DOI:
10.1039/C5RA14637B
(Paper)
RSC Adv., 2015,
5, 87400-87410
Amide functionalized metal–organic frameworks for diastereoselective nitroaldol (Henry) reaction in aqueous medium†
Received
23rd July 2015
, Accepted 29th September 2015
First published on 2nd October 2015
Abstract
The two new metal–organic frameworks (MOFs) of zinc(II) and copper(II) [Zn2L2(1,4-BDC)]n·2n(DMF) (1) and [Cu(L)2]n·4n(DMF)·n(H2O) (2) (L = 4-(pyridin-4-ylcarbamoyl)benzoate; BDC = benzenedicarboxylate), respectively, have been synthesized from a pyridyl amide functionalized benzoic acid (HL). They were characterized by elemental, FT-IR, thermogravimetric, powder X-ray and single crystal X-ray diffraction analyses. Single crystal X-ray crystallography reveals that 1 and 2 exhibit 2D and 3D polymeric architectures, respectively. Topological analysis illustrates a 6-connected pcu alpha-Po primitive cubic topology for 1 and an 8-connected body centred cubic (bcu) topology for 2. Both 1 and 2 act as heterogeneous catalysts for the nitroaldol reaction in aqueous medium, with a high yield and moderate to good diastereoselectivities under ambient conditions. They can be recycled and reused without any significant loss of the catalytic efficiency.
Introduction
The design and construction of metal–organic frameworks (MOFs),1 also known as coordination polymers (CPs), have attracted enormous attention owing, e.g., to their simplistic preparation, structural diversity, tunable pore metrics and easy functionalization of their surfaces. As a result, MOFs have found widespread applications in storage of fuel and environmental gases,2,3 molecular separation,4 luminescence,5 magnetism,6 drug delivery,7 biomedical imaging8 and sensing.9 Recently, MOFs have found applications in the field of catalysis,10–15 where their high density of active sites and high porosity can be advantageous features. Usually, the catalytic activity of MOFs is dependent, e.g., on the Lewis acidity of the metal cations,12 the presence of basic groups13,14 (such as pyridine, amide and amine) in constructing linkers and the redox properties of the metal centres.10,11,15
Thus, in pursuing our interest on the synthesis of MOFs for possible application in catalysis,10–14 we chose 4-(pyridin-4-ylcarbamoyl)benzoic acid (HL) as a ligand source (Fig. 1), based on the following reasons: (i) the amide backbone in the ligand framework may provide a basic environment which may facilitate the catalytic reaction;13b,16 (ii) the ligand comprises multiple coordinating groups which should be suitable for building extended frameworks with a large surface area, also favourable for catalysis.
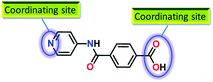 |
| Fig. 1 4-(Pyridin-4-ylcarbamoyl)benzoic acid (HL). | |
Concerning a possible catalytic application, the nitroaldol (or Henry) reaction is as an important carbon–carbon bond formation reaction and widely used in organic synthesis.11,17–20 The resulting α-hydroxy nitro compounds are valuable starting materials for, e.g., 1,2-amino alcohols or β-hydroxy acids.21 Generally, such a reaction is performed with homogeneous basic catalysts, such as alkali metal hydroxides, alkoxides or amines, with a good efficiency.22 A number of homogeneous catalysts have already been reported by our group for such a type of reaction.10a,11,23
However, heterogeneous catalysts for such a transformation are scant,10a,11d–g,24–28 and thus we wish to extend this topic. On the other hand, organic reactions in water have attracted a significant attention because of the natural abundance and non-toxicity of this medium as compared to typical organic solvents.29,30 Recently, a few mononuclear copper(II) complexes were reported by our group11d to act as heterogeneous catalysts in water towards the nitroaldol reaction, but the obtained yield (77%) should be improved. Thus, the development of new, efficient and selective heterogeneous catalysts in aqueous medium would be a significant addition to this field and are worth exploring. Further, to our knowledge there are no reports on Zn(II) or Cu(II) MOF catalysts, where the Henry reaction has been performed in aqueous medium.
Hence, in quest for developing efficient heterogeneous catalysts for the Henry reaction in aqueous medium, herein we present the syntheses and crystal structures of two new MOFs with a bridged amide pyridyl benzoate (L−, Fig. 1), viz. [Zn2L2(1,4-BDC)]n·2n(DMF) (1) (BDC = benzenedicarboxylate) and [Cu(L)2]n·4n(DMF)·n(H2O) (2), and their catalytic application in such a reaction. The structural features of the obtained MOFs have been established by single crystal X-ray diffraction, topological and thermogravimetric studies.
Experimental section
Materials and physical measurements
Solvents were dried and distilled prior to their use following standard procedures.31 4-Aminopyridine, terephthalic acid (H2DA), thionyl chloride (SOCl2) and the metal nitrates Zn(NO)3·6H2O (98.0% purity) and Cu(NO3)2·3H2O were purchased from Sigma Aldrich Chemical Co. and used as received. Methyl-4-(chlorocarbonyl)benzoate was prepared following established literature procedures with slight modifications.32 FT-IR spectra were recorded on a Bruker Vertex 70 instrument in KBr pellets. 1H (300 MHz) and 13C (75.45 MHz) NMR spectra were obtained at room temperature (RT) on a Bruker Avance II + 300 (UltraShield™ Magnet) spectrometer using tetramethylsilane [Si(CH3)4] as an internal reference. Carbon, hydrogen and nitrogen elemental analyses were carried out by the Microanalytical Service of the Instituto Superior Técnico. Powder X-ray diffraction (PXRD) was conducted in a D8 Advance Bruker AXS (Bragg Brentano geometry) theta–2-theta diffractometer, with copper radiation (Cu Kα, λ = 1.5406 Å) and a secondary monochromator, operated at 40 kV and 40 mA. Flat plate configuration was used and the typical data collection range was between 5° and 40°.
Synthesis of 4-(pyridin-4-ylcarbamoyl)benzoic acid (HL)
The synthesis of the ligand (HL) involves a five-step reaction sequence. The first three-steps concern, the synthesis of monomethyl ester chloride from terephthalic acid, used in the synthesis of the HL, and are described in the Experimental section of ESI.† The relevant 1H and 13C NMR spectra are presented in Fig. S1–S6, ESI.†
In the fourth step, a solution of 4-aminopyridine (0.5 g, 5.3 mmol) and distilled triethylamine (3.0 mL, 28 mmol) in anhydrous THF (100 mL) was added dropwise to a solution of methyl 4-(chlorocarbonyl)benzoate (1 g, 5 mmol) in anhydrous THF (100 mL) at room temperature. Triethylamine (3.0 mL, 28 mmol) was added, and the reaction mixture was stirred for 24 h. The resulting white product of methyl 4-(pyridin-4-ylcarbamoyl)benzoate thus formed was collected by filtration, washed with THF and several times with water, and dried under vacuum to get the desired methyl ester MeL (1.2 g, 80%). 1H-NMR (300 MHz, CDCl3, δ ppm): δ 10.79 (s, 1H, NH), 8.50 (d, 2H, J = 6.3 Hz, Hpy), 8.10 (d, 2H, J = 9.0 Hz, HAr), 8.07 (d, 2H, J = 9.0 Hz, Hpy), 7.79 (d, 2H, J = 6.0 Hz, HAr), 3.90 (s, 3H, –O–CH3). 13C-NMR (75.45 MHz, CDCl3, δ ppm): 167.94, 165.62, 136.12, 135.20, 133.86, 132.56, 131.46, 129.40, 52.78 (–O–CH3) ESI-MS: m/z [(M + H)]+, calcd 243.23, found 243.1.
Finally, the isolated MeL (1 g, 4 mmol) and NaOH (0.5 g, 13 mmol) were dissolved in 20 mL of MeOH
:
H2O (4
:
1). The resultant reaction mixture was refluxed for 6 h at 80 °C, after which the solvent was reduced nearly to dryness under reduced pressure and the solution was acidified (pH ∼ 1–2) with dilute HCl solution. The white solid product of HL thus obtained was removed by filtration, washed several times with water until neutral and dried under vacuum (yield: 0.6 g, 60%). Anal. calcd for C13H10N2O3: C, 64.46; H, 4.16; N, 11.56. Found: C, 64.72; H, 4.32; N, 11.43. FT-IR (KBr, cm−1): 3383 (bs), 2547 (mb), 1691 (s), 1590 (s), 1507 (s), 1378 (s), 1320 (m), 1196 (m), 1109 (w), 1057 (w), 961 (s), 759 (s), 883 (s), 730 (s), 528 (w); 1H-NMR (300 MHz, DMSO-d6, δ ppm): δ 10.80 (s, 1H, NH), 8.52 (d, 2H, J = 6.0 Hz, Hpy), 8.10 (d, 2H, J = 6.0 Hz, HAr), 8.04 (d, 2H, J = 9.0 Hz, Hpy), 7.83 (d, 2H, J = 6.0 Hz, HAr). 13C-NMR (77 MHz, DMSO-d6, δ ppm): 167.15, 166.14, 160.50, 150.58, 146.20, 137.91, 137.21, 134.30, 129.93, 128.62. ESI-MS: m/z [(M + H)]+, calcd 243.07, found 243.1, [(M + Na)]+, calcd 265.07, found 265.1.
