DOI:
10.1039/C5RA15860E
(Paper)
RSC Adv., 2015,
5, 79635-79643
Influence of electronic vs. steric factors on the solid-state photochromic performances of new polyoxometalate/spirooxazine and spiropyran hybrid materials†
Received
7th August 2015
, Accepted 14th September 2015
First published on 14th September 2015
Abstract
Two new photochromic hybrid organic–inorganic supramolecular materials (SN)2[W6O19] (SN2W6) and (SP)2[W6O19]·0.5CH3CN (SP2W6) have been elaborated by combining for the very first time an isopolyoxotungstate [W6O19]2− with both photoswitchable cationic spirooxazine (SN) and spiropyran (SP), via electrostatic interactions. (SN)2[Mo6O19] (SN2Mo6), a third new photochromic compound which contains the [Mo6O19]2− unit, has been also designed. Their structure was solved by single-crystal X-ray diffraction analysis, and their solid-state photochromic properties in ambient conditions were thoroughly investigated by UV-visible diffuse reflectance spectroscopy. SN2W6 and SN2Mo6 have been proved to be isostructural while SP2W6 is isostructural with its already reported counterpart SP2Mo6. In the SN2M6 and SP2M6 series, the SN and SP molecules are in very close contact with the POM units, which a priori should dramatically limit their photoisomerization in the solid state. However, this strongly varies with the nature of the metal in the [M6O19]2− unit. Indeed SN2W6 and SP2W6 exhibit remarkable photochromism under low-power UV excitation (λex = 365 nm) while SN2Mo6 and SP2Mo6 develop very limited color-change effects under similar UV exposure. These results have unambiguously highlighted that the efficiency of the SN and SP photoisomerizations in these hybrid systems is much more impacted by an electronic factor resulting from the intrinsic optical absorption properties of both SP and POM components than by a steric factor induced by crystal packing.
1. Introduction
In recent years, considerable efforts have been made to elaborate efficient solid-state photochromic materials that exhibit reversible light-induced switching between two optically distinguishable states.1 The ability to control their optical properties with light is of great interest in a wide range of technological and marketable applications, such as smart pigments for paints and windows,2 optical switches,3 and more recently, 3D high-density optical data storage.4 For all these listed applications, ideal materials should develop efficient photoactivity in the solid state and in ambient conditions (strong coloration contrast, optical bistability, fast photoswitch rates, high cyclability). Currently several organic molecules possess attractive photoswitchable properties but many do not meet all the criteria. For example, the classes of spiropyrans5 (SP) and spirooxazines6 (SN) have probably been the most widely studied in these last fifty years, because of their high optical performances and their synthetic accessibility. Such molecules absorb UV irradiation in the 350–400 nm range, and exhibit efficient ring-opening/ring-closure processes between a nonplanar colorless closed form and a coloured opened merocyanin (MC) form (Fig. 1a). MC exhibits a broad absorption band in the visible domain and can be converted back into the closed-ring form by visible-light irradiation or heating. These reversible photoisomerizations are generally highly efficient in solution or in crystalline state but only at very low temperature.7 Actually, in most cases, SP and SN solid-state photochromism is weak or totally annihilated in ambient conditions, which strongly limits the incorporation of such molecules into efficient photoadressable solid devices. Last decades, several innovative strategies were successfully managed to increase the photochromic activities of spiro-derivative molecules in the solid state. For example, the embedding of spiropyrans and spirooxazines into the cavities of porous organic polymers or sol–gel host matrixes leads to amorphous soft materials with strong photoresponses.8 The elaboration of crystallized hybrid systems is an alternative approach which, besides, allows the X-ray structural determination of the spiro-derivative/host matrix interface, opening the way to the establishment of fine structure/property relationships.
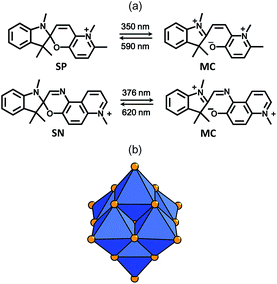 |
| Fig. 1 (a) Photoinduced equilibriums involving the cationic spiropyran (SP) and spirooxazine (SN). (b) Polyhedral representation of the [M6O19]2− (M = Mo, W) Lindqvist-type polyoxometalate (gold sphere: oxygen). | |
Recently, we evidenced that the coupling with polyoxometalates (POMs) constitutes an attractive opportunity to reach such crystallized hybrid materials, and to drastically improve the solid-state photochromic performances of spiro-derivatives at room temperature. POMs are described as soluble metal-oxides of early transition metals (usually W, Mo, V) in high oxidation states.9 The huge diversity of their compositions, structures, charges and sizes make them relevant for applications in numerous fields in optic, which include photochromism,10 electrochromism,11 NLO,12 and photoluminescence.13 Focusing on photochromism, it has been shown that the assembly of POMs with SP molecules can in some cases exalt the SP → MC photoconversion because of the strong stabilization of the zwitterionic MC form in polar POM frameworks. Noticeably, innovative bistable materials with remarkable photoresponses were obtained from spiropyrans and spirooxazines initially nonphotochromic in their crystalline state.14 Two new classes of hybrid materials were conceived from two complementary synthesis strategies. The covalent approach consisted in grafting via peptidic bonds one or two neutral SP molecules onto an Anderson-type {MnMo6O18} platform.15 The first multi-photochromic single molecule incorporating one SP and one SN fragments onto a POM core has been even successfully obtained.16 In a second ionic approach, supramolecular SP/POM assemblies were designed by associating a cationic spiropyran (SP) with different polyoxomolybdates via electrostatic interactions.17 Strikingly, the SP ⇆ MC photoconversion efficiency strongly varies in such ionic hybrid systems. For example, (SP)4[Mo8O26]·2CH3CN and (SP)3(NH4)[Mo8O26] are remarkably photochromic, while (SP)2[Mo6O19]·0.5CH3CN (SP2Mo6) exhibits a very limited UV-induced color-change effect.17a
Overall, the photochromic performances of the SP/POM assemblies are governed by both steric and electronic factors. The first one reflects the relative arrangement of the SP cations and the POM units in the frameworks, while the second one refers to the relative positioning of their absorption bands in wavelength space. For example, the weak photochromic performances of SP2Mo6 were assumed to result from a negative combination of these two factors. In contrast with best photochromic systems, the SP cations and the [Mo6O19]2− units are strongly linked via π–π stacking interactions. This specific arrangement dramatically limits the free volume around the SP molecules and should disadvantage their photoisomerization. In addition, the absorption bands of the [Mo6O19]2− anion strongly overlap those of the SP cation in the UV domain. Consequently, [Mo6O19]2− should dramatically absorb the UV excitation energy needed to activate the ring-opening process of the spiropyran.
