and formed light yellow aqueous solutions. The UV-vis absorption spectra exhibited a high and obvious peak around 281 nm, as shown in Fig. 3. This can be attributed to complex electron transition on the surface, the π conjugated aromatic system, and the n–π* transition of the carbonyl and other oxygen-containing groups.25,26 It can be clearly seen that increased reaction temperatures led to the characteristic peak becoming broader and weaker, as the conjugate system is to reduce the total energy and increase the stability,27 the structure of the CNDt prepared at low temperature was incomplete with lower stability, hence causing the conjugate peak to be more obvious. This result is consistent with the FT-IR analysis.
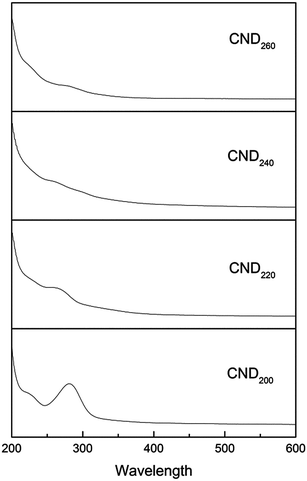 |
| Fig. 3 UV-vis absorption spectrum of CNDt samples. | |
The emission spectrum of CND200 at around 400–600 nm exhibited a peak at 440 nm upon excitation at 350 nm. A corresponding peak centered at 350 nm in the excitation spectrum appeared at an emission wavelength of 440 nm. With increasing hydrothermal temperature, both the excitation and emission peaks exhibited blue shift to lower wavelength, as shown in Fig. 4 and Table 2. It is well known that the PL properties of a material can be attributed to energy-level transition, surface energy traps, and the radiative recombination of electrons and holes,28 as well as interactions between electrons and holes and the environment around them. Additionally, polycyclic aromatic compounds within the CNDs may have been responsible for their excellent PL properties.29
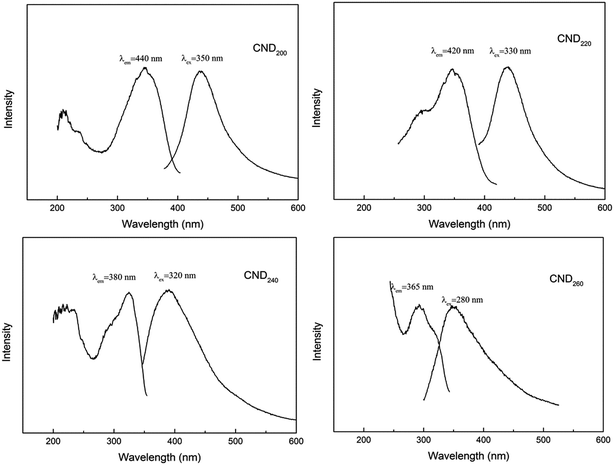 |
| Fig. 4 PL emission and excitation spectra of CNDt samples. | |
Table 2 Characteristic parameters of CNDt
Samples |
Average diameter (nm) |
Quantum yield (%) |
Characteristics excitation wavelength (nm) |
Characteristics emission wavelength (nm) |
Photocatalytic degradation efficiency as photosensitizer (%) |
CND200 |
20.35 |
13.4 |
350 |
440 |
89.7 |
CND220 |
16.4 |
14.9 |
330 |
420 |
93.6 |
CND240 |
11.9 |
16.6 |
320 |
380 |
95.9 |
CND260 |
6.48 |
18.1 |
280 |
365 |
99.0 |
Size is an important factor that affects the excitation and emission wavelengths, and can give a material special characteristics.30 Furthermore, changes in structural and surface properties such as the degree of aromatization and amount of oxygen-containing groups can cause changes in the energy-level difference between the first excited state and ground states, leading to changes in the energy of absorbed and emitted photons, showing as obvious blue shift.31
The PL spectra also indicated that the maximum emission and its intensity had a direct relationship with the excitation wavelength; this is a PL characteristic of C dots.32 For CND200, upon changing the excitation wavelength from 260 to 420 nm, the emission peak red-shifted from 428 to 493 nm with a gradual decrease in intensity. Therefore, visible emission from CND200 over the blue-to-red wavelength range was obtainable using the same sample under UV and visible excitation. For samples prepared at different temperatures, the excitation wavelength exhibited no obvious shift, while the main peak of the emission spectrum shifted from 440 nm to around 360 nm, in accordance with the analysis above (Fig. 5).
