DOI:
10.1039/C5RA14944D
(Paper)
RSC Adv., 2015,
5, 86529-86535
Transfer hydrogenation of nitroarenes into anilines by palladium nanoparticles via dehydrogenation of dimethylamine borane complex†
Received
28th July 2015
, Accepted 8th October 2015
First published on 8th October 2015
Abstract
This work reports a simple and highly efficient protocol for reduction of nitroarenes to corresponding amines via dehydrogenation of dimethylamine borane using palladium nanoparticle (Pd NPs) as a versatile heterogeneous catalyst. The facile approach for the synthesis of Pd NPs within 15 min in aqueous medium has been reported. The Pd NPs were well characterized using various analytical techniques such as XRD, FEG-SEM, TEM, EDAX and XPS. The developed catalytic system uses environmentally benign dimethylamine borane as a reducing agent which is highly stable, water soluble and nontoxic. The various amines were synthesized from nitroarenes in excellent yields within 10–60 min at room temperature. The catalyst was reused up to four successive cycles without significant loss in its catalytic activity.
Introduction
Hydrogen has been playing an important role as an alternative power source for the transportation of fuel and fuel cell vehicles.1 However, from the safety point of view, it is very difficult to control its storage and release limits.2 So, nowadays various materials such as metal–organic frameworks (MOF),3 metal-hydrides4 and organic materials5 have been tested for the storage of hydrogen. Moreover, the above-mentioned materials require high temperature, pressure and have problem with storage capacity. Recently, ammonia-borane class of family has attracted great attention as a versatile material for chemical hydrogen storage because of their low thermolysis temperature, high stability, high water solubility and non toxicity.6 The dehydrogenation of ammonia-borane (NH3BH3, AB) and its derivatives like methylamine-borane (CH3NH2BH3, MeAB) and dimethylamine-borane (Me2NHBH3, DMAB) have found wide potential applications in material science,7 polymer synthesis,8 transfer hydrogenation9 and tandem dehydrogenation–hydrogenation reactions.10 In particularly, the dehydrogenation of DMAB was studied using various transition-metal catalysts such as palladium, ruthenium, iridium,11 rhodium,12 rhenium,13 zirconium and titanium.14,15 Recently, Chirik's reported homogeneous titanium precursor complex [(η5-C5H3-1,3-(SiMe3)2)2Ti]2(μ2,η1,η1-N2) for dehydrogenation of DMAB.14 It is noteworthy that, Williams and co-workers explored homogenous ruthenium and copper complexes for hydrogenation of various organic moieties with DMAB as the reducing agent.16 However, in spite of their potential utility, the above reports suffer from one or more drawbacks such as use of phosphine ligands, longer reaction time, harsh reaction conditions, difficulties in catalyst-product separation and complicated catalyst preparation procedure. So, considering these drawbacks of homogenous system, the effort was made to develop better heterogeneous recyclable catalytic system for the dehydrogenation of DMAB.
Amine and their derivatives are important intermediates in pharmaceuticals, agrochemicals, dyes, pigments, fine chemical industries, biotechnology and material science.17 The catalytic hydrogenation or transfer hydrogenation of nitro compounds is one of the most useful methods for the synthesis of primary amines. In this regard, Beller and co-workers have developed heterogeneous cobalt oxide and iron oxide based catalytic system for the hydrogenation of nitroarenes using molecular hydrogen.18 Nowadays, use of AB and DMAB as a reducing agent has paying considerable attention due to its high volume/mass hydrogen density, non toxicity and high stability in water.19 In 2013, Stratakis and co-workers reported Au NPs catalyzed hydrogenation of nitroarenes using AB as reducing agent.20 Later, Goksu et al. employed graphene-supported NiPd and CoPd alloy catalytic system for reduction of nitroarenes using AB as a hydrogen source.21 Subsequently, Yurderi et al. have explored Ru(0) NPs stabilized by MOF for hydrogenation of unsaturated hydrocarbons with dehydrogenation of DMAB.22