Synthesis of [Zn2(L)2(1,4-BDC)]n·2n(DMF) (1)
Zn(NO3)2·6H2O (6.6 mg, 0.022 mmol), HL (5 mg, 0.022 mmol) and terephthalic acid (5 mg, 0.022 mmol) were placed in H2O (1 mL) and DMF (1 mL) in a sealed 8 mL glass vessel and heated at 80 °C for 48 h. White crystals of 1 were obtained upon gradual cooling to room temperature (at 0.2 °C min−1), washed with deionized water, DMF and then dried in air. 1 is not soluble in the usual solvents. Yield: 70% (based on Zn). Anal. calcd for C40H36N6O12Zn2: C, 52.01; H, 3.93; N, 9.10. Found: C, 51.99; H, 3.91; N, 9.06. IR (KBr/pellet, cm−1): 3080 (w, br), 1666 (w), 1596 (s), 1516 (w), 1428 (s), 1388 (s), 1331 (w), 1296 (m, sh), 1210 (m), 1095 (w), 1024 (m), 897 (m), 841 (m), 732 (s, br), 573 (m), 538 (m).
Synthesis of [Cu(L)2]n·4n(DMF)·n(H2O) (2)
A similar synthetic procedure as that used for 1 was followed, but using HL (15 mg, 0.067 mmol) and Cu(NO3)2·3H2O (10 mg, 0.060 mmol) in DMF
:
H2O (1 mL
:
1 mL), without terephthalic acid. The blue block crystals of 2 suitable for X-ray diffraction analysis obtained were subsequently washed with water, DMF and dried in air. 2 is not soluble in the usual solvents. Yield: 60% (based on Cu). Anal. calcd for C41H54CuN9O11.5 [obtained from a batch different of that used for X-ray analysis and fitting to [Cu(L)2]n·5n(DMF)·0.5n(H2O)]: C, 53.97; H, 5.97; N, 13.81. Found: C, 53.93; H, 5.92; N, 13.78. IR (KBr/pellet, cm−1): 3426 (w, br), 1678 (w), 1595 (w), 1518 (w), 1386 (s), 1334 (w), 1297 (m), 1267 (m), 1213 (s), 1099 (w), 1026 (m), 875 (m), 836 (m), 732 (s, br), 538 (m), 471 (m).
Procedure for nitroaldol (Henry) reaction catalyzed by the MOFs 1 and 2
In a typical reaction, a mixture of an aldehyde (1 mmol), nitroethane (4 mmol) and the Zn- or the Cu-MOF catalyst (3.0 mol%) was placed in a capped glass vessel, and then 2.0 mL water was added into it. The mixture was heated at 70 °C for 48 h, and subsequently quenched by centrifugation and filtration at room temperature. The filtrate was extracted with dichloromethane. The organic extracts were collected over anhydrous sodium sulfate; subsequent evaporation of the solvent gave the crude product. The product was dissolved in CDCl3 and analyzed using 1H NMR. The yield of the β-nitroalkanol product (relative to the aldehyde) was established typically by taking into consideration the relative amounts of these compounds, as given by 1H NMR and as previously reported.11d–g The ratio between the syn and anti isomers was also determined by 1H NMR spectroscopy. In the 1H NMR spectra, the values of vicinal coupling constants (for the β-nitroalkanol products) between the α-N–C–H and the α-O–C–H protons identify the isomers, being J = 7–9 or 3.2–4 Hz for the syn or anti isomers, respectively.11d–g The yield and selectivity of MOFs 1 and 2 for the Henry reaction was calculated (see Fig. S12 and S13, ESI,† as examples) according to a procedure reported earlier on the basis of 1H-NMR spectra.11e
The catalytic recycling experiment was performed by washing the used catalyst (separated by centrifugation and filtration of the supernatant solution) with water and dried in air at room temperature. It was then subsequently reused for the nitro-aldol (Henry) reaction as described above.
Crystal structure determination
X-ray quality single crystals of the compounds were immersed in cryo-oil, mounted in a nylon loop and measured at room temperature (1) or at 150 K (2). Intensity data were collected using a Bruker AXS-KAPPA APEX II or a Bruker APEX-II PHOTON 100 diffractometer with graphite monochromated Mo-Kα (λ 0.71069) radiation. Data were collected using phi and omega scans of 0.5° per frame and a full sphere of data was obtained. Cell parameters were retrieved using Bruker SMART33a software and refined using Bruker SAINT33a on all the observed reflections. Absorption corrections were applied using SADABS.33a Structures were solved by direct methods by using SIR97 (ref. 33b) and refined with SHELXL-2014/7.33c Calculations were performed using the WinGX System-Version 2014.1,33d and molecular graphics were done with Mercury 3.5.1.33e The hydrogen atoms attached to carbon atoms were inserted at geometrically calculated positions and included in the refinement using the riding-model approximation; Uiso(H) were defined as 1.2Ueq of the parent carbon atoms for phenyl and methylene residues and 1.5Ueq for methyl groups. The hydrogen atoms bound to nitrogen were located from the final difference Fourier map and their positions refined with DFIX restraints, and with Uiso = 1.5Ueq of their parent N-atoms. For the final refinements of structure 1, the TWIN/BASF instructions were included in the SHELXL2014 instruction file, in order to take into consideration the contribution of two twin components to the structure-factor calculations during the least-squares optimization; the major twin fraction refined to 0.499(15). The Platon SQUEEZE procedure33e was applied to compound 2 to recover 538 electrons per unit cell in a void (total volume 1445 Å3), that is, 135 electrons per formula unit. The electron count suggests the presence of ca. one water and three dimethylformamide molecules per asymmetric unit. These were removed from the crystal structures, but included in the empirical formula of CIF. The thermogravimetric analysis and the elemental analysis also support these results. Least square refinements with anisotropic thermal motion parameters for all the non-hydrogen atoms and isotropic for the remaining atoms were employed. Crystallographic data are summarized in Table 1 and selected bond distances and angles are presented in Tables S1 and S2, ESI.† CCDC 1414611 and 1414612 for 1 and 2, respectively, contain the ESI crystallographic data for this paper.†
Table 1 Crystal data and structure refinement details for compounds 1 and 2
Identification name |
1 |
2 |
Formulae |
C40H36N6O12Zn2 |
C38H48CuN8O11 |
Mol. wt |
923.49 |
856.38 |
Crystal system |
Monoclinic |
Monoclinic |
Space group |
P21 |
P21/n |
Temperature/K |
296(2) |
150(2) |
Wavelength/Å |
0.71073 |
0.71073 |
a/Å |
8.0350(9) |
8.3259(14) |
b/Å |
24.892(3) |
22.773(4) |
c/Å |
10.8856(13) |
20.105(3) |
α/° |
90 |
90 |
β/° |
111.560(4) |
93.700(5) |
γ/° |
90 |
90 |
V/Å3 |
2024.9(4) |
3804.1(11) |
Z |
2 |
4 |
Density/Mg m−3 |
1.515 |
1.495 |
Abs. coeff./mm−1 |
1.255 |
0.648 |
F(000) |
948 |
1796.0 |
Refl. collected |
46 996 |
43 898 |
Refl. unique |
7404 |
6972 |
Max. 2θ/° |
25.372 |
25.40 |
Ranges (h, k, l) |
−9 ≤ h ≤ 9 |
−10 ≤ h ≤ 10 |
−30 ≤ k ≤ 30 |
−27 ≤ k ≤ 27 |
−13 ≤ l ≤ 13 |
−24 ≤ l ≤ 24 |
Complete to 2θ (%) |
99.8 |
98.5 |
Refl. with I > 2σ(I) |
7306 |
4604 |
Data/restraints/parameters |
7404/1/552 |
6972/80/360 |
Goof (F2) |
1.109 |
1.057 |
R1 [I > 2s(I)] |
0.0318 |
0.0834 |
wR2 [I > 2s(I)] |
0.0795 |
0.2110 |
R1 [all data] |
0.0322 |
0.1243 |
wR2 [all data] |
0.0801 |
0.2268 |
Results and discussion
Synthesis and characterization
The ligand 4-(pyridin-4-ylcarbamoyl)benzoic acid (HL) was synthesised (Scheme 1) by reacting 4-aminopyridine with methyl 4-(chlorocarbonyl)benzoate in anhydrous THF in the presence of triethylamine, followed by hydrolysis according to a literature procedure.11g It was characterized by elemental, IR, 1H and 13C NMR and ESI-MS analyses. The 1H and 13C NMR spectra are presented in Fig. S7–S10, ESI.† Compounds 1 and 2 were synthesized (Scheme 1) by hydrothermal reaction of HL with Zn(NO3)2·6H2O and Cu(NO3)2·3H2O, respectively, in the former case in the presence of terephthalic acid (H2DA), and were characterized by elemental analysis, IR, single crystal X-ray crystallography, powder X-ray diffraction and thermogravimetric analyses (Fig. S11, ESI†). They are insoluble in water and in common organic solvents.