To date, the influence of both electronic and steric factors on the photochromism efficiency of the SP/POM assemblies have not been uncorrelated because both factors vary in all reported materials. Herein, the main goal of the present work is to specifically investigate the effect of the electronic factor, everything else being equal. For a given topology, the absorption bands of the POMs vary in energy with the nature of the metal.18 Then, the substitution of Mo by W in the POM units appears as a very useful tool to tune the electronic factor without any structural change. Furthermore, the combination of spiropyrans and spirooxazines with isopolyoxotungstates using the ionic approach has never been explored so far. With this aim, we report the synthesis of a new supramolecular system (SP)2[W6O19]·0.5CH3CN (SP2W6) which is built upon the same SP cation (Fig. 1a) associated with the [W6O19]2− unit. Noticeably, SP2W6 is isostructural with SP2Mo6, what allows keeping constant the steric factor.
To extend our study to other spiro-derivatives, we have also designed two new isostructural hybrid systems (SN)2[W6O19] (SN2W6) and (SN)2[Mo6O19] (SN2Mo6) which combine for the very first time POMs units and a rare cationic spirooxazine (SN) derivative (Fig. 1a). The high photochromic performances of spirooxazines are well-recognized and have found applications in ophthalmology, being used for example in Orgavert™ sunglasses and in PHOTOLITE™ photochromic lenses.5a It has also been noticed that while the structure of SN and SP are close, their photochromic properties can differ both on a photogenerated hue and a coloration rate point of view.16 The structural characterizations of SP2W6, SN2W6, and SN2Mo6 are thus reported. Besides, their optical properties are widely discussed and compared with those of SP2Mo6. This study reveals that, in strong contrast with their Mo counterparts, SN2W6 and SP2W6 exhibit remarkable solid-state photochromism in ambient conditions.
2. Results and discussion
2.1 Synthesis and structural description
(SP)2[W6O19]·0.5CH3CN (SP2W6), (SN)2[W6O19] (SN2W6), and (SN)2[Mo6O19] (SN2Mo6) all contain the Lindqvist-type [M6O19]2− anion19 (M = Mo or W) (Fig. 1b) which is built upon six edge-shared [MO6] octahedra. Yellow-ochre powder of SP2W6 has been isolated from a method derived on the synthesis of (SP)2[Mo6O19]·0.5CH3CN (SP2Mo6),17a starting from (NBu4)2[W6O19] and two equivalents of SP(NO3) dissolved in acetonitrile. Orange single crystals have been also obtained using slow diffusion of ethanol into the filtrate. SN2W6 and SN2Mo6 have been synthesised starting from (NBu4)2[M6O19] and two equivalents of SN(NO3) dissolved in DMF. Orange-red single crystals of SN2W6 and red single crystals of SN2Mo6 have been directly obtained from the solutions using slow diffusion crystallization techniques. Elemental analyses, TGA/DSC measurements (Fig. S1, ESI†), and IR spectroscopy analyses (Fig. S2, ESI†) show that these two latter compounds are free from crystallized DMF molecules. Importantly, the coupling of the SP and SN molecules with the [M6O19]2− anion has a beneficial effect on the thermal resistance of the photoswitchable organic molecules. SN2W6 and SN2Mo6 decompose at 245 °C and 250 °C respectively, whereas SN(NO3) is thermally stable up to 190 °C only. Similarly, SP2W6 and SP2Mo6 decompose at 240 °C and 255 °C respectively, while SP(NO3) is thermally stable up to 200 °C only.
Crystal structures of SP2W6, SN2Mo6, and SN2W6. Single-crystal X-ray diffraction analysis of SP2W6 reveals that this new compound is isostructural with SP2Mo6. SP2W6 is built upon isolated [W6O19]2− units, SP cations and crystallized acetonitrile molecules that are assembled in a supramolecular framework. The [W6O19]2− entities are well surrounded by the SP cations which interact with the POM surfaces via electrostatic interactions and C–H⋯O contacts (Fig. 2a). In particular, the planar methylpyridinium rings of two SP ions and the oxygen facets of the [W6O19]2− unit perfectly face each other with a very low dihedral angle (α = 4.0°), and the shortest C⋯O distance of 3.389(3) Å. These values are quite comparable with those observed for SP2Mo6 (dC⋯O = 3.372(5) Å, and α = 4.4°).
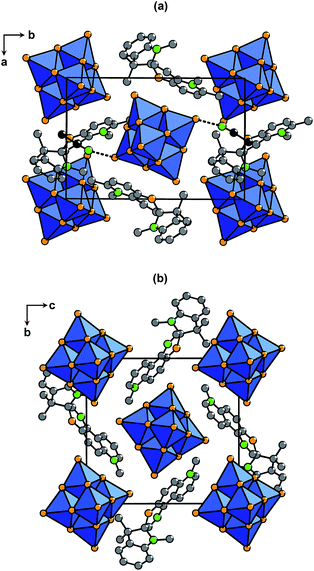 |
| Fig. 2 Mixed polyhedral and ball-and-stick representations of the crystal packing in (a) SP2W6 and (b) SN2W6 showing the interactions between the π-donor methylpyridinium groups of the SP and SN cations and the [W6O19]2− oxygen facets. (blue octahedra = WO6, grey spheres: carbons of SP and SN cations; dark spheres: carbons of acetonitrile molecules; green sphere: nitrogen; gold sphere: oxygen). N⋯O interactions between acetonitrile molecules and [W6O19]2− units in SP2W6 are displayed as dotted lines. The hydrogen atoms are omitted for clarity. | |
The isostructural compounds SN2W6 and SN2Mo6 are built upon [M6O19]2− blocks and SN cations (Fig. 2b). The structure of SN2W6 has been arbitrary chosen to describe the crystal packing of this series. The [W6O19]2− anions are well separated from each other in a cage-like supramolecular organic framework delimited by the SN cations (the shortest O⋯O distance between two adjacent [W6O19]2− units is 5.67 Å). The methylpyridinium group of four SN cations are quite parallel with the oxygen facets of the [W6O19]2− block and they interact together in a large contact zone, with a short interplanar distance (shortest C⋯O distance of 2.970(5) Å), and a low dihedral angle (α = 7.5°). These values match very well with those observed for SN2Mo6 (dC⋯O = 2.954(1) Å, and α = 7.0°).