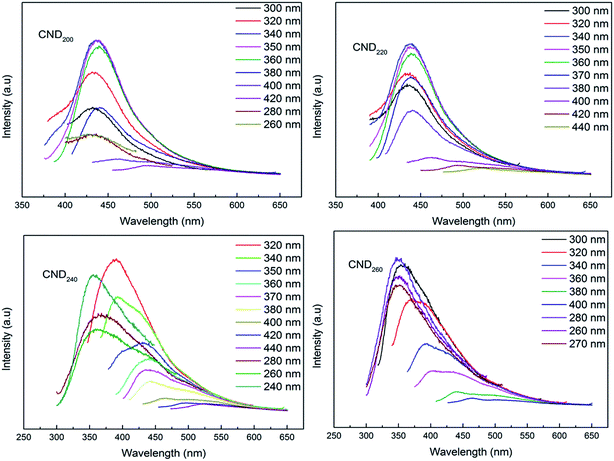 |
| Fig. 5 Emission spectra of CNDt at different excitation wavelengths. | |
Using Rhodamine B in ethanol as a standard, the quantum yield of CND200 was calculated to be about 13.4%, higher than that of most reported CNDs.23,28 The quantum yield increased obviously to 18.1% for CND260, confirming that increasing the reaction temperature caused an increase in quantum yield (as shown in Table 2).
3.4 pH sensitivity and chemical stability tests
Digital photos and emission spectra of CND220 at different pH, adjusted using a sodium bicarbonate and sodium carbonate buffer solution, are shown in Fig. 6. All prepared samples (CND200, CND220, CND240, and CND260) exhibited color ranging from light yellow to dark yellow as the pH was varied from 4.0 to 10.0. The intensity of the emission peak decreased with increasing pH without a change in wavelength, as previously reported.33 The abundant oxygen-containing groups on the surface imparted acid properties to the CNDs, so at high pH values an increasing negative charge density accumulated on the surface. This influenced the π–π* and n–π* electronic transitions, leading to the observed color changes and fluorescence quenching.
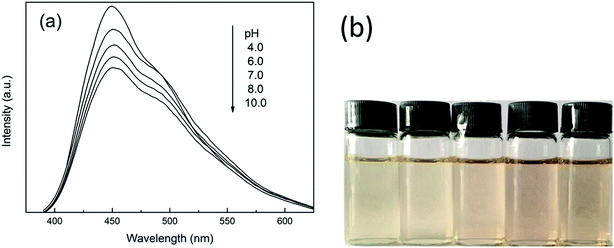 |
| Fig. 6 PL spectra of CND220 excited at 320 nm in solutions of different pH. | |
To investigate the chemical stability, FL quantum yields (QY) and optical absorption intensity (OAI) of CNDt synthesized for six weeks were tested as shown in Table 3. They all exhibit good stability on both quantum yields (QY) and optical absorption intensity even samples lasted for six weeks. Both the QY and fluorescence intensity keep almost constant, confirming the chemical stability of obtained CNDt.
Table 3 Quantum yields and optical absorption intensity parameters of CNDt synthesized for six weeksa
|
QY (%) |
QYa (%) |
OAI (a.u.) |
OAIa (a.u.) |
QY = sample quantum yield, QYa = quantum yield of samples synthesized for six weeks, OAI = optical absorption intensity, OAIa = optical absorption intensity of samples synthesized for six weeks, optical absorption intensity was tested via irradiation by their respective characteristic excitation wavelength. |
CND200 |
13.4 |
13.0 |
673.5 |
642.1 |
CND220 |
14.9 |
14.5 |
685.2 |
650.9 |
CND240 |
16.6 |
16.1 |
692.9 |
658.3 |
CND260 |
18.1 |
17.2 |
693.4 |
662.1 |
The photochemical was tested by fluorescence intensity vs. time of carbon nanodots under continuous UV irradiation (using UV lamp with power of 30 W, wavelength of 254 nm, light intensity of 107 μW cm−2), the results show that under constant UV illumination for 5 h, the fluorescence intensity remained constant, almost no bleaching was observed, suggesting the emission was quite stable (Fig. 7).