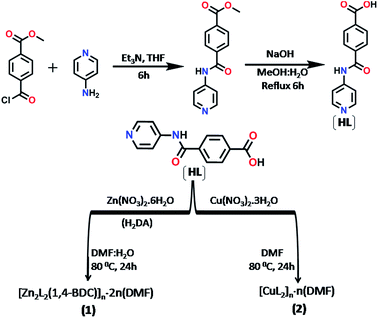 |
| Scheme 1 Syntheses of HL, zinc(II) (1) and copper(II) (2) MOFs. | |
Their infrared spectra (Fig. S12, ESI†) support the metal coordination of the carboxylate group of L−. The band due to the asymmetric stretching vibration [νasym(OCO)] exhibits a shift of ca. 100 cm−1 relative to the free ligand HL (1691 cm−1), being observed at 1596 cm−1 and 1595 cm−1 for 1 and 2, respectively. The coordination of the carboxylate group is confirmed by X-ray crystal structure determination as discussed below.
Crystal structure analysis
Single-crystal X-ray diffraction studies of 1 revealed that it crystallizes in the monoclinic P21 space group, and that the asymmetric unit contains two Zn2+ ions, two deprotonated ligands L−, one benzenedicarboxylate and two non-coordinated DMF molecules [Fig. 2(A) and (B)]. The L− and 1,4-benzenedicarboxylate ligands coordinate to the metal centres in a bridging bidentate fashion, the former remaining almost planar as indicated by the angle between the least square planes of the phenyl and of the piridyl rings (ca. 10.13°). Compound 1 features a rhombic grid type three-dimensional framework having approximately 11 × 15 Å2 channels along the crystallographic b-axis [Fig. 2(C)] and with 3-fold interpenetrating networks [Fig. 4(C)]. The dinuclear zinc(II) paddle-wheel clusters act as secondary building blocks through four L− and two 1,4-benzenedicarboxylate ligands. Both the Zn(II) centres adopt square pyramidal geometries (τ5 of 0.00).34 The equatorial planes of the metal ions are occupied by four O-atoms, two from L− and other two from 1,4-benzenedicarboxylate ligands; the axial site is engaged with the pyridyl N-atom. The Zn–O bond lengths are in the range of 2.019(3)–2.084(4) Å and the Zn–N distances lie between 2.010(5) and 2.025(4) Å. The shortest metal⋯metal distance in 1 is of 2.9936(5) Å.
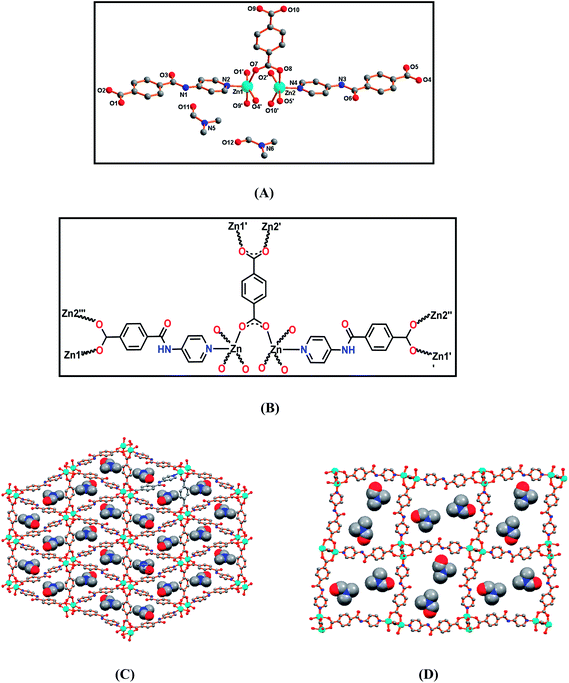 |
| Fig. 2 (A) Asymmetric unit of 1 with partial atom labelling scheme; (B) schematic representation of an asymmetric unit of 1 (excluding DMF molecules); representation of the 3D network of 1 along the crystallographic a-axis (C) and b-axis (D) (DMF molecules are represented as spacefill model). Hydrogen atoms are omitted for clarity. | |
The DMF molecules are encapsulated in the lattice [Fig. 2(D)] and involved in hydrogen bonding interactions connecting the amide NH– moiety as donor and the oxygen from DMF as acceptor [N4–H4N⋯O20, D⋯A 2.985(8) Å, <DHA 157(5)°; N6–H6N⋯O21, D⋯A 2.980(9) Å, <DHA 164(5)°]. Several C–H⋯O interactions are also relevant and help to stabilize the lattice (Table S2, ESI†).
Compound [Cu(L)2]n·4n(DMF)·n(H2O) (2) crystallizes in the monoclinic P21/n space group, its asymmetric unit containing one Cu2+ ion, two deprotonated L− ligands and one non-coordinated DMF molecule [Fig. 3(A) and (B)]. The dimetallic cluster in 2 acts as a secondary building block through eight L− ligands [Fig. 3(C)]. The Cu(II) centre presents a distorted square pyramidal geometry (τ5 = 0.32),35 the equatorial plane including two O-atoms and two N-atoms, whereas the axial site is occupied by one carboxylate O-atom. The Cu–O bond lengths are in the 1.961(3)–2.342(4) Å range and the Cu–N distances assume values of 2.004(4) and 2.020(4) Å. The minimum metal⋯metal distance is of 4.340(1) Å.
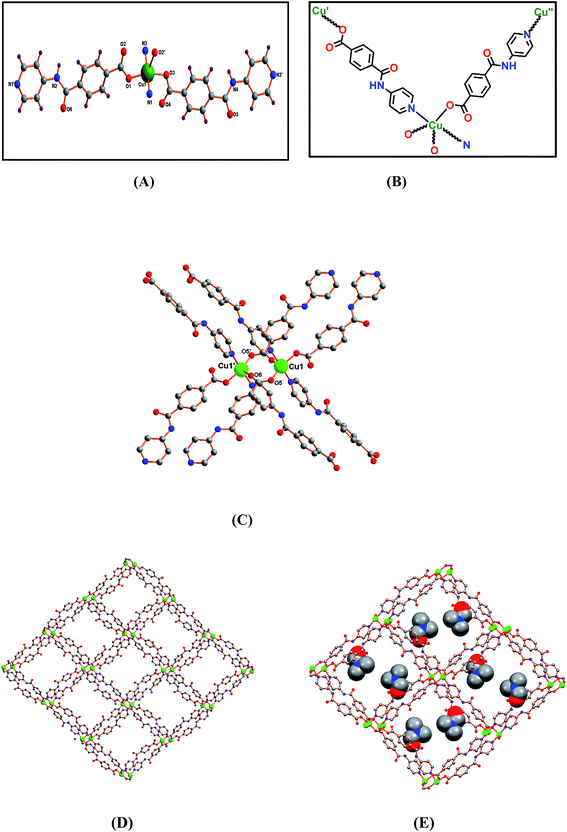 |
| Fig. 3 (A) Asymmetric unit of 2 with partial atom labelling scheme; (B) schematic representation of the asymmetric unit of 2 (excluding DMF molecules); (C) representation of the 3D network of 2; (D) 3D packing diagram of 2; (E) 3D packing diagram of 2 (DMF molecules are represented as space fill model). Hydrogen atoms are omitted for clarity. | |
The packing view of 2 is characterized by open channels along the crystallographic a-axis [Fig. 3(D)] with approximate dimension of 15 × 15 Å2 and a void space of 34% per unit cell according to PLATON.33e The open channels are occupied by DMF molecules which are hydrogen bonded through the amide hydrogen atoms to the network [N2–H2N⋯O8, D⋯A 2.943(6) Å, <DHA 171(4)°] [Fig. 3(E)]. Intermolecular C–H⋯O contacts are relevant and help to stabilize the structure (Table S2, ESI†).