To sum up, the structural studies of the SP2M6 and SN2M6 series well evidence that the spiro-molecules undergo very strong geometrical constraints, due to their very close proximity with the [M6O19]2− units, which should a priori be highly unfavourable for efficient photoisomerization. Nevertheless in each series, the organic cations interact with the POMs in a comparable way, enabling to maintain constant the steric factor, and to specifically investigate the influence of the nature of the metal in the POM unit, and thus the electronic factor, on the solid-state photochromic performances.
2.2 Optical properties
Optical properties before UV irradiation. The optical properties of SP2W6, SP2Mo6, SN2W6 and SN2Mo6 before UV irradiation have been investigated in ambient conditions by diffuse reflectance spectroscopy of microcrystalline powders. The main optical characteristics of the four hybrid systems are gathered in Table 1. Fig. 3 displays the absorption spectra of SP2W6 and SP2Mo6 compared with those of the iodide salt of the SP cation SP(I) and the (NBu4)2[M6O19] salts that contain similar [M6O19]2− units without SP entities. As previously discussed,17a the absorption spectrum of SP2Mo6 (Fig. 3a) in the UV domain is the sum of the absorption bands of the SP cation (for which the low-energy absorption is located at λmax(spiro) = 350 nm), and the broad ligand-to-metal charge-transfer (LMCT) transitions of the [Mo6O19]2− anion18 (λ(POM) = 269 and 327 nm). The absorptions of both POM and SP moieties exhibit a significant degree of overlapping which can be well assessed by introducing the Δλ parameter defined as Δλ = λmax(spiro) − λmax(POM), with λmax(POM) the low-energy LMCT transition centred on the POM unit. SP2Mo6 is then characterized by a very small Δλ value (23 nm). In strong contrast, for SP2W6 (Fig. 3b), the LMCT transitions of [W6O19]2− are located at much higher energies than those of [Mo6O19]2− i.e., λ(POM) = 220 nm (not shown in Fig. 3b) and 280 nm,18 and then, the Δλ value significantly increases to reach 70 nm. Furthermore, the [W6O19]2− anion does not absorb at λmax(spiro) = 350 nm. A similar effect is observed in the SN2M6 series (Fig. S3, ESI†). Indeed, Δλ = 48 and 95 nm for SN2Mo6 and SN2W6, respectively. These values are higher than those of SP2Mo6 and SP2W6 because SN absorbs at a higher wavelength (λmax(spiro) = 375 nm) than SP. In summary, the electronic factor is strongly modified by substituting Mo by W in the SP2M6 and SN2M6 series. This leads to a better separation in energy between the absorption bands of both organic and inorganic components in the UV domain. Consequently, in marked contrast with its Mo counterpart, the [W6O19]2− anion does not compete with SP and SN to absorb the excitation UV energy at λex = 365 nm needed to activate their ring-opening processes.
Table 1 Characteristic optical parameters of the SP2M6 and SN2M6 series
|
SP2Mo6 |
SP2W6 |
SN2Mo6 |
SN2W6 |
Lower energy POM LCMT transition. Δλ = λmax(spiro) − λmax(POM). Range of wavelength for the charge-transfer transition. Color of powdered sample before UV irradiation. |
λmax(POM)a (nm) |
327 |
280 |
327 |
280 |
λmax(spiro) (nm) |
350 |
350 |
375 |
375 |
Δλb (nm) |
23 |
70 |
48 |
95 |
λCTc (nm) |
430–540 |
400–510 |
430–590 |
420–560 |
Colord |
Brown-ochre |
Yellow-ochre |
Red-ochre |
Brown-ochre |
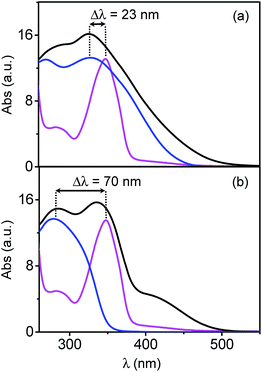 |
| Fig. 3 Normalized Kubelka–Munk transformed reflectivity spectra before UV irradiation of (a) SP2Mo6 (black), SP(I) (magenta), and (TBA)2[Mo6O19] (blue), and (b) SP2W6 (black), SP(I) (magenta), and (TBA)2[W6O19] (blue). The Δλ parameter is defined as Δλ = λmax(spiro) − λmax(POM), with λmax(POM) the low-energy LMCT transition of the POM unit. | |
The absorption spectra of SP2W6, SN2W6 and SN2Mo6 also exhibit an additional weak absorption band in the range 400–600 nm (Table 1), which is responsible for the color of powdered materials. This absorption is ascribed to an intermolecular Charge-Transfer (CT) transition between the electron-rich “π-donor” spiro-derivative and the electron-poor [M6O19]2− “acceptor”, due to their close proximity in the solid-state (see above).17a The CT band totally disappears when the samples are dissolved in highly polar solvents such as DMSO, and the resulting spectra exactly match the sum of the absorption spectra of isolated organic and [M6O19]2− ions (Fig. S4, ESI†). For a given electron-donor organic molecule, the energy of the CT transition increases with that of the LMCT band of the associated POM unit.17a Then, λCT are systematically blue-shifted in SN2W6 and SP2W6, and the color of these two powdered samples are less pronounced than those of their Mo counterparts (Fig. S5, ESI†), enabling us to speculate a better coloration contrast during the photochromic process.
Solid-state photochromic properties. First, the photochromic properties of SP2W6 and SP2Mo6 in ambient conditions have been compared (Fig. 4). While the color-change effect of SP2Mo6 is very limited under low-power near UV excitation (λex = 365 nm),17a SP2W6 exhibits a remarkably improved photochromic response under similar UV exposure (Fig. 4a). The color of the yellowish-ochre powder gradually shifts to purple-brown with a high coloration contrast and a fast coloration speed. Similarly as observed for SP2Mo6, the color change of SP2W6 deals with the appearance of the MC form of the SP cation that is optically characterized by an absorption band arising at λmax(MC) = 590 nm (Fig. 4b). This quite evidences that, even if both materials are isostructural, the SP → MC photoisomerization is much more efficient in SP2W6 than in SP2Mo6. This can be explained considering that in the case of SP2Mo6, most of the incident UV-light flow is strongly absorbed by the POM unit, and the resulting incident UV-light flow, available for the SP → MC photoisomerization, is much more limited than for SP2W6. Thus, the concentration of the photogenerated MC form is weaker in the hybrid polyoxomolybdate, and consequently, its color-change effect is dramatically affected. Furthermore, let us also noticed that the [M6O19]2− units do not contribute to the photogenerated coloration because, in contrast with the known photochromic polyoxometalate/organoammonium hybrid materials,10 their photoreduction does not occur in SP/POM assemblies.
