DOI:
10.1039/C5RA14515E
(Paper)
RSC Adv., 2015,
5, 78406-78413
Resistivity minima in the disordered cluster glass intermetallic compound Dy5Pd2: influence of quantum interference effects
Received
22nd July 2015
, Accepted 2nd September 2015
First published on 3rd September 2015
Abstract
The intermetallic compound Dy5Pd2 has been investigated by means of structural, magnetic, heat capacity, and electrical resistivity measurements. The results indicate the formation of a cluster glass state below 42 K, which originates from atomic disorder and spin frustration in the magnetic atom sublattice of the cubic unit cell. The behavior of the heat capacity and electrical resistivity of Dy5Pd2 has also been studied theoretically. The magnetic part of the specific heat exhibits a T3/2 temperature dependence below the cluster glass freezing temperature. A minimum in the temperature dependence of the resistivity has been observed around 60 K. This behavior has been interpreted in terms of the combined effects of electron–electron interactions and weak localization as well as contributions from magnetic and phonon scattering.
1 Introduction
The resistivity minimum in metallic alloys has always been a topic of considerable interest. Resistivity minima were first observed in crystalline alloys with dilute magnetic elements at very low temperatures due to the Kondo effect.1,2 Later on, the Kondo effect was also observed in a concentrated system.3 After that, resistivity minima similar to the Kondo systems have been observed in metallic glasses,4,5 bulk ceramics,6 epitaxially grown films,7,8 single crystals,9 composite materials10,11 etc. Extensive studies were performed both experimentally and theoretically to investigate the origin of the occurrence of these resistivity minima. Different mechanisms, responsible for the observations of resistivity minima, like spin-polarized tunneling via grain boundaries,6,11 Kondo-like effects,12 quantum interference effects arising from electron–electron (e–e) interactions and weak localization (WL),7,10 were reported. Among them, the observation and studies of resistivity minima due to the quantum interference effects arising from electron–electron interactions and weak localization are just a few. There are also a few reports on the resistivity minima in crystalline intermetallic compounds13–16 with concentrated magnetic elements.
Earlier studies on intermetallic R5Pd2 (R = rare earth) compounds found complex electrical transport and magnetic behavior which was reported to occur due to a frustration effect that originated from antiferromagnetic ordering with a triangular lattice configuration of the rare earth ions.17 Later on, the absence of long range magnetic ordering and cluster glass (CG) magnetic behavior18 were confirmed from neutron scattering and ac and dc magnetization measurements in R5Pd2 (R = Ho, Tb) compounds. However the interesting electrical transport properties in R5Pd2 compounds have remained unrevealed.
The compounds of this series are also important from an application point of view because they show a large magnetocaloric cooling power.19,20 Recently, we studied the magnetic and magnetocaloric properties of Dy5Pd2.21 A giant inverse magnetocaloric effect was observed in this compound due to its magnetic irreversibility. Here we have investigated the magnetic relaxation, rejuvenation and memory effects in Dy5Pd2. Glassy behavior such as fascinating memory effects and ageing properties are not only experimental techniques to distinguish the glassy behavior of the compounds, but are also believed to be of practical use.22 In the present paper, we have also studied temperature dependent X-ray diffraction (XRD), heat capacity and electrical resistivity both experimentally and theoretically. Our main motivation is to reveal the complex electrical transport behavior in this compound. We have analyzed the transport properties in Dy5Pd2 in detail. The observed resistivity minimum in the temperature dependence has been interpreted in terms of the e–e interaction effects in the presence of WL and magnetic contributions due to the CG behavior of this compound.
2 Sample preparation and characterization
The polycrystalline sample was prepared by arc melting of its constituent elements of purity greater than 99.9% in an argon gas atmosphere. The ingot was remelted several times to ensure uniformity of the prepared sample. Dy of 99.9% purity was bought from Alfa Aesar (a Johnson Matthey company) and Pd of purity higher than 99.99% was bought from Arora-Matthey Limited. The room temperature and low temperature XRD study was carried out by using a Cu Kα source of 1.54 Å wavelength in a rotating anode X-ray diffractometer (Rigaku TTRAX-III). The dc magnetization measurements were performed using a superconducting quantum interference device (SQUID VSM, Quantum Design) magnetometer. AC susceptibility measurements were performed at frequencies in the range 37–1737 Hz using a commercial susceptometer (CryoBIND model). Specific heat studies were carried out using the physical property measurement system (PPMS, Quantum Design). The temperature dependence of resistivity (ρ(T)) was measured by the conventional four probe method.