Topological analysis
To improve the description of the crystal structures of 1 and 2 their topological analysis was performed36 by reducing their multidimensional structures to simple node-and-linker nets where the metallic nodes and the organic linkers represent secondary building units (SBUs).36a,b The entire lattice and coordinated solvent molecules were first removed from the frameworks. In case of 1, the dinuclear zinc(II) unit was considered as a single node coordinated to two L and four 1,4-benzenedicarboxylate anions, thus affording a 6-connected pcu alpha-Po primitive cubic topology [Fig. 4(A)]. This network also has threefold interpenetrated nets as presented in Fig. 4(B). In case of 2, where each Cu1 centre is coordinated to four different carboxylate ligands, the dinuclear unit was considered as a single node which subsequently coordinated via 8 different carboxylate linkers. As a result, the framework represents an 8-connected body centred cubic (bcu) topology [Fig. 4(C)].
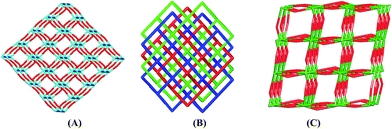 |
| Fig. 4 Node-and-linker-type descriptions of the 2D coordination frameworks in 1 (A), of the 3D coordination frameworks in 2 (B), and the three fold interpenetrated network of 1 (C). The metal nodes are represented in green (Cu) and cyan (Zn) and the linkers in red. | |
Catalytic activity of MOFs 1 and 2 for the nitro-aldol reaction
In view of the insolubility of the frameworks 1 and 2 in water, we have tested them as solid heterogeneous catalysts for the nitroaldol (or Henry) reaction in water of nitroethane with various aldehydes (Scheme 2). In a typical reaction, a mixture of aldehyde (1.0 mmol), nitroethane (0.3 mL, 4.0 mmol) and 1 or 2 (3.0 mol%) in 2.0 mL water, contained in a capped glass vessel, was stirred at 70 °C for 48 h, whereupon the solution was filtered or centrifuged to remove the solid catalyst. The filtrate was extracted with dichloromethane. The organic extracts were collected over anhydrous sodium sulfate; subsequent evaporation of the solvent gave the crude product which was a mixture of the β-nitroalkanol diastereoisomers (syn and anti forms, with predominance of the former; Scheme 2) which were analyzed by 1H NMR (Fig. S13 and S14, ESI†).
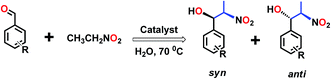 |
| Scheme 2 Nitroaldol (Henry) reaction of benzaldehydes and nitroethane, with 1 or 2 as catalyst. | |
To begin with, MOFs 1 and 2 were screened as catalysts with p-nitrobenzaldehyde and nitroethane as representative substrates; we found that 1 showed a slightly higher catalytic activity than 2 under similar experimental conditions. This eventually could be accounted for by the higher Lewis acidic nature of Zn(II). Hence, the optimization of the reaction conditions with reaction time (2–60 h), catalyst loading (1–7 mol%) and temperature (22–80 °C) was investigated in a model p-nitrobenzaldehyde and nitroethane system with 1 as catalyst (Table 2).
Table 2 Optimization of the parameters of the nitro-aldol reaction between p-nitrobenzaldehyde and nitroethane with 1 as catalysta
Entry |
Catalyst |
Time (h) |
Amount of catalyst (mol%) |
T (°C) |
Solvent |
Yieldb (%) |
Selectivityc (syn/anti) |
TONd |
Reaction conditions: 3.0 mol% of catalyst 1 or 2 (mole corresponds to the repeating formula unit), benzaldehyde (1.0 mmol), nitroethane (0.3 mL, 4.0 mmol) and water (2.0 mL) unless stated otherwise. Number of moles of β-nitroalkanol per 100 moles of aldehyde. Calculated by 1H NMR. Number of moles of β-nitroalkanol per mole of catalyst. |
1 |
1 |
48 |
3 |
70 |
H2O |
93 |
74 : 26 |
31 |
2 |
2 |
48 |
3 |
70 |
H2O |
90 |
65 : 35 |
30 |
3 |
1 |
48 |
3 |
70 |
CH3CN |
10 |
73 : 27 |
3.3 |
4 |
1 |
48 |
3 |
70 |
THF |
25 |
74 : 26 |
8.3 |
5 |
1 |
48 |
3 |
70 |
MeOH |
75 |
75 : 25 |
25 |
6 |
1 |
48 |
3 |
70 |
H2O |
93 |
74 : 26 |
31 |
7 |
Blank |
48 |
— |
70 |
H2O |
11 |
70 : 30 |
3.7 |
8 |
Zn(NO3)2·6H2O |
48 |
3 |
70 |
H2O |
18 |
82 : 18 |
6 |
9 |
Cu(NO3)2·3H2O |
48 |
3 |
70 |
H2O |
22 |
81 : 19 |
7.3 |
10 |
HL |
48 |
3 |
70 |
H2O |
28 |
78 : 22 |
9.3 |
11 |
1 |
48 |
1 |
70 |
H2O |
44 |
78 : 22 |
44 |
12 |
1 |
48 |
5 |
70 |
H2O |
92 |
74 : 26 |
18.4 |
13 |
1 |
48 |
7 |
70 |
H2O |
93 |
73 : 27 |
13.2 |
14 |
1 |
48 |
3 |
22 (RT) |
H2O |
18 |
73 : 27 |
6 |
15 |
1 |
48 |
3 |
50 |
H2O |
77 |
78 : 22 |
25.7 |
16 |
1 |
48 |
3 |
100 |
H2O |
91 |
76 : 24 |
30.3 |
17 |
1 |
2 |
3 |
70 |
H2O |
8 |
76 : 24 |
2.6 |
18 |
1 |
4 |
3 |
70 |
H2O |
15 |
73 : 27 |
5 |
19 |
1 |
6 |
3 |
70 |
H2O |
23 |
74 : 26 |
7.7 |
20 |
1 |
8 |
3 |
70 |
H2O |
31 |
75 : 25 |
10.3 |
21 |
1 |
12 |
3 |
70 |
H2O |
42 |
78 : 22 |
14 |
22 |
1 |
24 |
3 |
70 |
H2O |
69 |
73 : 27 |
23 |
23 |
1 |
36 |
3 |
70 |
H2O |
82 |
73 : 27 |
27.3 |
24 |
1 |
48 |
3 |
70 |
H2O |
93 |
74 : 26 |
31 |
25 |
1 |
60 |
3 |
70 |
H2O |
93 |
75 : 25 |
31 |
When 3 mol% of solid compound 1 was used as catalyst at 70 °C, an yield of 93% (syn/anti = 74
:
26) of benzaldehyde into β-nitroalkanol is reached (entry 1, Table 2) after 48 h, which does not change upon further prolongation of the reaction time to 60 h (entry 25, Table 2) [Fig. 5(A)]. With 2 as catalyst a slightly lower yield of 90% (syn/anti = 65
:
35) was obtained under the same experimental condition (entry 2, Table 2). The system exhibits diastereoselectivity towards the syn isomer, typically leading to syn
:
anti molar ratios in the range from 65
:
35 to 78
:
22 using nitroethane as substrate.
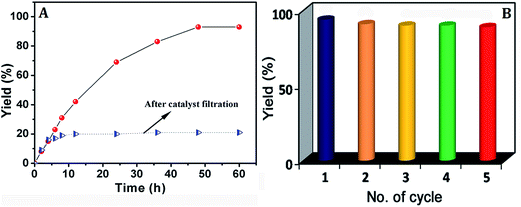 |
| Fig. 5 (A) Plot of β-nitroalkanol yield vs. time for the Henry reaction of p-nitrobenzaldehyde and nitroethane with 1. (B) Obtained yield in five consecutive reaction cycles employing 1 as catalyst. | |
A blank test with p-nitrobenzaldehyde was performed in the absence of any metal catalyst at 70 °C in aqueous medium, leading to ca. 11% conversion of p-nitrobenzaldehyde into β-nitroalkanol, after a reaction time of 48 h (Table 2, entry 7). The simple metal salts Zn(NO3)2·6H2O and Cu(NO3)2·3H2O and the ligand HL were also tested in water and the obtained reaction yields were much lower than that of catalyst 1 (Table 2, entries 8–10).
The effect of catalyst 1 concentration (1 to 7 mol%), with all other parameters unchanged, was tested and the best yield was obtained in presence for 3 mol% of catalyst (Table 2, entry 1). A decrease in the catalyst loading from 3% to 1% led to a high decrease in the yield (Table 2, entry 11), but an increase in the catalyst loading did not have any significant effect (Table 2, entries 12 and 13).