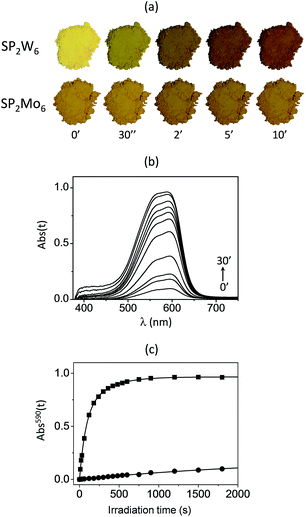 |
| Fig. 4 (a) Photographs of powders of SP2W6, and SP2Mo6 at different time (in min) during the coloration process under UV light (λex = 365 nm). (b) Temporal evolution of the absorption at 590 nm for SP2W6 after 0, 0.166, 0.333, 0.5, 1, 2, 3, 4, 5, 7, 10, and 30 min of 365 nm-UV irradiation. (c) Experimental (point) and fitted (line) Abs590(t) vs. t plots for SP2W6 (■), and SP2Mo6 (●). | |
The solid-state photochromic properties of SN2W6, and SN2Mo6 in ambient conditions exactly follow the same trend (Fig. 5). Noticeably, the brown-ochre powder of SN2W6 gradually changes to dark-green under UV excitation at λex = 365 nm (Fig. 5a). The photochromic effect that is detectable with naked eyes after only 2 s, finally reaches a saturation level after only 10 min, revealing a very fast photoresponse and a remarkable coloration contrast, even more pronounced than those of SP2W6. The intense photogenerated absorption band of the MC form of the SN cation is located at λmax(MC) = 620 nm in SN2W6 (Fig. 5b). This band is also observable in the optical spectrum of SN2Mo6 (Fig. S6, ESI†) but its intensity is much weaker. This latter compound develops very limited photochromic performances, and the color-change effect remains hardly detectable by naked eyes.
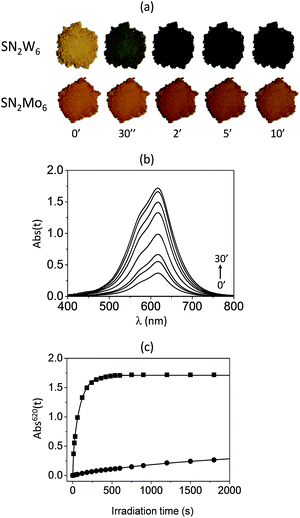 |
| Fig. 5 (a) Photographs of powders of SN2W6, and SN2Mo6 at different time (in min) during the coloration process under UV light (λex = 365 nm). (b) Temporal evolution of the absorption at 620 nm for SN2W6 after 0, 0.166, 0.333, 0.5, 1, 2, 3, 6, and 10 min of 365 nm-UV irradiation. (c) Experimental (point) and fitted (line) Abs620(t) vs. t plots for SN2W6 (■), and SN2Mo6 (●). | |
The coloration kinetics of the four hybrid systems have been quantified at room temperature by monitoring the photogenerated absorption at λmax(MC) (Absλmax(t)) as a function of the UV irradiation time t. The Absλmax(t) vs. t plots have been adequately fitted using a biexponential rate law16,17a (Fig. 4c and 5c for the SP2M6 and SN2M6 series, respectively), and the kinetics parameters are gathered in Table S1, ESI†. The photocoloration rates of the compounds can be well compared by considering their half-life time (t1/2) namely the UV irradiation time required for Absλmax(t) to reach half of its maximum value. SN2W6 exhibits the most effective photocoloration process, much more intense and faster than the three other compounds. Importantly, its photocoloration rates (t1/2 = 0.8 min) is twenty times faster than that of SN2Mo6 (t1/2 = 16.0 min). In the SP2M6 series, the photocoloration rate is about eleven more times faster for SP2W6 (t1/2 = 1.3 min) than for SP2Mo6 (t1/2 = 15.0 min). This quite evidences that the SP and SN photoisomerization rates are drastically improved by substituting [Mo6O19]2− by [W6O19]2− in both series, despite the strong steric constraints applied to the spiro-derivative molecules by the POM framework.
The fading kinetics of SN2W6 and SP2W6 have been also investigated at room temperature to evaluate the efficiency of the MC → SN and MC → SP back conversions in the dark (thermal fading), and under visible-light irradiation at 630 and 590 nm, respectively, i.e. with energies corresponding to the absorption band of the MC forms of the SN and SP ions. The Absλmax(t) vs. t plots of the coloration and fading processes are displayed in Fig. 6a for SN2W6 and S7, ESI† for SP2W6. Decays have been well fitted using a biexponential rate law, (see Table S2, ESI† for the detailed fading kinetic parameters). The fading rates have been compared by considering their fading half-life time. Under visible-light irradiation, SN2W6 and SP2W6 exhibit very fast and full bleaching processes. Very interestingly, the fading rates are comparable with the coloration ones (for the bleaching process, t1/2 = 0.6 and 0.2 min for SN2W6 and SP2W6, respectively). In strong contrast, both hybrid systems develop very limited thermal fading processes. Indeed, the maximum of absorption loss after 2 hours reaches only 6% for SN2W6 and 8% in the case of SP2W6, revealing that after switching off the UV irradiation, most of the MC forms remain stabilized in the polar hybrid POM framework. Hence, SN2W6 and SP2W6 are quasi-bistable at room temperature.