3 Results and discussion
3.1 X-ray diffraction studies
The cubic crystalline structure (space group Fd3m) and single phase nature of the compound has been confirmed by XRD measurements. The lattice parameters and atomic coordinates of the bulk crystalline sample at different temperatures have been determined by a standard Rietveld refinement using the FULLPROF 2009 program. During refinement the occupancy factors were fixed to the previously reported values.23 Using the Rietveld profile fit, the evaluated value of the lattice parameter from the room temperature XRD pattern is found to be 13.517(1) Å, which agrees with the previously reported value.23 The atomic coordinates along with the occupancy factors are presented in Table 1. The crystal structure of Dy5Pd2 along with the different Wyckoff sites occupied by the Dy and Pd atoms, as mentioned in Table 1, are shown in Fig. 1.
Table 1 Atomic coordinates and occupancy factors for Dy5Pd2
Atom |
x |
y |
z |
Occupancy |
Dy (1) |
0.8114 |
0.125 |
0.125 |
1 |
Dy (2) |
0.0232 |
x |
x |
1/2 |
Dy (3) |
0.1815 |
x |
x |
1/8 |
Pd |
0.2237 |
x |
x |
7/8 |
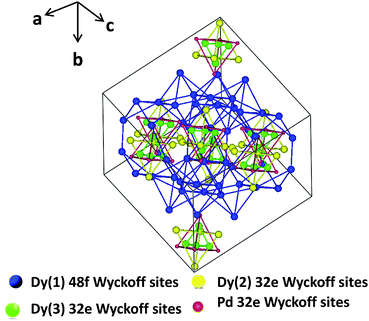 |
| Fig. 1 Crystal structure of Dy5Pd2 showing the different Dy and Pd sites. | |
In accordance with the earlier work,23 the dysprosium and palladium atoms occupy three nonequivalent positions:
(i) The 48f Wyckoff site occupied by dysprosium atoms with a factor of 100%.
(ii) The 32e Wyckoff site occupied by dysprosium atoms with a factor not greater than 50%.
(iii) 4 dysprosium atoms and 28 palladium atoms sharing almost the same 32e Wyckoff site.
This complex structure provides substantial atomic disorder in Dy5Pd2. The temperature dependence of the unit cell volume is shown in Fig. 2. We have analyzed the data using a Debye–Grüneisen formula24 described by:
|
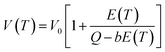 | (1) |
where
V0 is the unit cell volume at zero temperature, and
Q and
b are related to the zero temperature isothermal bulk modulus and its first derivative with respect to pressure.
E(
T) reflects the internal energy due to lattice vibrations and is given by the Debye model:
|
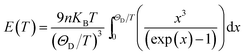 | (2) |
where
ΘD is the Debye temperature,
n is the number of atoms per unit cell, and
KB is the Boltzmann constant. The dashed line in
Fig. 2 is the fitted curve using
eqn (1) with the following four parameters:
ΘD = 148 K,
V0 = 2445 Å
3,
Q = 9.43 × 10
−17 J, and
b = 0.52.
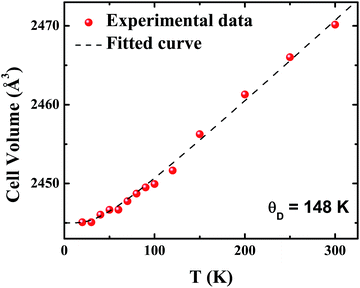 |
| Fig. 2 The temperature dependence of the refined unit cell volume is shown together with the theoretical curve obtained from the Debye–Grüneisen formula. | |
Thus, the XRD results follow typical Debye–Grüneisen behavior with no anomaly or structural change in the entire temperature region.
3.2 DC magnetization
The temperature dependence of the zero-field-cooled (ZFC) and field-cooled (FC) dc magnetization (M) data for an applied magnetic field of 100 Oe is shown in Fig. 3(a). The temperature dependence of magnetization measured in the ZFC protocol exhibits a cusp at about Tf = 38 K. The ZFC and FC magnetic susceptibility exhibit strong irreversibility. The irreversibility in the ZFC and FC magnetization along with the increase of FC magnetization upon cooling is likely to be associated with a cluster glass or long range ferromagnetic state. The difference between the FC and ZFC magnetization as a function of temperature is shown in Fig. 3(b). The point to be noted is that the irreversibility in the FC–ZFC magnetization actually starts well above Tf. To get deeper insight into the low temperature phase aging, rejuvenation and memory effect experiments have been performed and are described in the following sections.