We have also examined the effect of temperature. Its decrease below 70 °C resulted in an yield lowering (entries 1, 14 and 15, Table 2). However, increase in the reaction temperature above 70 °C had no significant effect on the yield (entries 1 and 16, Table 2).
The effects of solvents other than water, such as methanol (MeOH), tetrahydrofuran (THF) and acetonitrile (CH3CN), were also analyzed (Table 2, entries 3–6). Acetonitrile led to the lowest yield (ca. 10%), followed by THF (ca. 25%) and MeOH (ca. 75%). These yields are much lower than that (93%) obtained with water which appears as the best solvent.
Finally, under the optimized reaction conditions for 1 and p-nitrobenzaldehyde, a series of substituted benzaldehydes and aliphatic ones were examined, producing the corresponding β-nitroalkanols with yields ranging from 45 to 90% (Table 3). Aromatic aldehydes with electron-withdrawing groups i.e., p-chloro and p-fluoro substituent (entries 7 and 8, Table 3) lead to yields approaching that of the p-nitro substituent with a comparable diastereoselectivity. However, electron-donor substituents, as well as aliphatic aldehydes, provide lower reactivity and diastereoselectivity (entries 2, 3 and 5, 6, Table 3). Such observations are in accord with the expected electrophilic character of the aldehyde carbon towards the Henry reaction (see below).
Table 3 Henry reaction of various aldehydes and nitroethane with catalyst 1a
Entry |
Aldehyde |
Yieldb (%) |
Selectivityc (syn/anti) |
TONd |
Reaction conditions: 3.0 mol% of catalyst 1, aldehyde (1.0 mmol), nitroethane (0.3 mL, 4.0 mmol) and water (2.0 mL). Number of moles of β-nitroalkanol per 100 moles of aldehyde. Calculated by 1H NMR. Number of moles of β-nitroalkanol per mole of catalyst. |
1 |
Benzaldehyde |
77 |
78 : 22 |
25.6 |
2 |
p-Anisaldehyde |
45 |
76 : 24 |
15 |
3 |
p-Methylbenzaldehyde |
53 |
70 : 30 |
17.6 |
4 |
m-Hydroxybenzaldehyde |
61 |
78 : 22 |
20.3 |
5 |
Acetaldehyde |
56 |
72 : 28 |
18.7 |
6 |
Propionaldehyde |
62 |
69 : 31 |
20.7 |
7 |
p-Chlorobenzaldehyde |
87 |
78 : 22 |
29 |
8 |
p-Fluorobenzaldehyde |
90 |
76 : 24 |
30 |
To investigate the catalyst stability and reusability, we have carried out a recycling study using p-nitrobenzaldehyde and nitroethane as the model substrates, adopting the aforesaid optimized conditions. The catalyst 1 was recovered and reused in five consecutive runs, without any apparent loss of the catalytic activity [Fig. 5(B)]. Furthermore, the catalysts were found to be stable, retaining their structural integrity as evidenced from FT-IR spectra (Fig. S15†) and powder XRD (Fig. S16, ESI†), where no significant changes were observed after the reaction.
In order to test the possibility of catalyst leaching and the heterogeneity of the catalytic system, an experiment was performed following a procedure described by Sheldon et al.15c In our experiment, the catalyst 1 was removed by filtration from the mixture when the conversion was ca. 15% (after 4 h reaction time) followed by transferring the supernatant fluid and stirring for an additional 44 h under the same reaction conditions. As shown in Fig. 5(A), after removal of the catalyst by filtration, the conversion of benzaldehyde is almost negligible. These results evidence that the catalysis is heterogeneous in nature and no significant catalyst leaching occurs. Additionally, the potential leaching of the catalyst into the reaction mixture was also analyzed by ICP. For this purpose, the catalyst was removed by filtration after 48 h, the filtrated solution was evaporated to dryness and the residue was dissolved in HNO3. The ICP analysis of the sample showed the presence of zinc in only ca. 0.02% of the amount used in the reaction, thus ruling out any significant leaching of the catalyst.
In contrast to the previously reported heterogeneous catalysts (Table 4),11f,24a,28 our catalysts have the advantages of being easy-to-prepare and operate preferably in aqueous medium producing high product yields.
Table 4 Comparison of activities of MOFs as heterogeneous catalysts for the Henry reaction with aldehyde and nitroethane
Catalyst |
Solvent/temp./time |
Aldehyde |
Yield (%) |
Selectivity syn : anti |
Reference |
1 |
H2O/70 °C/48 h |
p-Nitrobenzaldehyde |
93 |
74 : 26 |
This paper |
2 |
H2O/70 °C/48 h |
p-Nitrobenzaldehyde |
90 |
65 : 35 |
This paper |
Zn(II) MOF with 4,4-bypyridine and 2-acetamidoterephthalic acid |
MeOH/70 °C/48 h |
p-Nitrobenzaldehyde |
98 |
78 : 22 |
11f |
Cu(II) MOF with pyridine carboxylates |
Solvent free/70 °C/36 h |
p-Nitrobenzaldehyde |
85 |
Not determined |
24a |
Zn(II) MOF with 1,3,5-tri(4-carboxyphenoxy)benzene |
Solvent free/70 °C/72 h |
p-Nitrobenzaldehyde |
15 |
Not determined |
28a |
Zn(II) MOF with terphenyl-3,3,-dicarboxylate and 1,4-diazabicyclo[2.2.2]octane (DABCO) |
Solvent free/60 °C/120 h |
p-Nitrobenzaldehyde |
34 |
Not determined |
28b |
The nitroaldol reaction generally involves the nucleophilic addition of a nitronate ion (which is generated in situ by the deprotonation of nitroalkane ligand by an external base or by the catalyst) to the aldehyde carbonyl group to give a β-hydroxynitroalkane.11a,f,17f,18c,19a–c The metal Lewis acid character and the basic environment provided by the amide backbone in the ligand framework are favourable to the nitroaldol reaction which occurs efficiently. The water solvent, in view of its amphoteric character, can also be involved in the deprotonation/protonation steps.
Conclusions
In conclusion, we have described the syntheses and characterization of two new novel metal–organic frameworks of Zn(II) and Cu(II) (1 and 2 respectively), using a new amide appended ligand HL. The single crystal X-ray diffraction analysis revealed 1 and 2 possess 2D and 3D dimensional structures, respectively. Further, the 2D framework of 1 contains a 3-fold interpenetration which is not observed in 2. Topological analysis of 1 and 2 show a 6-connected pcu alpha-Po primitive cubic and a 8-connected body centred cubic (bcu) topology, respectively.
Both complexes 1 and 2 effectively catalyze the nitro-aldol reaction of aldehydes with nitroethane producing the corresponding β-nitroalkanols in high yields with a significant diastereoselectivity in aqueous medium. To our knowledge, 1 and 2 provide the first examples of a Zn- or Cu-MOF heterogeneous catalyst for this reaction in water. The preferential use of water instead of an organic solvent is an important feature towards green catalysis for the preparation of simple nitro alcohols. In addition, the catalyst are highly stable and can be reused a number of times, without significant loss of catalytic property. They deserve to undergo further investigation as possible heterogeneous catalysts in other important types of organic reactions in aqueous medium.
Acknowledgements
This work has been partially supported by the Fundação para a Ciência e a Tecnologia (FCT), Portugal, and its UID/QUI/00100/2013 program. The authors A. P. and A. K. are grateful to the Foundation for Science and Technology (FCT), Portugal, for the award of a postdoctoral fellowship (ref.: SFRH/BPD/88450/2012 and SFRH/BPD/76192/2011). The authors also acknowledge the Portuguese NMR Network (IST-UL Centre) for access to the NMR facility.
References
-
(a) H. Zhou and S. Kitagawa, Chem. Soc. Rev., 2014, 43, 5415 RSC;
(b) M. D. Allendorf and V. Stavila, CrystEngComm, 2015, 17, 229 RSC.
-
(a) M. Eddaoudi, J. Kim, N. Rosi, D. Vodak, J. Wachter, M. O'Keefee and O. M. Yaghi, Science, 2002, 295, 469 CrossRef CAS PubMed;
(b) J. L. C. Rowsell, E. C. Spencer, J. Eckert, J. A. K. Howard and O. M. Yaghi, Science, 2005, 309, 1350 CrossRef CAS PubMed;
(c) L. J. Murray, M. Dinca and J. R. Long, Chem. Soc. Rev., 2009, 38, 1294 RSC.