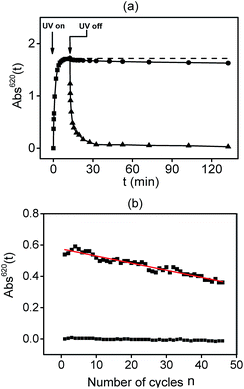 |
| Fig. 6 (a) Comparison of the temporal evolutions of the absorbance at 620 nm for SN2W6 under red light (λex = 630 nm) (▲) and in the dark (●) for a sample initially irradiated for 10 min with UV light (λex = 365 nm) (■). The dashed line shows the absorbance value just before switching off the UV light. (b) Evolution of the absorbance monitored at 620 nm for SN2W6 during successive coloration/bleach cycles at room temperature. In each cycle, the sample was irradiated for 1 min with UV light (λex = 365 nm), and then for 10 min with red light (λex = 630 nm). The red line corresponds to the linear fit of Abs620(n) vs. n (R2 = 0.965). | |
Finally, the robustness of SN2W6 and SP2W6 for repeatable “recording–erasing” processes at room temperature was investigated by monitoring the evolution of Absλmax of samples alternatively irradiated under UV light and visible light. We managed to perform a long study of cyclability by following the variation of the absorption on approximately fifty cycles, what to our knowledge, was never reported for powdered photochromic samples so far. In the case of SN2W6, the evolution of Abs620(n) vs. n, i.e., the number of coloration/fading cycles, reveals that this hybrid system shows a good cyclability with a high coloration contrast (Fig. 6b). For each cycle, the bleaching process is full, but a fatigue is observed during the successive photocoloration processes. The photogenerated absorption progressively decreases with n, and the absorption loss is about 33% at the end of fifty cycles. Surprisingly, the decrease of Abs620(n) with n, can be fitted with the empirical linear relationship described in eqn (1). This relationship allows predicting that the variation of absorption between both colorless and colored states should be zero at the end of 128 cycles.
|
Abs620(n) = −0.0045n + 0.5751
| (1) |
The photocoloration fatigue is more marked for SP2W6, and the absorption loss reaches about 50% at the end of fifty cycles (Fig. S7b, ESI†). Similarly, Abs590(n) linearly decreases with n according to eqn (2), revealing that the color-change effect should disappear at the end of 98 cycles.
|
Abs590(n) = −0.0040n + 0.3916
| (2) |
At first sight, the poorer cyclability of SP2W6 could result from the presence of the crystallized acetonitrile molecules which is expected to introduce some flexibility in the hybrid framework.
3. Conclusion
In conclusion, the two first supramolecular hybrid isopolyoxotungstates SN2W6 and SP2W6 have been successfully designed by assembling the Lindqvist-type [W6O19]2− unit with cationic spirooxazine and spiropyran. Noticeably, while the SN and SP ions undergo strong steric constraints in the frameworks, these systems exhibit remarkable solid-state photochromic properties in ambient conditions, which include high photocoloration contrasts, fast coloration and fading rates, and good cyclabilities. Besides, SN2W6 and SP2W6 are quasi-bistable systems at room temperature, and they possess high light-driven “recording–erasing” potentialities. Strikingly, their photochromic performances are drastically improved compared to SN2Mo6 and SP2Mo6, their isostructural Mo counterparts, revealing that the photoisomerization of the spiro-molecules is much more impacted by the electronic factor than by the steric one. In strong contrast with [Mo6O19]2−, the high-energy LMCT transition of [W6O19]2− does not overlap the absorption of the SN and SP cations in the UV domain, and both organic and inorganic components do not compete to absorb the excitation UV light.
The findings may open a new pathway for the design of supramolecular SP or SN/POMs assemblies with outstanding solid-state photochromic properties. Importantly, they quite evidence that efficient photochromic hybrid systems can be designed even if their crystal packings are a priori highly unfavourable for the photoisomerization of the spiro-molecules. In addition, the substitution of Mo by W is a very useful tool to favourably tune the electronic factor. We are currently working to characterize the structure of SP2W6 and SN2W6 in their colored forms using single-crystal X-ray photodiffraction analyses. Exploitation of the remarkable photochromic properties of SN2W6 and SP2W6 is also now under investigation. In particular, their integration into organic polymers or as thin film to elaborate photonic devices and sensors will be considered. This kind of immobilisation is also expected to increase the resistance to “fatigue”.20 In addition, the synthesis of new supramolecular assemblies built upon other isopolyoxotungstates varying in shape, size and charge are currently under study. Finally, new hybrid systems, based on polyoxotungstates and photoactive organic dyes associating both photochromism and fluorescence are also underway.
4. Experimental section
Synthesis
SP(NO3),21 SN(I),22 (NBu4)2[W6O19]23 and (NBu4)2[Mo6O19]24 have been synthesized according to the reported procedures.
SN(NO3). SN(I) (1 g, 2.1 mmol) was dissolved in a cosolvent MeOH/CH3CN (30/25 mL) (solution 1). AgNO3 (360 mg, 2.1 mmol) was dissolved in MeOH (15 mL) (solution 2). Solution 2 was added dropwise to solution 1 under stirring leading to the precipitation of a yellow solid of AgI. The solution was filtered through a short plug of celite to afford an oily solution after removal of the solvent under reduced pressure. The solution was then precipitated in ether and the brown powder obtained was washed with ether and dried in air. SN(NO3) was obtained in 40% yield. 1H NMR (300 MHz, CDCl3) δ (ppm) 9.82 (d, 1H), 9.60 (d, 1H), 8.30 (d, 1H), 8.1 (dd, 1H), 7.92 (s, 1H), 7.7 (d, 1H), 7.11 (d, 1H), 6.97 (t, 1H), 6.62 (d, 1H), 4.84 (s, 1H), 2.77 (s, 3H), 1.40 (s, 3H), 1.36 (s, 3H).
(SN)2[Mo6O19] (SN2Mo6). (NBu4)2[Mo6O19] (220 mg, 0.16 mmol) was dissolved in DMF (9 mL). The solution (solution 1) was stirred for a few minutes at room temperature. In addition, a second solution (solution 2) was obtained by dissolving SN(NO3) (150 mg, 0.37 mmol) in DMF (5 mL) at room temperature. Solution 2 was added dropwise to solution 1 under vigorous stirring. The mixture was stirred at 40 °C for two hours, kept at room temperature, and then filtered. Crystallization of the product by diffusion of ethanol into the filtrate gave suitable single crystals for X-ray diffraction. Yield in Mo: 64%. Anal. calcd for C44H44O21N6Mo6: C, 33.42; H, 2.78; N, 5.32. Found: C, 33.38; H, 2.80; N, 5.29. FT-IR (KBr, cm−1): SN cations 1629 (m), 1604 (s), 1584 (s), 1531 (m), 1487 (s), 1467 (m), 1441 (m), 1425 (m), 1394 (s), 1368 (m), 1349 (sh), 1306 (m), 1284 (s), 1237 (m), 1189 (w), 1169 (m), 1147 (m), 1130 (m), 1119 (w), 1108 (sh), 1079 (m), 1055 (s), 1023 (m), 1003 (w); νMo
O, νMo–O–Mo 959 (vs), 928 (sh), 912 (w), 892 (w), 796 (vs), 751 (s), 682 (w), 655 (w), 623 (sh), 600 (m), 577 (m), 538 (m), 522 (sh), 477 (sh), 463 (m), 437 (m). From ATG/DSC measurements, SN2Mo6 is stable up to 280 °C.