The nanoparticles have advantages over bulk materials such as high surface area, which lead to increase in contact between reactants, lower catalyst loading, reaction does not require any ligand source, and low temperature.23 The palladium metal has been extensively used for hydrogenation and transfer hydrogenation reactions.24 In current research, the Pd NPs have been growing steadily due to their fascinating properties and activity. Hence, the Pd NPs have found several applications in various fields such as lithium-ion batteries, electronics, biomedical sensing, surface enhanced Raman scattering, hydrogen storage, magnetizations, water treatment and heterogeneous catalyst.25–29 Literature study reveals that many methods have been developed for the synthesis of Pd NPs like using solar energy,29 chemical reduction,30 by supercritical CO2,31 sonochemical method,32 electrochemical method33 and biological method.34 Nagaraju and co-workers demonstrated the synthesis of graphene–palladium nanocomposites using hydrazine hydrate.35 Kalbasi and Mosaddegh reported Pd-poly(N-vinyl-2-pyrrolidone)/KIT-5 nanocomposite using hydrazine hydrate.36 Shen and co-workers synthesized poly(acrylic acid)–Pd(0) composites films using hydrazine hydrate.37 However, these protocols have some drawbacks such as use of hazards reagents, high temperature and long reaction time, use of specialized instruments and necessity of extra additives. Hence, there is need for the development of simple, faster and convenient method for the synthesis of Pd NPs. Here, we have developed a simple and inexpensive method for the synthesis of spherical Pd NPs via chemical reduction of PdCl2 using PEG-6000 as a capping agent and hydrazine hydrate as a reducing agent in aqueous medium within 15 min. Furthermore, the transfer hydrogenation of nitroarenes into corresponding amines using present Pd NPs via dehydrogenation of DMAB as a hydrogen source was developed at room temperature (Scheme 1).
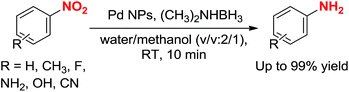 |
| Scheme 1 Transfer hydrogenation of nitroarenes via dehydrogenation of DMAB. | |
Experimental
Materials
The palladium chloride (PdCl2) was acquired from Parekh Industries Ltd. Mumbai, India. Polyethylene glycol (PEG-6000) and hydrazine hydrate (100%) were procured from S. D. Fine chemicals Pvt. Ltd. India. Dimethylamine-borane (Me2NHBH3) was purchased from Sigma-Aldrich. All chemicals were procured from reputed firm and was used without further purification.
Preparation of Pd NPs
In a round bottom flask, 20 mg of PdCl2 and 40 mg of PEG-6000 were dissolved in 5 mL distilled water and then heated at 80 °C for 5 min. After 5 min, the hydrazine hydrate (300 μL, 100%) was added drop wise into the above mixture and then stirred for 10 min at 80 °C. After completion of reaction, the color of the reaction mass changes reddish to black this indicates the formation of Pd NPs. The catalyst was separated by centrifugation of reaction mixture at 5000 rpm for 10 min. The final product was washed several times with distilled water followed by absolute ethanol and dried at 60 °C for 1 h in the oven.
Methods of characterization
X-ray diffraction (XRD) patterns was recorded on a Shimadzu XRD-6100 using Cu Kα radiations = 1.5405 Å with a scanning rate of 2° per min and 2 theta (θ) angle ranging from 20° to 80° with current 30 mA and voltage 40 kV. The morphology of prepared nanocatalyst was observed using field emission gun-scanning electron microscope (FEG-SEM) analysis on Tescan MIRA 3 model with operating voltage between 10.0 kV to 20.0 kV using secondary electron (SE) detector and transmission electron microscopy (TEM) using Philips CM-200, operating voltage at 200 kV. The energy dispersive X-ray spectrum (EDAX) was obtained using INCA x-act Oxford instrument (Model 51-ADD0007). The X-ray photoelectron spectrum (XPS) analysis of synthesized Pd NPs were recorded using PHI 5000 versa probe scanning ESCA microprobe.
Results and discussion
Catalyst characterization
The synthesized Pd NPs were characterized using various techniques. The phase identification of Pd NPs was carried out using an X-ray diffractometer (Fig. 1), which show the diffraction peaks at 2θ values of 40°, 47°, 68°, 82° and 87° correspond to the (111), (200), (220), (311) and (222) phases for Pd NPs. The obtained results from XRD are in good agreement with JCPDS card 05-0681. The morphology of Pd NPs was carried out using field emission gun-scanning electron microscope (FEG-SEM). The low magnification (Fig. 2a) and high magnification (Fig. 2b) FEG-SEM images display the size and shape of the Pd NPs. It shows the formation of spherical Pd NPs and the particles are in nano region.