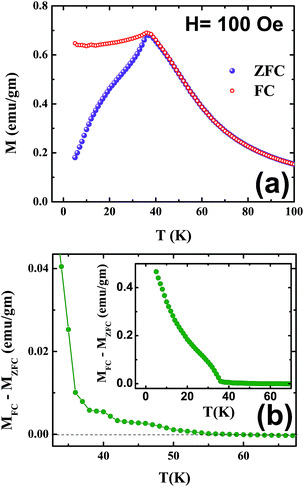 |
| Fig. 3 (a) The temperature dependence of zero-field-cooled and field-cooled magnetization measured under a 100 Oe magnetic field. The ZFC curve shows a cusp around 38 K. (b) The difference in magnetization (MFC − MZFC) as a function of temperature. | |
3.2.2 Memory effect. The memory effect in the temperature dependence of field-cooled dc magnetization in Dy5Pd2 has been probed following the protocol27 by Sun et al. At first the sample was cooled at H = 500 Oe from 200 to 2 K with intermediate halts of duration tw = 1 h each at Tstop = 50, 30, 20, 10 and 5 K. During each stop, the temperature was kept constant while the magnetic field was reduced to zero. After each stop, the magnetic field was reapplied and cooling was resumed, which resulted in a step-like magnetization curve (MFCCstop). After reaching the lowest temperature the sample was heated in the presence of a H = 500 Oe magnetic field without any stops. The memory curve (MFCHmem) was obtained which exhibits a clear upturn at each Tstop below the freezing temperature shown in Fig. 5. A reference curve is also presented in the same figure which was simply recorded in the field-cooled protocol without any stops (MFCHRef). The observed memory effect signifies the nonergodic behavior28 of the sample in the low temperature region. For all the measurements done for memory effects the temperature ramp rate was kept very slow, fixed at 1 K min−1.
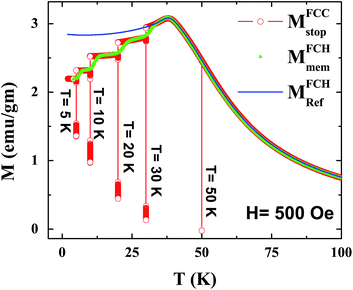 |
| Fig. 5 Memory effect in the temperature variation of the ZFC dc magnetization of Dy5Pd2. The curves were obtained by cooling the sample in the presence of a 500 Oe magnetic field with intermediate stops of 1 h at T = 50, 30, 20, 10 and 5 K (MFCCstop). The memory curve was obtained by subsequent field heating (MFCHmem). A reference curve (MFCHref) was measured during heating after the sample was cooled in presence of a 500 Oe magnetic field without any intermediate stops. | |
We have performed simultaneous temperature and magnetic field cycling in the time dependence of magnetization to check the robustness of magnetic memory in this system (Fig. 6(a)). In this experiment the sample was cooled down to the temperature T0 = 20 K from 300 K at a cooling rate of 1 K min−1 in the absence of a magnetic field. After reaching T0, a magnetic field of 500 Oe was applied and subsequently the magnetization was recorded as a function of time (t). After the period of time t1 = 1 h, the temperature was decreased to T0 − ΔT = 15 K very quickly and the magnetic field was switched off at the faster rate. Under these conditions, the magnetization was recorded for the time period t2 = 1 h. After the time period of t2, the sample was heated back to T0, the magnetic field of 500 Oe was reapplied and the magnetization was recorded for another time t3 = 1 h. The relaxation process during t3 is simply a continuation of the process during t1. This reflects that the state of the system at the end of t1 (just before cooling) is recovered when the sample is cycled back to the initial temperature and magnetic field.