(SN)2[W6O19] (SN2W6). (NBu4)2[W6O19] (305 mg, 0.16 mmol) was dissolved in DMF (8 mL). The solution (solution 1) was stirred for a few minutes at room temperature. In addition, a second solution (solution 2) was obtained by dissolving SN(NO3) (200 mg, 0.49 mmol) in DMF (5 mL) at room temperature. Solution 2 was added dropwise to solution 1 under vigorous stirring. The mixture was stirred at 40 °C for one hour and a half, kept at room temperature, and then filtered. Crystallization of the product by diffusion of ethanol into the filtrate gave suitable single crystals for X-ray diffraction. Yield in W: 46%. Anal. calcd for C44H44O21N6W6: C, 25.19; H, 2.10; N, 4.01. Found: C, 25.15; H, 2.07; N, 4.06. FT-IR (KBr, cm−1): SN cations 1628 (w), 1601 (m), 1583 (m), 1529 (m), 1487 (s), 1466 (m), 1440 (m), 1424 (m), 1395 (m), 1366 (m), 1347 (sh), 1324 (w), 1305 (m), 1283 (s), 1260 (sh), 1236 (m), 1188 (w), 1168 (m), 1144 (w), 1128 (m), 1119 (w), 1106 (w), 1077 (m), 1056 (m), 1024 (m); νW
O, νW–O–W 980 (vs), 927 (m), 907 (w), 890 (w), 810 (vs), 752 (s), 679 (m), 670 (m), 653 (sh), 632 (sh), 623 (sh), 583 (m), 537 (m), 520 (w), 443 (s). From ATG/DSC measurements, SN2W6 is stable up to 260 °C.
(SP)2[W6O19]·0.5CH3CN (SP2W6). (NBu4)2[W6O19] (305 mg, 0.16 mmol) was dissolved in acetonitrile (10 mL). The solution (solution 1) was stirred for a few minutes at room temperature. In addition, a second solution (solution 2) was obtained by dissolving SP(NO3) (200 mg, 0.49 mmol) in acetonitrile (6 mL) at room temperature. Solution 2 was added dropwise to solution 1 under vigorous stirring leading to the slow precipitation of an ochre powder of SP2W6. The mixture was stirred at 40 °C for one hour and a half, kept at room temperature, and then filtered. The powder was washed with acetonitrile and ethanol, and dried in air. Yield in W: 53%. Crystallization of the product by diffusion of ethanol into the filtrate gave suitable single crystals for X-ray diffraction. Anal. calcd for C41H47.5O21N4.5W6: C, 24.09; H, 3.32; N, 3.08. Found: C, 24.15; H, 3.34; N, 3.12. FT-IR (KBr, cm−1): SP cations 1608 (m), 1486 (s), 1472 (m), 1454 (m), 1433 (w), 1386 (w), 1362 (m), 1311 (s), 1297 (s), 1279 (w), 1252 (w), 1236 (w), 1207 (sh), 1190 (w), 1168 (w), 1047 (w), 1017 (w), 1104 (w), 1057 (m), 1025 (m); νW
O, νW–O–W 977 (vs), 926 (m), 915 (m), 878 (w), 810 (vs), 765 (m), 707 (m), 661 (w), 585 (m), 552 (w), 530 (w), 445 (s). From DSC/TGA measurements, SP2W6 loses the acetonitrile molecules above 180 °C.
X-ray crystallography
Data collection was carried out by using a Siemens SMART three-circle diffractometer for SN2Mo6 and by using a Bruker Nonius X8 APEX 2 diffractometer for SN2W6 and SP2W6. Both were equipped with a CCD bi-dimensional detector using the monochromatized wavelength λ (Mo Kα) = 0.71073 Å. Absorption correction was based on multiple and symmetry-equivalent reflections in the data set using the SADABS program25 based on the method of Blessing.26 The structures were solved by direct methods and refined by full-matrix least-squares using the SHELX-TL package.27 Crystallographic data are given in Table 2. CCDC 1414602–1414604 contain the supplementary crystallographic data for this paper. These data can be obtained free of charge from the Cambridge Crystallographic Data Centre via http://www.ccdc.cam.ac.uk/data_request/cif.†
Table 2 Crystallographic data
|
SN2Mo6 |
SN2W6 |
SP2W6 |
Empirical formula |
C44H44N6O21Mo6 |
C44H44N6O21W6 |
C41H47.5N4.5O21W6 |
Formula weight [g] |
1568.49 |
2095.95 |
2042.43 |
Temperature [K] |
298 |
298 |
298 |
Crystal system |
Monoclinic |
Monoclinic |
Monoclinic |
Space group |
P21/n |
P21/n |
P21/n |
a [Å] |
14.3898(6) |
14.410(3) |
10.4923(2) |
b [Å] |
12.7305(5) |
12.743(3) |
15.1018(3) |
c [Å] |
14.4617(7) |
14.549(3) |
16.1338(2) |
β [°] |
111.279(2) |
111.339(10) |
104.6525(6) |
V [Å3] |
2468.6(2) |
2488.5(9) |
2473.30(7) |
Z |
2 |
2 |
2 |
ρcalc [g cm−3] |
2.110 |
2.797 |
2.743 |
μ [mm−1] |
1.566 |
13.899 |
13.979 |
Data/parameters |
7214/353 |
7253/353 |
7227/340 |
Rint |
0.0353 |
0.0307 |
0.0489 |
GOF |
0.959 |
1.032 |
1.119 |
R1 (>2σ(I)) |
0.0264 |
0.0208 |
0.0320 |
wR2 |
0.0600 |
0.0563 |
0.0908 |
Physical measurements
Elemental analyses of the solids were performed by the “Service de microanalyses ICSN CNRS, in Gif sur Yvette (France), and by the “Service d'Analyse du CNRS”, in Vernaison (France). FT-IR spectra were recorded in the 4000–400 cm−1 range on a BRUKER Vertex equipped with a computer control using the OPUS software. Differential scanning calorimetry (DSC) and thermogravimetric analysis (TGA) were performed by flowing dry argon with a heating and cooling rate of 5 °C min−1 on a SETARAM TG-DSC 111 between 20 and 800 °C. Diffuse reflectance spectra were collected at room temperature on a finely ground sample with a Cary 5G spectrometer (Varian) equipped with a 60 mm diameter integrating sphere and computer control using the “Scan” software. Diffuse reflectance was measured from 250 to 1550 nm with a 2 nm step using Halon powder (from Varian) as reference (100% reflectance). The reflectance data were treated by a Kubelka–Munk transformation28 to obtain the corresponding absorption data. The photocoloration and fading kinetics were quantified by monitoring the temporal evolution of the photogenerated absorption Absλmax(t) defined as Absλmax(t) = −log(Rλmax(t)/Rλmax(0)), where Rλmax(t) and Rλmax(0) are the reflectances at the time t and at t = 0, respectively. For the coloration kinetics, the samples were irradiated with a Fisher Bioblock labosi UV lamp (λex = 365 nm, P = 6 W) at a distance of 50 mm. Absλmax(t) vs. t plots have been fitted according to a biexponential rate law Absλmax(t) = (A1 + A2) − A1
exp(−k1ct) − A2
exp(−k2ct), with k1c and k2c the extracted coloration rate constants. For the bleaching processes, the samples were first irradiated under 365 nm-UV excitation for 10 minutes in the case of SN2W6, and for 20 minutes in the case of SP2W6, times for which the photoinduced absorptions are quasi-saturated. Then, the compounds were put under Thorlabs LED Array light sources (590 nm to 1.4 W cm−2 and 630 nm to 2.4 W cm−2) at a distance of 100 mm. The bleaching kinetics were determined at room temperature by monitoring the temporal decays of Absλmax(t) of samples once irradiated Absλmax(t) vs. t plots have been fitted according to a biexponential rate law Absλmax(t) = (A0 − A1 − A2) + A1
exp(−k1ft) + A2
exp(−k2ft), with k1f and k2f the extracted fading rate constants.