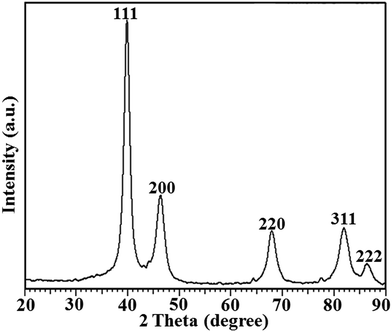 |
| Fig. 1 XRD pattern of Pd NPs. | |
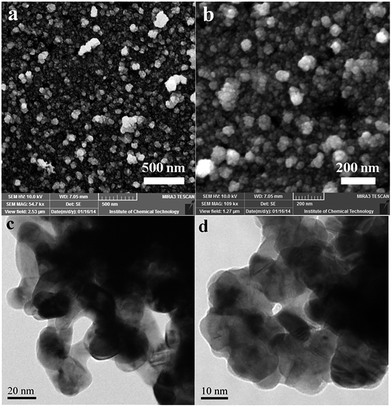 |
| Fig. 2 (a and b) FEG-SEM images (c and d) TEM images of Pd NPs. | |
The TEM analysis was carried out by dispersing powder sample in absolute ethanol and then sonicated for 10 min in sonication bath. The drop of that slurry was deposited on copper grid. The TEM images (Fig. 2c and d) for the Pd NPs displays the formation of spherical NPs having size range 20 nm to 40 nm. PEG-6000 acts as a capping agent in a reaction which interact with Pd metal and helps to get shape and size controlled morphology of nanoparticles. Fig. 3a displays the EDAX spectrum of Pd NPs. It shows only the presence of Pd peaks and no other impurities were observed. XPS analysis describes elemental oxidation state of the metal from the material. XPS spectrum shows the peak at 335.1 eV and 340.4 eV which corresponds to Pd 3d5/2 and Pd 3d3/2 respectively (Fig. 3b). The XPS spectrum confirms that the Pd is in zero Pd(0) state.
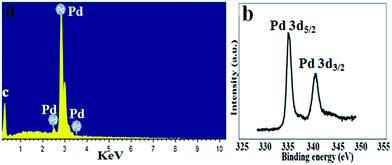 |
| Fig. 3 (a) EDAX spectrum (b) XPS spectrum of Pd 3d5/2 and 3d3/2 regions of Pd NPs. | |
Study of optimization parameters for transfer hydrogenation of nitroarenes
Initially, the various reaction parameters were optimized for the synthesis of aniline from nitrobenzene using Pd NPs catalyst via tandem dehydrogenation of DMAB along with in situ reduction of nitrobenzene (Table 1). Firstly, we have studied the effect of catalyst loading for the reduction of nitrobenzene (Table 1, entries 1–3). It was found that the 6 mol% of Pd NPs catalyst furnished the corresponding aniline in highest yield (Table 1, entry 3). However, when the reaction was performed without catalyst, no product formation was observed which shows the necessity of catalyst (Table 1, entry 4). Next, we have studied the effect of hydrogen donor concentration and it was notice that yield of the product decreases with decrease in concentration of DMAB (Table 1, entries 5 and 6). Furthermore, it was observed that reaction does not work in the absence of DMAB (Table 1, entry 7).
Table 1 Optimization of reaction conditions for transfer hydrogenation of nitroarenesa
During the catalytic process, the hydrogen released from DMAB is, immediately utilized for reduction of starting material. However some hydrogen released by DMAB get liberated to the atmosphere therefore 3 equivalents of DMAB is required to catalyze the reaction (Scheme 2).
 |
| Scheme 2 Pd NPs catalyzed dehydrogenation of DMAB. | |
Subsequently, we have also studied the effect of time and it was found that 10 min is sufficient to get maximum yield of the desired product (Table 1, entries 8 and 9). Thus, the best optimized reaction parameter for tandem dehydrogenation of DMAB and in situ transfer hydrogenation of nitroarenes were; nitroarene (0.5 mmol), DMAB (3 equiv.) and Pd NPs (6 mol%) for 10 min at room temperature (28–30 °C).
We also carried out the caparison study of Pd NPs with Pd/C for transfer hydrogenation of nitrobenzene to aniline. It was observed that Pd NPs provides good yield with high TOF than the Pd/C (Table 2, entries 1 and 2).