-
(a) H. Furukawa and O. M. Yaghi, J. Am. Chem. Soc., 2009, 131, 8875 CrossRef CAS PubMed;
(b) C. E. Wilmer, O. K. Farha, T. Yildirim, I. Eryazici, V. Krungleviciute, A. A. Sarjeant, R. Q. Snurr and J. T. Hupp, Energy Environ. Sci., 2013, 6, 1158 RSC;
(c) Y. B. He, W. Zhou, G. D. Qian and B. L. Chen, Chem. Soc. Rev., 2014, 43, 5657 RSC;
(d) J. A. Mason, M. Veenstra and J. R. Long, Chem. Sci., 2014, 5, 32 RSC;
(e) X. Lin, I. Telepeni, A. J. Blake, A. Dailly, C. M. Brown, J. M. Simmons, M. Zoppi, G. S. Walker, K. M. Thomas, T. J. Mays, P. Hubberstey, N. R. Champness and M. Schröder, J. Am. Chem. Soc., 2009, 131, 2159 CrossRef CAS PubMed.
-
(a) J. R. Li, R. J. Kuppler and H. C. Zhou, Chem. Soc. Rev., 2009, 38, 1477 RSC;
(b) A. Corma, H. Garcia and F. X. Llabre i Xamena, Chem. Rev., 2010, 110, 4606 CrossRef CAS PubMed;
(c) J. Rong Li, J. Sculley and H. C. Zhou, Chem. Rev., 2012, 112, 869 CrossRef PubMed.
-
(a) M. D. Allendorf, C. A. Bauer, R. K. Bhakta and R. J. T. Houk, Chem. Soc. Rev., 2009, 38, 1330 RSC;
(b) J. Rocha, L. D. Carlos, F. A. Almeida Paz and D. Ananias, Chem. Soc. Rev., 2011, 40, 926 RSC;
(c) Y. Cui, Y. Yue, G. Qian and B. Chen, Chem. Rev., 2012, 112, 1126 CrossRef CAS PubMed.
- M. Kurmoo, Chem. Soc. Rev., 2009, 38, 1353 RSC.
-
(a) R. Vaidhyanathan, D. Bradshaw, J. N. Rebilly, J. P. Barrio, J. A. Gould, N. G. Berry and M. J. Rosseinsky, Angew. Chem., Int. Ed., 2006, 45, 6495 CrossRef CAS PubMed;
(b) P. Horcajada, T. Chalati, C. Serre, B. Gillet, C. Sebrie, T. Baati, J. F. Eubank, D. Heurtaux, P. Clayette, C. Kreuz, J. S. Chang, Y. K. Hwang, V. Marsaud, P. N. Bories, L. Cynober, S. Gil, G. Ferey, P. Couvreur and R. Gref, Nat. Mater., 2010, 9, 172 CrossRef CAS PubMed;
(c) P. Horcajada, R. Gref, T. Baati, P. K. Allan, G. Maurin, P. Couvreur, G. Ferey, R. E. Morris and C. Serre, Chem. Rev., 2012, 112, 1232 CrossRef CAS PubMed.
- A. C. McKinlay, R. E. Morris, P. Horcajada, G. Férey, R. Gref, P. Couvreur and C. Serre, Angew. Chem., Int. Ed., 2010, 49, 6260 CrossRef CAS PubMed.
-
(a) S. Achmann, G. Hagen, J. Kita, I. M. Malkowsky, C. Kiener and R. Moos, Sensors, 2009, 9, 1574 CrossRef CAS PubMed;
(b) J. D. Rocca and W. Lin, Eur. J. Inorg. Chem., 2010, 3725 CrossRef PubMed;
(c) L. E. Kreno, K. Leong, O. K. Farha, M. Allendorf, R. P. van Duyne and J. T. Hupp, Chem. Rev., 2012, 112, 1105 CrossRef CAS PubMed.
-
(a) Y. Y. Karabach, A. M. Kirillov, M. F. C. Guedes da Silva, M. N. Kopylovich and A. J. L. Pombeiro, Cryst. Growth Des., 2006, 6, 2200 CrossRef CAS;
(b) R. Q. Zou, H. Sakurai, S. Han, R. Q. Zhong and Q. Xu, J. Am. Chem. Soc., 2007, 129, 8402 CrossRef CAS PubMed;
(c) R. Banerjee, A. Phan, B. Wang, C. Knobler, H. Furukawa, M. O'Keeffe and O. M. Yaghi, Science, 2008, 319, 939 CrossRef CAS PubMed;
(d) M. V. Kirillova, A. M. Kirillov, M. F. C. Guedes da Silva and A. J. L. Pombeiro, Eur. J. Inorg. Chem., 2008, 3423 CrossRef CAS PubMed;
(e) Y. Y. Karabach, A. M. Kirillov, M. Haukka, M. N. Kopylovich and A. J. L. Pombeiro, J. Inorg. Biochem., 2008, 102, 1190 CrossRef CAS PubMed;
(f) S. Contaldi, C. Di Nicola, F. Garau, Y. Y. Karabach, L. M. D. R. S. Martins, M. Monari, L. Pandolfo, C. Pettinari and A. J. L. Pombeiro, Dalton Trans., 2009, 4928 RSC;
(g) A. M. Kirillov, M. V. Kirillova and A. J. L. Pombeiro, Coord. Chem. Rev., 2012, 256, 2741 CrossRef CAS PubMed;
(h) T. C. O. Mac Leod, M. N. Kopylovich, M. F. C. Guedes da Silva, K. T. Mahmudov and A. J. L. Pombeiro, Appl. Catal., A, 2012, 439, 15 CrossRef PubMed.
-
(a) M. N. Kopylovich, T. C. O. Mac Leod, K. T. Mahmudov, M. F. C. Guedes da Silva and A. J. L. Pombeiro, Dalton Trans., 2011, 40, 5352 RSC;
(b) C. Pettinari, F. Marchetti, A. Cerquetella, R. Pettinari, M. Monari, T. C. O. Mac Leod, L. M. D. R. S. Martins and A. J. L. Pombeiro, Organometallics, 2011, 30, 1616 CrossRef CAS;
(c) H. Naıli, F. Hajlaoui, T. Mhiri, T. C. O. Mac Leod, M. N. Kopylovich, K. T. Mahmudov and A. J. L. Pombeiro, Dalton Trans., 2013, 42, 399 RSC;
(d) A. Karmakar, S. Hazra, M. F. C. Guedes da Silva and A. J. L. Pombeiro, New J. Chem., 2014, 38, 4837 RSC;
(e) M. Sutradhar, M. F. C. Guedes da Silva and A. J. L. Pombeiro, Catal. Commun., 2014, 57, 103 CrossRef CAS PubMed;
(f) A. Karmakar, M. F. C. Guedes da Silva and A. J. L. Pombeiro, Dalton Trans., 2014, 43, 7795 RSC;
(g) A. Karmakar, M. F. C. Guedes da Silva, S. Hazra and A. J. L. Pombeiro, New J. Chem., 2015, 39, 3004 RSC.
-
(a) K. Schlichte, T. Kratzke and S. Kaskel, Microporous Mesoporous Mater., 2004, 73, 81 CrossRef CAS PubMed;
(b) F. Vermoortele, M. Vandichel, B. van de Voorde, R. Ameloot, M. Waroquier, V. van Speybroeck and D. E. de Vos, Angew. Chem., Int. Ed., 2012, 51, 4887 CrossRef CAS PubMed;
(c) S. Horike, M. Dinca, K. Tamaki and J. R. Long, J. Am. Chem. Soc., 2008, 130, 5854 CrossRef CAS PubMed;
(d) A. Henschel, K. Gedrich, R. Kraehnert and S. Kaskel, Chem. Commun., 2008, 4192 RSC;
(e) P. Horcajada, S. Surble, C. Serre, D. Y. Hong, Y. K. Seo, J. S. Chang, J. M. Greneche, I. Margiolaki and G. Ferey, Chem. Commun., 2007, 2820 RSC;
(f) L. Alaerts, E. Seguin, H. Poelman, F. Thibault-Starzyk, P. A. Jacobs and D. E. de Vos, Chem.–Eur. J., 2006, 12, 7353 CrossRef CAS PubMed;
(g) F. G. Cirujano, F. X. Llabrés i Xamena and A. Corma, Dalton Trans., 2012, 41, 4249 RSC.
-
(a) J. S. Seo, D. Whang, H. Lee, S. I. Jun, J. Oh, Y. J. Jeon and K. Kim, Nature, 2000, 404, 982 CrossRef CAS PubMed;
(b) S. Hasegawa, S. Horike, R. Matsuda, S. Furukawa, K. Mochizuki, Y. Kinoshita and S. Kitagawa, J. Am. Chem. Soc., 2007, 129, 2607 CrossRef CAS PubMed.