Acknowledgements
This work was supported by the CNRS, the Ministère de l'Enseignement Supérieur et de la Recherche, the ANR-11-BS07-011-01 BIOOPOM, and the LUMOMAT project supported by the Région des Pays de la Loire.
Notes and references
-
(a) Y. Hirshberg and E. Fisher, J. Chem. Phys., 1955, 23, 1723 CrossRef CAS;
(b) H. Bouas-Laurent, Photochromism: Molecules and Systems, Elsevier, Amsterdam, 2003 Search PubMed.
- C. Bechinger, S. Ferrere, A. Zaban, J. Sprague and B. A. Gregg, Nature, 1996, 383, 608 CrossRef CAS.
- B. L. Feringa, Molecular Switches, Wiley-VCH, New York, 2001 Search PubMed.
-
(a) M. Irie, Chem. Rev., 2000, 100, 1685 CrossRef CAS PubMed;
(b) S. Kawata and Y. Kawata, Chem. Rev., 2000, 100, 1777 CrossRef CAS PubMed;
(c) J. A. Delaire and K. Nakatani, Chem. Rev., 2000, 100, 1817 CrossRef CAS PubMed;
(d) C. C. Corredor, Z.-L. Huang, K. D. Belfield, A. R. Morales and M. V. Bondar, Chem. Mater., 2007, 19, 5165 CrossRef CAS;
(e) Y. Yokoyama, Chem. Rev., 2000, 100, 1717 CrossRef CAS PubMed.
-
(a) M. Natali and S. Giordani, Chem. Soc. Rev., 2012, 41, 4010 RSC;
(b) V. I. Minkin, Chem. Rev., 2004, 104, 2751 CrossRef CAS PubMed;
(c) G. Berkovic, V. Krongauz and V. Weiss, Chem. Rev., 2000, 100, 1741 CrossRef CAS PubMed.
-
(a) B. Schaudel, C. Guermeur, C. Sanchez, K. Nakatani and J. A. Delaire, J. Mater. Chem., 1997, 7, 61 RSC;
(b) J. Biteau, F. Chaput and J.-P. Boilot, J. Phys. Chem., 1996, 100, 9024 CrossRef CAS;
(c) W. Yuan, L. Sun, H. Tang, Y. Wen, G. Jiang, W. Huand, L. Jiang, Y. Song, H. Tian and D. Zhu, Adv. Mater., 2005, 17, 156 CrossRef CAS;
(d) R. A. Kopelman, S. M. Snyder and N. L. Franck, J. Am. Chem. Soc., 2003, 125, 13684 CrossRef CAS PubMed.
- J. Harada, Y. Kawazoe and K. Ogawa, Chem. Commun., 2010, 46, 2593 RSC.
-
(a) B. Osterby, R. D. McKelvey and L. Hill, J. Chem. Educ., 1991, 68, 424 CrossRef CAS;
(b) D. Levy, Chem. Mater., 1997, 9, 2666 CrossRef CAS;
(c) C. Sanchez, B. Lebeau, F. Chaput and J.-P. Boilot, Adv. Mater, 2003, 15, 1969 CrossRef CAS;
(d) M.-Q. Zhu, L. Zhu, J. J. Han, J. K. Hurst and A. D. Q. Li, J. Am. Chem. Soc., 2006, 128, 4303 CrossRef CAS PubMed;
(e) I. Khale, O. Tröber, S. Trentsch, H. Richter, B. Grünler, S. Hemeltjen, M. Schlesinger, M. Mehring and S. Spange, J. Mater. Chem., 2011, 21, 5083 RSC.
-
(a) Polyoxometalate Chemistry: From Topology via Self-Assembly to Applications, ed. M. T. Pope and A. Müller, KLUWER, Dordrecht, Netherlands, 2001 Search PubMed;
(b) Polyoxometalate Chemistry for Nano-Composite Design, ed. T. Yamase and M. T. Pope, KLUWER, New York, 2002 Search PubMed;
(c) Polyoxometalate Molecular Science, ed. J. J. Borras-Almenar, E. Coronado, A. Müller and M. T. Pope, KLUWER, Dordrecht, Netherlands, NATO Science Series II, Vol. 98, 2003 Search PubMed;
(d) D.-L. Long, E. Burkholder and L. Cronin, Chem. Soc. Rev., 2007, 36, 105 RSC;
(e) A. Dolbecq, E. Dumas, C. R. Mayer and P. Mialane, Chem. Rev., 2010, 110, 6009 CrossRef CAS PubMed;
(f) U. Kortz, A. Müller, J. V. Salgeren, J. Schnack, N. S. Dalal and M. Dressel, Coord. Chem. Rev., 2009, 253, 2315 CrossRef CAS;
(g) P. Gouzerh and A. Proust, Chem. Rev., 1998, 98, 77 CrossRef CAS PubMed;
(h) D.-L. Long, R. Tsunashima and L. Cronin, Angew. Chem., Int. Ed., 2010, 49, 1736 CrossRef CAS PubMed.