Table 2 Catalysts comparisons for transfer hydrogenation of nitroarenesa
Entry |
Catalyst |
Catalyst loading (mol%) |
DMAB (equiv.) |
Time (min) |
Yieldb (%) |
TOF (min−1) |
Reaction conditions: nitrobenzene (0.5 mmol), RT, 3 mL of water/methanol (v/v = 2/1). Yield based on GC analysis. |
1 |
Pd NPs |
6 |
3 |
10 |
99 |
0.825 |
2 |
(10%) Pd/C |
6 |
3 |
10 |
85 |
0.708 |
With optimized conditions in hand, we investigate the general applicability of present protocol for transfer hydrogenation of various nitroarenes using DMAB. The several aromatic nitro compounds were reduced to the respective primary amines in excellent yields within 10–60 min at room temperature (Table 3). Nitrobenzene was reduced into aniline with a yield of >99% in 10 min (Table 3, entry 1). Further, we reduced different alkyl-substituted nitrobenzene such as o-, m- and p-nitrobenzenes to the respective amines in excellent yields (Table 3, entries 2–4). We have also screened chloro and bromo nitroarenes and observed the less yields of desired products along with dehalogenated products (Table 3, entries 5 and 6). The Pd NPs catalyst was also active for the reduction of 2-fluoro nitrobenzene to 2-fluoro aniline in excellent yield without affecting halogen group (Table 3, entry 7). Next, 2-nitro benzyl alcohol and 2-nitroaniline were also reduced giving good yield of desired product (Table 3, entries 8 and 9). Subsequently, we also check present protocol for reduction of m-nitro and p-nitro phenol and it was observed that reaction provided good yield of the desired products within 10 min (Table 3, entries 10 and 11). The 2-nitro-9H-fluorene was reduced giving corresponding product in moderate yield (Table 3, entry 12). Furthermore, we have also extended our protocol for chemoselective reduction of 4-methyl-3-nitrobenzonitrile, 2-(4-nitrophenyl)acetonitrile and interestingly observed that only selective reduction of nitro group into corresponding amines without affecting the cyano group (Table 3, entries 13 and 14).
Table 3 Pd NPs catalyzed transfer hydrogenation various nitroarenes via dehydrogenation of DMABa
Catalyst recyclability study
In order to make our present catalytic system more economically feasible, we have investigated recyclability of Pd NPs for transfer hydrogenation of nitrobenzene to aniline. After completion of reaction, Pd NPs catalyst was separated using centrifugation at 5000 rpm. The catalyst was then washed several times with water and absolute ethanol to remove the rest of organic impurities and dried in oven and reused for next run. The catalyst was efficiently recycled up to four successive cycles without significant loss in its catalytic activity (Fig. 4).
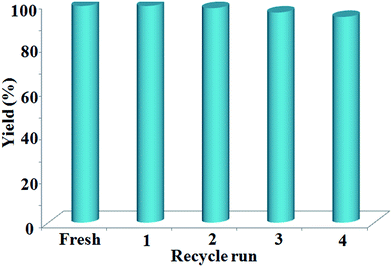 |
| Fig. 4 Recyclability study for Pd NPs. | |
The surface morphological study of fresh nanocatalyst and recycled nanocatalyst was studied with FEG-SEM analysis (Fig. 5). The FEG-SEM image (Fig. 5a) of fresh Pd nanoparticles display the spherical and well dispersed nanoparticles whereas, (Fig. 5b) display FEG-SEM analysis of nanocatalyst after the fourth run. The detail study of recycled catalyst reveals that recycled nanoparticles are more aggregation and disturb the morphology than that of fresh nanoparticles.
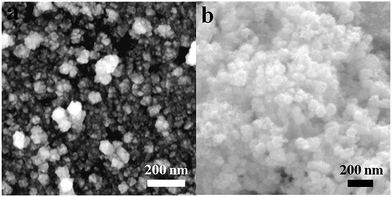 |
| Fig. 5 FEG-SEM images of (a) fresh Pd NPs (b) 4th recycled Pd NPs. | |
Conclusions
In summary, we have developed simple, efficient and greener catalytic system for tandem dehydrogenation of DMAB along with in situ reduction of aromatic nitro compounds into corresponding amines using Pd NPs as a heterogeneous catalyst. The present catalytic system could be easily recycled by simple centrifugation up to four successive cycles without loss in its activity. The current protocol offers several advantages such as (1) reuse of precious metal, (2) tandem dehydrogenation and hydrogenation reaction, (3) eco-friendly and nontoxic hydrogen source, (4) without using high pressure reactor, (5) reaction can perform under ambient condition providing excellent yield within short time and (6) wider substrate applicability with chemoselectivity.