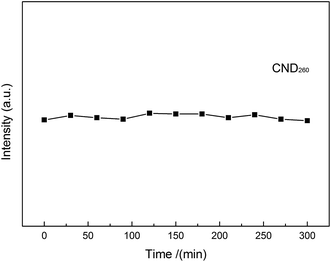 |
| Fig. 7 Photochemical stability of CND260 under continuous UV irradiation. | |
The decay curve of synthesized CNDt were measured by HORIBA Fluoromax-4, from Fig. 8 it can be clearly seen the decay curve of CND260 can be very well fitted to triple-exponential function, the lifetimes are τ1 = 8.34, τ2 = 2.59 and τ3 = 1.26 ns, and the mean lifetime is calculated to be 5.48 ns, which is longer than previous reports;34 based on the same calculation method, the life time was 3.23 ns, 4.32 ns, 5.06 ns and 5.48 ns for CND200, CND220, CND240, and CND260 respectively.
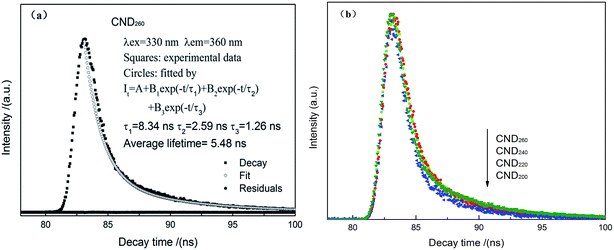 |
| Fig. 8 Fluorescence decay curve and the fitting curve of CND260 (a) decay curve comparison of CNDt. | |
3.5 Photocatalytic activity of CND/TiO2
The CNDt samples investigated here were rich in oxygen-containing groups and have been proved to be easily attached in a stable manner to the surface of TiO2.35 The CND were combined with TiO2 in a composite system, as shown in Fig. 9(a) and (b). The corresponding HRTEM image shows that CND260 (lattice spacing 0.22 nm) were in close contact with the anatase TiO2 nanoparticles with the (101) crystal plane exposed (lattice spacing 0.35 nm), confirming that CNDt were successfully attached to the surface of the TiO2.
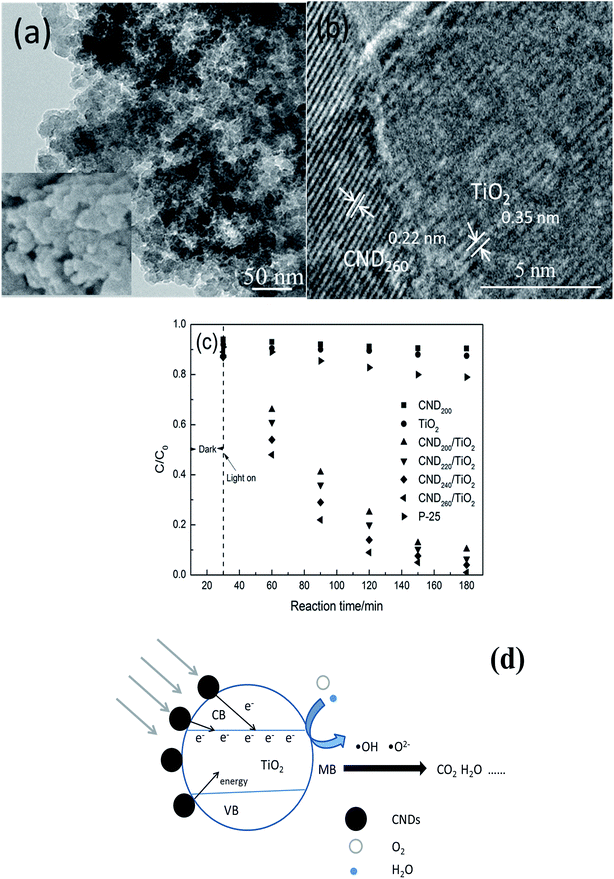 |
| Fig. 9 (a) and (b) SEM and corresponding high-resolution TEM images of CND/TiO2 composite, (c) TCH degradation percentage vs. time curves and (d) catalytic mechanism for CND/TiO2 under visible-light irradiation. | |
The visible-light catalytic activities of the prepared CNDt/TiO2 composites were evaluated using the decomposition of tetracycline hydrochloride (TCH) under visible-light irradiation, as shown in Fig. 9(c). Only 9.6% and 12.5% TCH reduction were obtained when pure CND200 and TiO2 were used as catalysts, respectively, and were mainly because of adsorption. Although the photocatalytic efficiency of P-25 was higher than pure CND200 and TiO2, the value was still low of only 21%. The CNDt/TiO2 composites all gave efficient catalysis with high TCH degradation, indicating that interaction between the CNDs and TiO2 occurred. The CND200/TiO2 composite exhibited a good photocatalytic activity and gave around 89.7% TCH degradation. The saturated adsorption value was low under the dark condition, confirming that the observed decrease in TCH concentration mainly derived from photocatalytic degradation. The degradation efficiency reached 93.6%, 95.9% and 99.0% for CND220/TiO2, CND240/TiO2 and CND260/TiO2, respectively, as shown in Table 2. Nearly 100% degradation of TCH was obtained for CND260/TiO2.