-
(a) T. Yamase, Chem. Rev., 1998, 98, 307 CrossRef CAS PubMed;
(b) L. Yang, Z. Zhou, P. Ma, J. Wang and J. Niu, Cryst. Growth Des., 2013, 13, 2540 CrossRef CAS;
(c) Y. Wang, P. Ma and J. Niu, Dalton Trans., 2015, 44, 4679 RSC;
(d) Y. Chen, G. Yu, F. Li, C. Xie and G. Tian, J. Mater. Chem. C, 2013, 1, 3842 RSC;
(e) X. Luo and C. Yang, Phys. Chem. Chem. Phys., 2011, 13, 7892 RSC;
(f) K. Hakouk, O. Oms, A. Dolbecq, H. El Moll, J. Marrot, M. Evain, F. Molton, C. Duboc, P. Deniard, S. Jobic, P. Mialane and R. Dessapt, Inorg. Chem., 2013, 52, 555 CrossRef CAS PubMed;
(g) R. Dessapt, M. Collet, V. Coué, M. Bujoli-Doeuff, S. Jobic, C. Lee and M.-H. Whangbo, Inorg. Chem., 2009, 48, 574 CrossRef CAS PubMed.
-
(a) S. Liu, D. G. Kurth, H. Möhwald and D. Volkmer, Adv. Mater., 2002, 14, 225 CrossRef CAS;
(b) G. Gao, L. Xu, W. Wang, W. An, Y. Qiu, Z. Wang and E. Wang, J. Phys. Chem. B, 2005, 109, 8948 CrossRef CAS PubMed;
(c) L. Jin, Y. Fang, P. Hu, Y. Zhai, E. Wang and S. Dong, Chem. Commun., 2012, 48, 2101 RSC.
-
(a) L. Xu, E. Wang, Z. Li, D. G. Kurth, X. Du, H. Zhang and C. Qin, New J. Chem., 2002, 26, 782 RSC;
(b) J.-D. Compain, P. Mialane, A. Dolbecq, J. Marrot, A. Proust, K. Nakatani, P. Yu and F. Sécheresse, Inorg. Chem., 2009, 48, 6222 CrossRef CAS PubMed.
-
(a) L. Xu, H. Zhang, E. Wang, D. G. Kurth and Z. Li, J. Mater. Chem., 2002, 12, 654 RSC;
(b) H. Li, W. Qi, W. Li, H. Sun, W. Bu and L. Wu, Adv. Mater., 2005, 17, 2688 CrossRef CAS.
- A. Parrot, G. Izzet, L.-M. Chamoreau, A. Proust, O. Oms, A. Dolbecq, K. Hakouk, H. El Bekkachi, P. Deniard, R. Dessapt and P. Mialane, Inorg. Chem., 2013, 52, 11156 CrossRef CAS PubMed.
- O. Oms, K. Hakouk, R. Dessapt, P. Deniard, S. Jobic, A. Dolbecq, T. Palacin, L. Nadjo, B. Keita, J. Marrot and P. Mialane, Chem. Commun., 2012, 48, 12103 RSC.
- A. Saad, O. Oms, J. Marrot, A. Dolbecq, K. Hakouk, H. El Bekkachi, S. Jobic, P. Deniard, R. Dessapt, D. Garrot, K. Boukheddaden, R. Liu, G. Zhang, B. Keita and P. Mialane, J. Mater. Chem. C, 2014, 2, 4748 RSC.
-
(a) K. Hakouk, O. Oms, A. Dolbecq, J. Marrot, A. Saad, P. Mialane, H. El Bekkachi, S. Jobic, P. Deniard and R. Dessapt, J. Mater. Chem. C, 2014, 2, 1628 RSC;
(b) J.-D. Compain, P. Deniard, R. Dessapt, A. Dolbecq, O. Oms, F. Sécheresse, J. Marrot and P. Mialane, Chem. Commun., 2010, 46, 7733 RSC;
(c) J.-D. Compain, P. Mialane, J. Marrot, F. Sécheresse, W. Zhu, E. Oldfield and A. Dolbecq, Chem.–Eur. J., 2010, 16, 13441 CrossRef PubMed.
- J. Li, J. Cluster Sci., 2002, 13, 137 CrossRef CAS.
- D. Hagrman, P. J. Hagrman and J. Zubieta, Angew. Chem., Int. Ed., 1999, 38, 3165 CrossRef CAS PubMed.
- R. Klajn, Chem. Soc. Rev., 2014, 43, 148 RSC.
- P. Mialane, G. J. Zhang, I. M. Mbomekallé, P. Yu, J.-D. Compain, A. Dolbecq, J. Marrot, F. Sécheresse, B. Keita and L. Nadjo, Chem.–Eur. J., 2010, 16, 5572 CrossRef CAS PubMed.
- S. Benard and P. Yu, Chem. Commun., 2000, 65 RSC.
- C. Sanchez, J. Livage, J. P. Launay and M. Fournier, J. Am. Chem. Soc., 1983, 105, 6817 CrossRef CAS.
- W. G. Klemperer, Inorg. Synth., 1990, 27, 74 CrossRef CAS.
- G. M. Sheldrick, SADABS; program for scaling and correction of area detector data, University of Göttingen, Germany, 1997 Search PubMed.
- R. Blessing, Acta Crystallogr., Sect. A: Found. Crystallogr., 1995, A51, 33 CrossRef CAS.
- G. M. Sheldrick, SHELX-TL Version 5.03, Software Package for the Crystal Structure Determination, Siemens Analytical X-ray Instrument Division, Madison, WI, USA, 1994 Search PubMed.
- P. Kubelka and F. Munk, Z. Tech. Phys., 1931, 12, 593 Search PubMed.
Footnote |
† Electronic supplementary information (ESI) available: Complementary Kubelka–Munk transformed reflectivity and absorption spectra, UV-vis absorption spectra in solution, photocoloration and fading kinetics parameters. CCDC 1414602–1414604. For ESI and crystallographic data in CIF or other electronic format see DOI: 10.1039/c5ra15860e |
|
This journal is © The Royal Society of Chemistry 2015 |