General procedure of Pd NPs catalyzed transfer hydrogenation of nitroarenes
The nitroarene (0.5 mmol) and Pd NPs catalyst (6 mol%) were mixed in 3 mL of water/methanol mixture (v/v = 2/1) in a Schlenk tube at room temperature. Then, dimethylamine-borane (3 equiv.) was added to the above reaction mixture and stirred for 10 min. The progress of reaction was monitored by thin layered chromatography (TLC) and gas chromatography (GC). After completion of the reaction, the catalyst was separated by centrifugation at 5000 rpm and washed several times with distilled water and absolute ethanol. The separated catalyst was dried in oven and reused for next reaction. The product was extracted with DCM (3 × 10 mL) and dried over anhydrous Na2SO4 and evaporated under vacuum to obtain the product. All the obtained products are well known in literature and were confirmed by GC (Perkin Elmer, Clarus 400) (BP-10 GC column, 30 m × 0.32 mm ID, film thickness 0.25 mm), GCMS (Shimadzu GC-MS QP 2010), 1H NMR and 13C NMR spectroscopy.
Characterisation data of products
2-Amino-9H-fluorene. White solid, 52% yield. 1H NMR (400 MHz, CDCl3) δ 7.62 (d, J = 7.6 Hz, 1H), 7.55 (d, J = 8.1 Hz, 1H), 7.45 (d, J = 7.4 Hz, 1H), 7.30 (t, J = 7.4 Hz, 1H), 7.17 (t, J = 7.4 Hz, 1H), 6.87 (s, 1H), 6.70 (d, J = 8.0 Hz, 1H), 3.79 (s, 2H); 13C NMR (101 MHz, CDCl3) δ 145.62, 145.21, 142.35, 142.07, 132.97,1 26.58, 125.04, 124.71, 120.61, 118.55, 113.96, 111.80, 36.79.
3-Amino-4-methylbenzonitrile. Yellow solid, 96% yield, 1H NMR (500 MHz, CDCl3) δ 7.11 (d, J = 7.7 Hz, 1H), 6.98 (dd, J = 7.7, 1.4 Hz, 1H), 6.90 (d, J = 1.4 Hz, 1H), 3.79 (s, 2H), 2.21 (s, 3H); 13C NMR (126 MHz, CDCl3) δ 145.11, 131.01, 127.54, 122.13, 119.39, 117.15, 110.40, 77.25, 77.00, 76.74, 17.65.
2-(4-Aminophenyl)acetonitrile. Orange solid, 97% yield, 1H NMR (400 MHz, CDCl3) δ 7.07 (d, J = 8.5 Hz, 2H), 6.65 (d, J = 8.4 Hz, 2H), 3.70 (s, 2H), 3.60 (s, 2H); 13C NMR (101 MHz, CDCl3) δ 146.18, 128.90, 119.28, 118.42, 115.44, 22.79.
Acknowledgements
Author N. M. Patil thankful to University grand commission (UGC-SAP), New Delhi and author M. B. Bhosale is grateful to Council of Scientific and Industrial Research (CSIR), India for providing fellowship. We are thankful to the Department of Science and Technology (DST), India for financial support for this work under Nano Mission Project No. SR/NM/NS-1097/2011.
Notes and references
-
(a) P. Chen, Z. Xiong, J. Luo, J. Lin and K. L. Tan, Nature, 2002, 420, 302–304 CrossRef CAS PubMed;
(b) W. Grochala and P. P. Edwards, Chem. Rev., 2004, 104, 1283–1316 CrossRef CAS PubMed.
- A. Zuttel, Mater. Today, 2003, 6, 24–33 CrossRef.
-
(a) J. L. C. Rowsell, A. R. Millward, K. S. Park and O. M. Yaghi, J. Am. Chem. Soc., 2004, 126, 5666–5667 CrossRef CAS PubMed;
(b) N. L. Rosi, J. Eckert, M. Eddaoudi, D. T. Vodak, J. Kim, M. O’Keeffe and O. M. Yaghi, Science, 2003, 300, 1127–1129 CrossRef CAS PubMed.
- S.-I. Orimo, Y. Nakamori, J. R. Eliseo, A. Zuttel and C. M. Jensen, Chem. Rev., 2007, 107, 4111–4132 CrossRef CAS PubMed.