The proposed degradation mechanism is as follows (Fig. 9(d)): the CNDt with abundant oxygen-containing groups are sensitizer, when irradiation by visible light they can act as a photosensitizer and absorb photons. Photons with adequate energy can excite electrons in the HOMO, thus transferring them to the LUMO of the CNDt. Free electrons as well energy can then be transferred to the conduction band of TiO2.36 When the energy is equal or greater than the band-gap energy of TiO2, conduction band electrons (e−) and valence band holes (h+) are generated. In this system, photogenerated holes could either recombine with electrons, react with OH− or H2O, oxidizing them to highly oxidizing species such as OH˙ and O2˙ radicals, or oxidize the adsorbed TCH molecules.6 Such highly oxidizing species were responsible for the decomposition of TCH by TiO2. Additionally, the photogenerated electrons could reduce adsorbed TCH or react with electron acceptors such as O2 adsorbed on the TiO2 surface or dissolved in water, reducing them to super-oxide radical anion O2˙−, which can be transformed to OH˙ radicals by further reactions. In neutral conditions, reversible isomerization of asymmetric carbon atoms can occur, forming epimers of TCH. These epimers and their dehydration products are the main degradation products.37
As discussed above, higher synthesis temperature led to changes in the size, structure, surface properties of the CNDt as well the amount of oxygen-containing groups on their surface.21 These changes can cause changes in the energy-level difference between the first excited state and ground states, leading to changes in the energy of absorbed and emitted photons, showing as obvious blue shift of emission and excitation spectra,30 CND260 exhibited smallest size with most abundant oxygen-containing groups, have strongest response to light irradiation, hence, CND260/TiO2 exhibited the highest photocatalytic activity.
4. Conclusion
Water-soluble carbon nano dots (CNDt) were synthesized via one-pot simple hydrothermal carbonization of lignocellulose-larch at different temperatures. The as-prepared CNDt were nanoscale spherical polymers with a low degree of aromatization and abundant oxygen-containing groups. They exhibited excellent photoluminescence (PL), excitation-dependence, and pH sensitivity, and their size, crystal structure, surface properties, and fluorescence characteristics could be varied by changing the reaction temperature from 200 °C to 260 °C. Increasing the reaction temperature decreased the average size and increased the fluorescence quantum yield of the CNDt, and was accompanied by variation in their ordered lattice structure from an obvious stripe-like hexagonal structure to a cubic structure. An obvious blue shift of the excitation and emission peaks was also observed, which arose from the differences in size and surface properties.