-
(a) Y. K. Hwang, D. Y. Hong, J. S. Chang, S. H. Jhung, Y. K. Seo, J. Kim, A. Vimont, M. Daturi, C. Serre and G. Férey, Angew. Chem., Int. Ed., 2008, 47, 4144 CrossRef CAS PubMed;
(b) M. Savonnet, S. Aguado, U. Ravon, D. Bazer-Bachi, V. Lecocq, N. Bats, C. Pinel and D. Farrusseng, Green Chem., 2009, 11, 1729 RSC;
(c) Q. R. Fang, D. Q. Yuan, J. Sculley, J. R. Li, Z. B. Han and H. C. Zhou, Inorg. Chem., 2010, 49, 11637 CrossRef CAS PubMed;
(d) P. Serra-Crespo, E. V. Ramos-Fernandez, J. Gascon and F. Kapteijn, Chem. Mater., 2011, 23, 2565 CrossRef CAS;
(e) S. Aguado, J. Canivet and D. Farrusseng, J. Mater. Chem., 2011, 21, 7582 RSC.
-
(a) C. Perego, A. Carati, P. Ingallina, M. A. Mantegazza and G. Bellussi, Appl. Catal., A, 2001, 221, 63 CrossRef CAS;
(b) A. Corma, L. T. Nemeth, M. Renz and S. Valencia, Nature, 2001, 412, 423 CrossRef CAS PubMed;
(c) R. A. Sheldon, M. Wallau, I. W. C. E. Arends and U. Schuchardt, Acc. Chem. Res., 1998, 31, 485 CrossRef CAS;
(d) A. Corma, M. E. Domine and S. Valencia, J. Catal., 2004, 215, 294 CrossRef;
(e) Y. Zhu, G. Chuah and S. Jaenicke, J. Catal., 2004, 227, 1 CrossRef CAS PubMed;
(f) M. F. C. Guedes da Silva and A. J. L. Pombeiro, Redox Potential-Structure and Parameterization Relationships in the Characterization and Identification of Organometallic Compounds, in Advances in Organometallic Chemistry and Catalysis (The Silver/Gold Jubilee ICOMC Celebratory Book), ed. A. J. L. Pombeiro, J. Wiley & Sons, 2014, ch. 50, p. 677 Search PubMed.
-
(a) B. Chen, L. Wang, Y. Xiao, F. R. Fronczek, M. Xue, Y. Cui and G. Qian, Angew. Chem., Int. Ed., 2009, 48, 500 CrossRef CAS PubMed;
(b) B. Zheng, J. Bai, J. Duan, L. Wojtas and M. J. Zaworotko, J. Am. Chem. Soc., 2011, 133, 748 CrossRef CAS PubMed;
(c) J. Duan, Z. Yang, J. Bai, B. Zheng, Y. Li and S. Li, Chem. Commun., 2012, 48, 3058 RSC;
(d) B. Zheng, Z. Yang, J. Bai, Y. Li and S. Li, Chem. Commun., 2012, 48, 7025 RSC.
-
(a) C. M. Lee and W. D. Kumler, J. Am. Chem. Soc., 1962, 84, 571 CrossRef CAS;
(b) H. A. Bent, Chem. Rev., 1968, 68, 587 CrossRef CAS;
(c) P. L. Huyskens, J. Am. Chem. Soc., 1977, 99, 2578 CrossRef CAS;
(d) M. Shibasaki and H. Groger, in Comprehensive Asymmetric Catalysis, ed. E. N. Jacobsen, A. Pfaltz and H. Yamamoto, Springer, Berlin, Germany, 1999, p. 1075 Search PubMed;
(e) M. Shibasaki, H. Groger and M. Kanai, in Comprehensive Asymmetric Catalysis, Supplement 1, ed. E. N. Jacobsen, A. Pfaltz and H. Yamamoto, Springer, Heidelberg, Germany, 2004, p. 131 Search PubMed;
(f) J. Boruwa, N. Gogoi, P. P. Saikia and N. C. Barua, Tetrahedron: Asymmetry, 2006, 17, 3315 CrossRef CAS PubMed;
(g) C. Palomo, M. Oiarbide and A. Laso, Eur. J. Org. Chem., 2007, 2561 CrossRef CAS PubMed.
-
(a) M. Shibasaki, M. Kanai, S. Matsunaga and N. Kumagai, in Bifunctional Molecular Catalysis, ed. T. Ikariya and M. Shibasaki, 2011, ch. 37, pp. 1–30 Search PubMed;
(b) C. Palomo, M. Oiarbide and A. Mielgo, Angew. Chem., Int. Ed., 2004, 43, 5442 CrossRef CAS PubMed;
(c) S. Jammi and T. Punniyamurthy, Eur. J. Inorg. Chem., 2009, 2508 CrossRef CAS PubMed;
(d) S. Jammi, M. A. Ali, S. Sakthivel, L. Rout and T. Punniyamurthy, Chem.–Asian J., 2009, 4, 314 CrossRef CAS PubMed;
(e) P. Chintala, S. K. Ghosh, E. Long, A. D. Headley and B. Nia, Adv. Synth. Catal., 2011, 353, 2905 CrossRef CAS PubMed;
(f) G. Lai, F. Guo, Y. Zheng, Y. Fang, H. Song, K. Xu, S. Wang, Z. Zha and Z. Wang, Chem.–Eur. J., 2011, 17, 1114 CrossRef CAS PubMed.
-
(a) A. Das, R. I. Kureshya, K. J. Prathap, M. K. Choudhary, G. V. S. Rao, N. H. Khana, S. H. R. Abdi and H. C. Bajaj, Appl. Catal., A, 2013, 459, 97 CrossRef CAS PubMed;
(b) N. Qi, R.-Z. Liao, J.-G. Yu and R.-Z. Li, J. Comput. Chem., 2009, 31, 1376 Search PubMed;
(c) A. Bulut, A. Aslan and O. Dogan, J. Org. Chem., 2008, 73, 7373 CrossRef CAS PubMed;
(d) R. Kowalczyk, L. Sidorowicz and J. Skarzewski, Tetrahedron: Asymmetry, 2007, 18, 2581 CrossRef CAS PubMed.
-
(a) V. J. Bulbule, V. H. Deshpande, S. Velu, A. Sudalai, S. Sivasankar and V. T. Sathe, Tetrahedron, 1999, 55, 9325 CrossRef CAS;
(b) R. Maggi, D. Lanari, C. Oro, G. Sartori and L. Vaccaro, Eur. J. Org. Chem., 2011, 5551 CrossRef CAS PubMed;
(c) T. Nitabaru, A. Nojiri, M. Kobayashi, N. Kumagai and M. Shibasaki, J. Am. Chem. Soc., 2009, 131, 13860 CrossRef CAS PubMed.
-
(a) F. A. Luzzio, Tetrahedron, 2001, 57, 915 CrossRef CAS;
(b) A. V. Davis, M. Driffield and D. K. Smith, Org. Lett., 2001, 3, 3075 CrossRef CAS PubMed;
(c) J. M. Concellon, H. R. Solla and C. Concellon, J. Org. Chem., 2006, 71, 7919 CrossRef CAS PubMed;
(d) T. Jiang, H. X. Gao, B. X. Han, G. Y. Zhao, Y. H. Chang, W. Z. Wu, L. Gao and G. Y. Yang, Tetrahedron Lett., 2004, 45, 2699 CrossRef CAS PubMed;
(e) J. A. Weeden and J. D. Chisholm, Tetrahedron Lett., 2006, 47, 9313 CrossRef CAS PubMed.
-
(a) A. C. Forsyth, R. M. Paton and I. Watt, Tetrahedron Lett., 1989, 30, 993 CrossRef CAS;
(b) F. A. Luzzio and R. W. Fitch, Tetrahedron Lett., 1994, 35, 6013 CrossRef;
(c) A. Cwik, A. Fuchs, Z. Hella and J. Clacens, Tetrahedron, 2005, 61, 4015 CrossRef CAS PubMed;
(d) D. Simoni, F. P. Invidiata, S. Manfredini, R. Ferroni, I. Ampronti and G. P. Pollini, Tetrahedron Lett., 1997, 38, 2749 CrossRef CAS;
(e) X. Wang and S. Cheng, Catal. Commun., 2006, 7, 689 CrossRef CAS PubMed;
(f) V. J. Mayani, S. H. R. Abdi, R. I. Kureshy, N. H. Khan, A. Das and H. C. Bajaj, J. Org. Chem., 2010, 75, 6191 CrossRef CAS PubMed;
(g) G. Madhavi, S. J. Kulkarni, K. R. Murthy, V. Viswanathan and K. V. Raghavan, J. Porous Mater., 2007, 14, 433 CrossRef CAS;
(h) C. Murugan, H. C. Bajaj and R. V. Jasra, Catal. Lett., 2010, 137, 224 CrossRef CAS;
(i) M. A. Khalilzadeh, A. Hosseini and A. Pilevar, Eur. J. Org. Chem., 2011, 1587 CrossRef CAS PubMed;
(j) B. Basudeb, P. Das and S. Das, Curr. Org. Chem., 2008, 12, 14118 Search PubMed;
(k) W. Xie and X. Huang, Catal. Lett., 2006, 107, 53 CrossRef CAS;
(l) L. Kurti and B. Czako, Strategic Applications of Named Reactions in Organic synthesis, Elsevier Academic Press, M A, Burlington, 2005 Search PubMed.