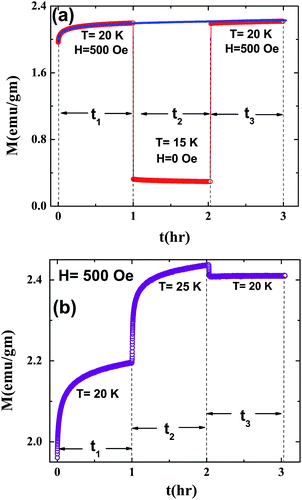 |
| Fig. 6 (a) Magnetic relaxation data measured in the zero-field-cooled state at 20 K for a 500 Oe magnetic field (t1 and t3) along with an intermediate measurement at 15 K in zero field (t2). The relaxation curve during t3 is the continuation of t1. The solid relaxation curve is measured at 20 K for a 500 Oe magnetic field at a stretch. (b) Magnetic relaxation data measured in the ZFC modes at 20 K for a 500 Oe magnetic field (t1 and t3) with intermediate heating (t2) at 25 K. In this case the positive temperature cycling rejuvenates the relaxation and destroys the memory effect. | |
3.2.3 Rejuvenation. The effect of positive temperature cycling on the magnetic relaxation has also been investigated and is shown in Fig. 6(b). In the experiment the sample was cooled down to the temperature T0 = 20 K from 300 K at a cooling rate of 1 K min−1 in the absence of a magnetic field. After reaching T0 a magnetic field of 500 Oe was applied and subsequently the magnetization was recorded as a function of time t. After the period of time t1 = 1 h, the temperature was increased to T0 + ΔT = 25 K. Under these conditions the magnetization was recorded for the time period t2 = 1 h. After the time period of t2, the sample was cooled back to T0 and the magnetization was recorded for another time t3 = 1 h. The magnetization at the beginning of t3 did not go back to the level it had reached just before the temporary heating. As a result, the temporary heating rejuvenates the magnetic relaxation and there is no memory effect in the positive temperature cycling.With respect to negative and positive temperature variations, the asymmetric response in the time dependence of magnetization actually favors the hierarchical model29 of the relaxation.
3.3 AC magnetization
AC susceptibility measurements have been performed to investigate the nature of the underlying magnetic state. The ac susceptibility measurement in the ac field Hac = 1.5 Oe with a frequency (ω) of 37–1737 Hz was performed. The frequency (f) as a function of the freezing temperature (Tf) is shown in Fig. 7. It is possible to fit the data with the empirical Vogel–Fulcher relation: |
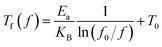 | (4) |
where Ea is the activation energy, f0 is the characteristic frequency and T0 is the VF temperature. The order of f0 for the best fitted curve is 1013 which signifies the CG behavior of the compound and the nonzero value of T0 = 41 K indicates the presence of finite interactions between the clusters.30
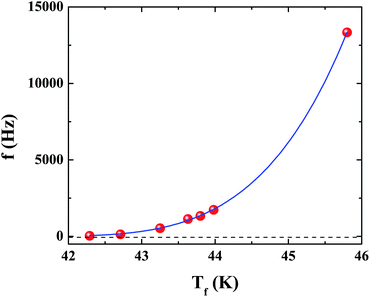 |
| Fig. 7 Frequency as a function of freezing temperature along with the curve fitted to the Vogel–Fulcher law. | |
The aging, rejuvenation and memory effects along with the ac magnetization results confirm the cluster glass behavior of the sample. Similar cluster glass behavior was also previously reported in compounds having the Fd3m pyrochlore structure.18,31
3.4 Heat capacity
The zero-field heat capacity [C0(T)] data of Dy5Pd2 measured at constant pressure are shown in Fig. 8(a). No distinct feature has been observed in our measured C0(T) data around Tf which suggests that the cusp in the magnetic susceptibility is not related to long range magnetic ordering.32 The low-temperature specific heat data do not follow the Cp(T) = γT + βT3 temperature dependence as can be seen from the Cp/T versus T2 plot (inset of Fig. 8(a)).
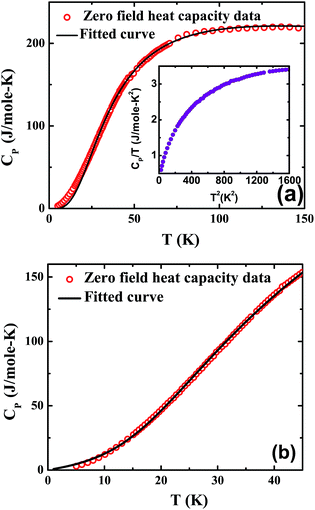 |
| Fig. 8 (a) Specific heat CP as a function of T. The solid line represents a fit to the model considering the phonon and electronic contribution to the specific heat. Inset: CP/T as a function of T2 which does not follow linear behavior at the lower temperature regime. (b) CP as a function of T below 45 K. The solid line represents a fit to the model considering the magnetic contribution due to cluster glass behavior along with the phonon and electronic contribution. | |
The Cp(T) data in the measured temperature range were fitted using the Debye model of lattice heat capacity:
|
Cp(T) = CDebye(T) + γT
| (5) |
where
CDebye represents the Debye lattice heat capacity
33 due to the acoustic phonons and is given by:
|
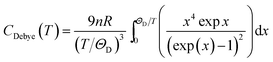 | (6) |
where
ΘD is the Debye temperature,
R is the molar gas constant and
n = 7 here. The experimental data and the Debye model fit, presented in
Fig. 8(a), significantly deviate in the low temperature region. However, the specific heat data below 45 K could be fitted well by including a magnetic term
34 σT3/2, which is typical for spin-glass systems, in
Cp(
T) =
γT +
CDebye,
i.e., by
Cp(
T) =
γT +
CDebye +
σT3/2 with the fitting parameters
γ = 731 mJ mol
−1 K
2,
ΘD = 138 K, and
σ = 49 mJ mol
−1 K
5/2. The fit is shown by the solid curve in
Fig. 8(b).