A CND/TiO2 system was found to be an effective photocatalyst; CNDt loaded on the surface of TiO2 acted as a photosensitizer. The spectral response of the composite system could hence be widened from the UV region to the visible-light region. Effective degradation of tetracycline hydrochloride (TCH) was achieved under visible-light irradiation. CNDt with smaller size, higher quantum yield and shorter excitation characteristic peak wavelength were found to be more beneficial as a photosensitizer in the CND/TiO2 system; nearly 100% TCH degradation was obtained for CND260/TiO2.
Acknowledgements
This work was financially supported by grants from Special Fund for Forest Scientific Research in the Public Welfare (201504605), the Fundamental Research Funds of the Central Universities (2572014EB01), the National Natural Science Foundation of China (No. 31570567, 31500467), and Zhejiang Key level 1 Discipline of Forestry Engineering (2014lygcz017).
References
- B. Halling-Sørensen, S. Nors-Nielsen, P. F. Lanzky, F. Ingerslev, H. C. Holten- Lutzhøft and S. E. Jørgensen, Occurrence, fate, and effects of pharmaceutical substances in the environment. A review, Chemosphere, 1998, 36, 357–393 CrossRef.
- A. Pruden, R. Pei, H. Storteboom and K. H. Carlson, Antibiotic resistance genes as emerging contaminants: Studies in Northern Colorado, Environ. Sci. Technol., 2006, 40, 7445–7450 CrossRef CAS.
- Y. B. Liu, X. J. Gan, B. X. Zhou, B. T. Xiong, J. H. Li, C. P. Dong, J. Bai and W. M. Cai, Photoelectrocatalytic degradation of tetracycline by highly effective TiO2 nanopore arrays electrode, J. Hazard. Mater., 2009, 171, 678–683 CrossRef CAS PubMed.
- C. Zhao, Y. Zhou, D. J. de Ridder, J. Zhai, Y. M. Wei and H. P. Deng, Advantages of TiO2/5A composite for photocatalytic degradation of antibiotic oxytetracycline in aqueous solution: Comparison between TiO2 and TiO2/5A composite system, Chem. Eng. J., 2014, 248, 280–289 CrossRef CAS PubMed.
- Q. H. Liang, W. J. Ma, Y. Shi, Z. Li and X. M. Yang, Easy synthesis of highly fluorescent carbon quantum dots from gelatin and their luminescent properties and applications, Carbon, 2013, 60, 421–428 CrossRef CAS PubMed.
- H. T. Li, X. D. He, Z. H. Kang, H. Huang, Y. Liu, J. L. Liu, S. Y. Lian, C. H. A. Tsang, X. B. Yang and S. T. Lee, Water-soluble fluorescent carbon quantum dots and photocatalyst design, Angew. Chem., Int. Ed., 2010, 49, 4430–4434 CrossRef CAS PubMed.
- L. Cao, S. Sahu, P. Anilkumar, C. E. Bunker, J. Xu, K. A. S. Fernando, P. Wang, E. A. Guliants, K. N. Tackett and Y. P. Sun, Carbon nanoparticles as visible-light photocatalysts for efficient CO2 conversion and beyond, J. Am. Chem. Soc., 2011, 133, 4754–4757 CrossRef CAS PubMed.
- X. Zhang, H. Ming, R. Liu, X. Han, Z. Kang, Y. Liu and yonglai Zhang, Highly sensitive humidity sensing properties of carbon quantum dots films, Mater. Res. Bull., 2013, 48, 790–794 CrossRef CAS PubMed.
- X. H. Wang, K. G. Qu, B. L. Xu, J. S. Ren and X. G. Qu, Multicolor luminescent carbon nanoparticles: Synthesis, supramolecular assembly with porphyrin, intrinsic peroxidase-like catalytic activity and applications, Nano Research, 2011, 4, 908–920 CrossRef CAS.