-
(a) M. N. Kopylovich, A. Mizar, M. F. C. Guedes da Silva, T. C. O. Mac Leod, K. T. Mahmudov and A. J. L. Pombeiro, Chem.–Eur. J., 2013, 19, 588 CrossRef CAS PubMed;
(b) K. T. Mahmudov, M. N. Kopylovich, M. Haukka, G. S. Mahmudova, E. F. Esmaeila, F. M. Chyragov and A. J. L. Pombeiro, J. Mol. Struct., 2013, 1048, 108 CrossRef CAS PubMed;
(c) S. Hazra, A. Karmakar, M. F. C. Guedes da Silva, L. Dlháň, R. Boča and A. J. L. Pombeiro, New J. Chem., 2015, 39, 3424 RSC;
(d) B. G. M. Rocha, T. C. O. Mac Leod, M. F. C. Guedes da Silva, K. V. Luzyanin, L. M. D. R. S. Martins and A. J. L. Pombeiro, Dalton Trans., 2014, 43, 15192 RSC.
-
(a) L. X. Shi and C. D. Wu, Chem. Commun., 2011, 47, 2928 RSC;
(b) D. D. Qin, W. H. Lai, D. Hu, Z. Chen, A. A. Wu, Y. P. Ruan, Z. H. Zhou and H. B. Chen, Chem.–Eur.
J., 2012, 18, 10515 CAS;
(c) B. Ni and J. He, Tetrahedron Lett., 2013, 54, 462 CrossRef CAS PubMed;
(d) E. Wolinska, Tetrahedron, 2013, 69, 7269 CrossRef CAS PubMed;
(e) Z. Kałuza, K. Bielawski, R. Ć. Wiek, P. Niedziejko and P. Kaliski, Tetrahedron: Asymmetry, 2013, 24, 1435 CrossRef PubMed.
- R. Ballini, G. Bosica and P. Forconi, Tetrahedron, 1996, 52, 1677 CrossRef CAS.
- N. Neelakandeswari, G. Sangami, P. Emayavaramban, R. Karvembu, N. Dharmaraj and H. Y. Kim, Tetrahedron Lett., 2012, 53, 2980 CrossRef CAS PubMed.
- K. K. Sharma, A. V. Biradar, S. Das and T. Asefa, Eur. J. Inorg. Chem., 2011, 3174 CrossRef CAS PubMed.
-
(a) X. M. Lin, T. T. Li, Y. W. Wang, L. Zhang and C. Y. Su, Chem.–Asian J., 2012, 7, 2796 CrossRef CAS PubMed;
(b) J. M. Gu, W. S. Kim and S. Huh, Dalton Trans., 2011, 40, 10826 RSC;
(c) P. W. Siu, Z. J. Brown, O. K. Farha, J. T. Hupp and K. A. Scheidt, Chem. Commun., 2013, 49, 10920 RSC.
-
(a) J. A. L. da Silva, J. J. R. Fraústo da Silva and A. J. L. Pombeiro, Coord. Chem. Rev., 2011, 255, 2232 CrossRef CAS PubMed;
(b) L. M. D. R. S. Martins and A. J. L. Pombeiro, Coord. Chem. Rev., 2014, 265, 74 CrossRef CAS PubMed;
(c) A. J. L. Pombeiro, in Advances in Organometallic Chemistry and Catalysis, ed. A. J. L. Pombeiro, John Wiley & Sons, Inc., Hoboken, NJ, USA, 2013, ch. 2, p. 15 Search PubMed.
-
(a) L. Gonsalvi and M. Peruzzini, Aqueous Phase Reactions Catalysed by Transition Metal Complexes of 7-Phospha-1,3,5-Triazaadamantane (PTA) and derivatives, in Phosphorus compounds: advanced tools in catalysis and material sciences, ed. M. Peruzzini and L. Gonsalvi, Springer, London, Catalysis by Metal Complexes series, 1st edn, 2011, ch. 7, vol. 37 Search PubMed;
(b) F. Joó, Aqueous Organometallic Catalysis, Kluwer, Dordrecht, 2001 Search PubMed;
(c) Catalytic rearrangements and allylation reactions in water, in Metal-Catalyzed Reactions in Water, ed. V. Cadierno, J. García-Álvarez, S. E. García-Garrido and P. H. Dixneuf, Wiley-VCH, 2013, p. 243 Search PubMed;
(d) T. Kitanosonoa and S. Kobayashi, Adv. Synth. Catal., 2013, 355, 3095 CrossRef PubMed;
(e) H. Gong, H. Zeng, F. Zhou and C. J. Li, Angew. Chem., Int. Ed., 2015, 54, 5718 CrossRef CAS PubMed;
(f) S. N. Arbuzova, N. K. Gusarova, S. I. Verkhoturova, T. I. Kazantseva, I. A. Ushakov, A. G. Mal'kina and B. A. Trofimov, Heterocycl. Chem., 2015, 26, 3 Search PubMed;
(g) S. Keesara, M. R. Mandapati and S. Parvathaneni, Appl. Catal., A, 2015, 496, 58 CrossRef CAS PubMed;
(h) A. K. Rathi, M. B. Gawande, R. Zboril and R. S. Varma, Coord. Chem. Rev., 2015, 291, 68 CrossRef CAS PubMed.
- D. D. Perrin, W. L. F. Armango and D. R. Perrin, Purification of Laboratory Chemicals, Pergamon, Oxford, U.K, 1986 Search PubMed.
- K. Splith, I. Neundorf, W. Hu, H. W. P. N'Dongo, V. Vasylyeva, K. Merzb and U. Schatzschneider, Dalton Trans., 2010, 39, 2536 RSC.
-
(a) Bruker, APEX2 & SAINT, Bruker AXS Inc., Madison, Wisconsin, USA, 2007 Search PubMed;
(b) G. M. Sheldrick, Acta Crystallogr., Sect. A: Found. Crystallogr., 2008, 64, 112 CrossRef CAS PubMed;
(c) A. Altomare, M. C. Burla, M. Camalli, G. L. Cascarano, C. Giacovazzo, A. Guagliardi, A. G. G. Moliterni, G. Polidori and R. Spagna, J. Appl. Crystallogr., 1999, 32, 115 CrossRef CAS;
(d) L. J. Farrugia, J. Appl. Crystallogr., 2012, 45, 849 CrossRef CAS;
(e) C. F. Macrae, I. J. Bruno, J. A. Chisholm, P. R. Edgington, P. McCabe, E. Pidcock, L. Rodriguez-Monge, R. Taylor, J. van de Streek and P. A. Wood, J. Appl. Crystallogr., 2008, 41, 466 CrossRef CAS;
(f) A. L. Spek, Acta Crystallogr., Sect. D: Biol. Crystallogr., 2009, 65, 148 CrossRef CAS PubMed.
- H. L. Hsiao, C. J. Wu, W. Hsu, C. W. Yeh, M. Y. Xie, W. J. Huang and J. D. Chen, CrystEngComm, 2012, 14, 8143 RSC.
- A. W. Addison, T. N. Rao, J. Reedijk, J. van Rijn and G. C. Verschoor, J. Chem. Soc., Dalton Trans., 1984, 1349 RSC.
-
(a) M. O'Keeffe and O. M. Yaghi, Reticular Chemistry Structure Resource, Arizona State University, Tempe, AZ, 2005, http://www.rcsr.anu.edu.au/ Search PubMed;
(b) V. A. Blatov, IUCr Computing Commission Newsletter, 2006, 7, 4 Search PubMed;
(c) V. A. Blatov, Struct. Chem., 2012, 23, 955 CrossRef CAS.
Footnote |
† Electronic supplementary information (ESI) available: Experimental procedure, thermogravimetric analyses, Fig. S1–S16 containing FT-IR, 1H and 13C-NMR, powder XRD and Tables S1 and S2. CCDC 1414611 and 1414612. For ESI and crystallographic data in CIF or other electronic format see DOI: 10.1039/c5ra14637b |
|
This journal is © The Royal Society of Chemistry 2015 |