- G. A. Deluga, J. R. Salge, L. D. Schmidt and X. E. Verykios, Science, 2004, 303, 993–997 CrossRef CAS PubMed.
-
(a) R. J. Keaton, J. M. Blacquiere and R. T. Baker, J. Am. Chem. Soc., 2007, 129, 1844–1845 CrossRef CAS PubMed;
(b) A. Staubitz, A. P. M. Robertson and I. Manners, Chem. Rev., 2010, 110, 4079–4124 CrossRef CAS PubMed.
- H. Dorn, J. M. Rodezno, B. Brunnhofer, E. Rivard, J. A. Massey and I. Manners, Macromolecules, 2003, 36, 291–297 CrossRef CAS.
- A. Staubitz, P. A. Soto and I. Manners, Angew. Chem., 2008, 120, 6308–6311 CrossRef PubMed.
- Y. Jiang and H. Berke, Chem. Commun., 2007, 34, 3571–3573 RSC.
-
(a) M. Couturier, B. M. Andresena, J. L. Tuckera, P. Dubea, S. J. Breneka and J. T. Negria, Tetrahedron Lett., 2001, 42, 2763–2766 CrossRef CAS;
(b) C. A. Jaska and I. Manners, J. Am. Chem. Soc., 2004, 126, 2698–2699 CrossRef CAS PubMed.
- C. A. Jaska, K. Temple, A. J. Lough and I. Manners, J. Am. Chem. Soc., 2003, 125, 9424–9434 CrossRef CAS PubMed.
-
(a) Y. Chen, J. L. Fulton, J. C. Linehan and T. Autrey, J. Am. Chem. Soc., 2005, 127, 3254–3255 CrossRef CAS PubMed;
(b) C. A. Jaska and I. Manners, J. Am. Chem. Soc., 2004, 126, 9776–9785 CrossRef CAS PubMed.
- Y. Jiang and H. Berke, Chem. Commun., 2007, 48, 3571–3573 RSC.
- D. Pun, E. Lobkovsky and P. J. Chirik, Chem. Commun., 2007, 48, 3297–3299 RSC.
- T. J. Clark, C. A. Russell and I. Manners, J. Am. Chem. Soc., 2006, 128, 9582–9583 CrossRef CAS PubMed.
-
(a) T. D. Nixon, M. K. Whittlesey and J. M. J. Williams, Tetrahedron Lett., 2011, 52, 6652–6654 CrossRef CAS PubMed;
(b) D. van der Waals, A. Pettmanb and J. M. J. Williams, RSC Adv., 2014, 4, 51845–51849 RSC.
-
(a) S. Gomez, J. A. Peters and T. Maschmeyer, Adv. Synth. Catal., 2002, 344, 1037–1057 CrossRef CAS;
(b) H. U. Blaser, H. Steiner and M. Studer, ChemCatChem, 2009, 1, 210–221 CrossRef CAS PubMed.
-
(a) F. A. Westerhaus, R. V. Jagadeesh, G. Wienhofer, M.-M. Pohl, J. Radnik, A.-E. Surkus, J. Rabeah, K. Junge, H. Junge, M. Nielsen, A. Brückner and M. Beller, Nat. Chem., 2013, 5, 537–543 CrossRef CAS PubMed;
(b) R. V. Jagadeesh, A. E. Surkus, H. Junge, M. M. Pohl, J. Radnik, J. Rabeah, H. M. Huan, V. Schunemann, A. Bruckner and M. Beller, Science, 2013, 342, 1073–1076 CrossRef CAS PubMed.
-
(a) E. Vasilikogiannaki, I. Titilas, G. Vassilikogiannakis and M. Stratakis, Chem. Commun., 2015, 51, 2384–2387 RSC;
(b) M. Gulcan, M. Zahmakiran and S. Ozkar, Appl. Catal., B, 2014, 147, 394–401 CrossRef CAS PubMed;
(c) D. Ozhava, N. Z. Kilicaslan and S. Ozkar, Appl. Catal., B, 2015, 162, 573–582 CrossRef CAS PubMed;
(d) L. Shi, Y. Liu, Q. Liu, B. Wei and G. Zhang, Green Chem., 2012, 14, 1372–1375 RSC;
(e) Q. Yang, Y.-Z. Chen, Z. U. Wang, Q. Xu and H.-L. Jiang, Chem. Commun., 2015, 51, 10419–10422 RSC.