- J. H. Shen, Y. H. Zhu, X. L. Yang and C. Z. Li, Graphene quantum dots: emergent nanolights for bioimaging, sensors, catalysis and photovoltaic devices, Chem. Commun., 2012, 48, 3686–3699 RSC.
- L. Zhao, L. Z. Fan, M. Q. Zhou, H. Guan, S. Y. Qiao, M. Antonietti and M.-M. Titirici, Nitrogen-containing hydrothermal carbons with superior performance in supercapacitors, Adv. Mater., 2010, 22, 5202–5206 CrossRef CAS PubMed.
- L. P. Xiao, Z. J. Shi, F. Xu and R. C. Sun, Hydrothermal carbonization of lignocellulosic biomass, Bioresour. Technol., 2012, 118, 619–623 CrossRef CAS PubMed.
- Q. Wu, W. Li, J. Tan and S. X. Liu, Flexible cage-like carbon spheres with ordered mesoporous structures prepared via a soft-template/hydrothermal process from carboxymethylcellulose, RSC Adv., 2014, 4, 61518–61524 RSC.
- G. B. Barin, I. F. Gimenez, L. P. Costa, A. G. S. Filho and L. S. Barreto, Hollow carbon nanostructures obtained from hydrothermal carbonization of lignocellulose biomass, J. Mater. Sci., 2014, 49, 665–672 CrossRef CAS.
- X. D. He, H. T. Li, Y. Liu, H. Huang, Z. H. Kang and S.-T. Lee, Water soluble carbon nanoparticles:
hydrothermal synthesis and excellent photoluminescence properties, Colloids Surf., B, 2011, 87, 326–332 CrossRef CAS PubMed.
- Q. Wu, W. Li, Y. J. Wu, Z. H. Huang and S. X. Liu, Pentosan-derived water-soluble carbon nano dots with substantial fluorescence: properties and application as a photosensitizer, Appl. Surf. Sci., 2014, 315, 66–72 CrossRef CAS PubMed.
- Q. Wu, W. Li, T. Tan, Y. J. Wu and S. X. Liu, Hydrothermal carbonization of carboxymethylcellulose: one-pot preparation of conductive carbon microspheres and water-soluble fluorescent carbon nanodots, Chem. Eng. J., 2015, 266, 112–120 CrossRef CAS PubMed.
- M. Sevilla and A. B. Fuertes, The production of carbon materials by hydrothermal carbonization of cellulose, Carbon, 2009, 47, 2281–2289 CrossRef CAS PubMed.
- S. K. Hoekman, A. Broch, C. Robbins, B. Zielinska and L. Felix, Hydrothermal carbonization (HTC) of selected woody and herbaceous biomass feedstocks, Biomass Convers. Biorefin., 2013, 3, 113–126 CrossRef CAS.
- M. Li, W. Li and S. X. Liu, Control of the morphology and chemical properties of carbon spheres prepared from glucose by a hydrothermal method, J. Mater. Res., 2012, 27, 1117–1123 CrossRef CAS.
- X. M. Sun and Y. D. Li, Colloidal carbon spheres and their core/shell structures with noble-metal nanoparticles, Angew. Chem., Int. Ed., 2004, 43, 597–601 CrossRef PubMed.
- S. X. Liu, J. Sun and Z. H. Huang, Carbon spheres/activated carbon composite materials with high Cr(VI) adsorption capacity prepared by a hysrothermal method, J. Hazard. Mater., 2010, 173, 377–383 CrossRef CAS PubMed.
- S. Liu, J. Q. Tian, L. Wang, Y. W. Zhang, X. Y. Qin, Y. L. Luo, A. M. Asiri, A. O. Al-youbi and X. P. Sun, Hydrothermal treatment of grass: a low-cost, green route to nitrogen-doped carbon-rich, photoluminescent polymer nanodots as an effective fluorescent sensing platform for label-free detection of Cu (II) ions, Adv. Mater., 2012, 24, 2037–2041 CrossRef CAS PubMed.
- C. C. Huang, H. S. Li and C. H. Chen, Effect of surface acidic oxides of activated carbon on adsorption of ammonia, J. Hazard. Mater., 2008, 159, 523–527 CrossRef CAS PubMed.
- X. F. Jia, J. Li and E. K. Wang, One-pot green synthesis of optically pH-sensitive carbon dots with upconversion luminescence, Nanoscale, 2010, 4, 5572–5575 RSC.
- C. Z. Zhu, J. F. Zhai and S. J. Dong, Bifunctional fluorescent carbon nanodots: green synthesis via soy milk and application as metal-free electrocatalysts for oxygen reduction, Chem. Commun., 2012, 48, 9367–9369 RSC.
- H. T. Li, Z. H. Kang, Y. Liu and S.-T. Lee, Carbon Nanodots: Synthesis, Properties and Applications, J. Mater. Chem., 2012, 22, 24230–24253 RSC.
- P. Anilkumar, L. Cao, J. J. Yu, K. N. Tackett, P. Wang, M. J. Meziani and Y. P. Sun, Crosslinked carbon dots as ultra-bright fluorescence probes, Small, 2013, 9, 545–551 CrossRef CAS PubMed.
- A. B. Bourlinos, A. Stassinopoulos, D. Anglos, R. Zboril, M. Karakassides and E. P. Giannelis, Surface functionalized carbogenic quantum dots, Small, 2008, 4, 455–458 CrossRef CAS PubMed.
- S. Liu, J. Q. Tian, L. Wang, Y. W. Zhang, X. Y. Qin, Y. L. Luo, A. M. Asiri, A. O. Al-youbi and X. P. Sun, Hydrothermal treatment of grass: a low-cost, green route to nitrogen-doped carbon-rich, photoluminescent polymer nanodots as an effective fluorescent sensing platform for label-free detection of Cu (II) ions, Adv. Mater., 2012, 24, 2037–2041 CrossRef CAS PubMed.
- S. N. Baker and G. A. Baker, Luminescent carbon nanodots: Emergent nanolights, Angew. Chem., Int. Ed., 2010, 49, 6726–6744 CrossRef CAS PubMed.
- Q. H. Liang, W. J. Ma, Y. Shi, Z. Li and X. M. Yang, Easy synthesis of highly fluorescent carbon quantum dots from gelatin and their luminescent properties and applications, Carbon, 2013, 46, 421–428 CrossRef PubMed.
- Y. M. Guo, Z. Wang, H. W. Shao and X. Y. Jiang, Hydrothermal synthesis of highly fluorescent carbon nanoparticals from sodium citrate and their use for the detection of mercury ions, Carbon, 2013, 52, 583–589 CrossRef CAS PubMed.
- Q. L. Wang, H. Z. Zheng, Y. J. Long, L. Y. Zhang, M. Gao and W. J. Bai, Microwave-hydrothermal synthesis of fliorescent carbon dots from graphite oxide, Carbon, 2011, 49, 3134–3140 CrossRef CAS PubMed.
- F. Wang, Y. Zhang, Y. Liu, X. Wang, M. Shen, S.-T. Lee and Z. Kang, Opto-electronic conversion logic behavior through dynamic modulation of electron/energy transfer states at the TiO2-carbon quantum dot interface, Nanoscale, 2013, 2, 1831–1835 RSC.
- S. Nishimura, N. Abrams, B. A. Lewis, L. I. Halaoui, T. E. Mallouk, K. D. Benkstein, J. van de Lagemaat and A. J. Frank, Standing wave enhancement of red absorbance and photocurrent in dyr-sensitized titanium dioxide photoelectrodes coupled to photonic crystals, J. Am. Chem. Soc., 2003, 125, 6306–6310 CrossRef CAS PubMed.
- M. E. Lindesey, M. Meyer and E. M. Thurman, Analysis of Trace level of sulfonamide and tetracycline antimicrobials on groundwater and surface water using solid-phase extraction and liquid chromatography/mass spectrometry, Anal. Chem., 2001, 73, 4640–4646 CrossRef.
|
This journal is © The Royal Society of Chemistry 2015 |