- E. Vasilikogiannaki, C. Gryparis, V. Kotzabasaki, I. N. Lykakis and M. Stratakis, Adv. Synth. Catal., 2013, 355, 907–911 CrossRef CAS PubMed.
-
(a) H. Goksu, S. F. Ho, O. Metin, K. Korkmaz, A. M. Garcia, M. S. Gultekin and S. Sun, ACS Catal., 2014, 4, 1777–1782 CrossRef CAS;
(b) H. Goksu, H. Can, K. Sendil, M. S. Gultekin and O. Metin, Appl. Catal., A, 2014, 488, 176–182 CrossRef CAS PubMed.
- M. Yurderi, A. Bulut, M. Zahmakiran, M. Gulcan and S. Ozkar, Appl. Catal., B, 2014, 160–161, 534–541 CrossRef CAS PubMed.
-
(a) D. Astruc, F. Lu and J. R. Aranzaes, Angew. Chem., Int. Ed., 2005, 44, 7852–7872 CrossRef CAS PubMed;
(b) M. A. Bhosale, T. Sasaki and B. M. Bhanage, Catal. Sci. Technol., 2014, 4, 4274–4280 RSC;
(c) M. A. Bhosale and B. M. Bhanage, RSC Adv., 2014, 4, 15122–15130 RSC.
-
(a) N. M. Patil, T. Sasaki and B. M. Bhanage, Catal. Lett., 2014, 144, 1803–1809 CrossRef CAS;
(b) N. M. Patil and B. M. Bhanage, Catal. Today, 2015, 247, 182–189 CrossRef CAS PubMed;
(c) D. B. Bagal, Z. S. Qureshi, K. P. Dhake, S. R. Khan and B. M. Bhanage, Green Chem., 2011, 13, 1490–1494 RSC.
- S. C. Raghu, M. Ulaganathan, V. Aravindan and T. M. Lim, ChemPhysChem, 2013, 14, 3887–3890 CrossRef CAS PubMed.
- M. T. Amin, A. A. Alazba and U. Manzoor, Adv. Mater. Sci. Eng., 2014, 825910 Search PubMed.
- H. Chen, G. Wei, A. Ispas, S. G. Hickey and A. Eychmuller, J. Phys. Chem. C, 2010, 114, 21976–21981 CAS.
- P. J. Cappillino, J. D. Sugar, M. A. Hekmaty, B. W. Jacobs, V. Stavila, P. G. Kotula, J. M. Chames, N. Y. Yanga and D. B. Robinson, J. Mater. Chem., 2012, 22, 14013–14022 RSC.
- A. B. Patil, D. S. Patil and B. M. Bhanage, J. Mol. Catal. A: Chem., 2012, 365, 146–153 CrossRef CAS PubMed.
- I. Saldan, Y. Semenyuk, I. Marchuk and O. Reshetnyak, J. Mater. Sci., 2015, 50, 2337–2354 CrossRef CAS.
- A. Kameo, T. Yoshimura and K. Esumi, Colloids Surf., A, 2003, 215, 181–189 CrossRef CAS.
- A. Nemamcha, J.-L. Rehspringer and D. Khatmi, J. Phys. Chem. B, 2006, 110, 383–387 CrossRef CAS PubMed.
- M. Yun, N. V. Myung, R. P. Vasquez, C. Lee, E. Menke and R. M. Penner, Nano Lett., 2004, 4, 419–422 CrossRef CAS.
- A. Bankar, B. Joshi, A. R. Kumar and S. Zinjarde, Mater. Lett., 2010, 64, 1951–1953 CrossRef CAS PubMed.
- D. H. Nagaraju, S. Devaraj and P. Balaya, Mater. Res. Bull., 2014, 60, 150–157 CrossRef CAS PubMed.
- R. J. Kalbasi and N. Mosaddegh, J. Solid State Chem., 2011, 184, 3095–3103 CrossRef CAS PubMed.
- Y. Tang, Y. Cao, S. Wang, G. Shen and R. Yu, Sens. Actuators, B, 2009, 137, 736–740 CrossRef CAS PubMed.
Footnote |
† Electronic supplementary information (ESI) available: General reaction procedure for synthesis of Pd NPs and transfer hydrogenation reaction, 1H NMR, 13C NMR and GC-MS data of products. See DOI: 10.1039/c5ra14944d |
|
This journal is © The Royal Society of Chemistry 2015 |