3.5 Electrical resistivity
The temperature dependence of the zero-field electrical resistivity is shown in Fig. 9(a). With the decrease of temperature, the resistivity decreases at first and then, showing a minimum around 60 K, it again increases. At a temperature above the minimum the curve has a metallic-like character. Previously resistivity minima were reported in pyrochlore compounds like Tl2−xBixMn2O7 (0 ≤ x ≤ 0.5),31 UCo2 (ref. 35) etc. In Tl2−xBixMn2O7 the minimum in the resistivity at lower temperature was interpreted to be related to freezing of the cluster glass state. The resistance minimum at low temperatures in UCox (x is close to 2.0) was reported to occur due to some Co atoms occupying the wrong sites in the cubic Laves phase structure.35 In Dy5Pd2 the overall change in the resistivity magnitude as the temperature is decreased from 150 to 2 K, which is quantified by a residual resistivity ratio [ρ(150 K)/ρ(2 K)], is small (about 1.2) though the residual resistivity itself is quite large ρ = 197 μΩ cm.
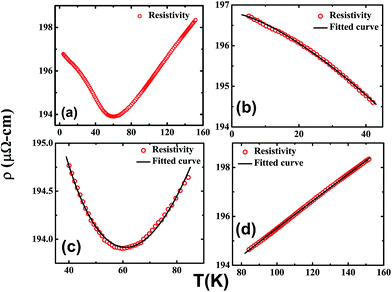 |
| Fig. 9 (a) The zero-field resistivity ρ as a function of temperature T. The temperature dependence of ρ data along with the fitted curves (solid lines) (b) in the low temperature region (T < Tf), (c) around the minimum (Tf < T < 2Tf), (d) in the high temperature region above the minimum (T > 2Tf). | |
Similar minima in the electrical resistivity were previously observed in some metals, where they were attributed to the Kondo effect, the magnetic superzone energy gap or the formation of a cluster glass state in disordered systems. The Kondo effect is not expected in the Dy5Pd2 system because in this case the 4f orbital is deep inside the Fermi level.
Another mechanism relates the increase of the electrical resistivity with decreasing temperature to the formation of magnetic superzone gaps in the ordered phase. Dy5Pd2 is a cluster glass system with no signature of long range ordering and thus the occurrence of a magnetic superzone gap seems unlikely.16
The presence of atomic disorder in Dy5Pd2 suggests the possibility of describing the low-temperature behavior of its electrical resistivity in terms of theories developed for disordered conductors. The quantum corrections to the resistivity which involve electron–electron interaction and weak localization (WL) effects have been taken into consideration. Both of these mechanisms give rise to the formation of minima in ρ(T). The e–e interactions can be better described by a
dependence14 in ρ(T). The contribution to the resistivity due to the quantum interference effect in the WL scenario16 can be described by ρWL ∝ Tn/2 for n ≥ 1.5. Besides the e–e interaction and WL effects, one also expects contributions to the measured resistivity from other competing effects like magnetic contribution due to the CG behavior of the system and from the phonon contribution.
For the phonon contribution, we have considered the standard Bloch–Grüneissen relation,
|
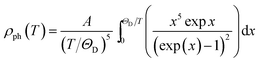 | (7) |
where
A is a constant. At low temperatures, below and around
Tf, the magnetic contribution to the resistivity arising from the scattering of conduction electrons by the spin diffusive modes in spin or cluster glasses is proportional to
T3/2.
The ρ(T) has been analyzed in three different temperature regions, viz., below Tmin, around Tmin and above Tmin. We have considered Matthiessen’s rule as the measured resistivity is the sum of all the contributions.
|
ρ(T) = ρ0 + ρe–e(T) + ρWL(T) + ρmag(T) + ρph(T)
| (8) |
where
ρ0 is the residual resistivity,
ρe–e(
T) is the contribution due to e–e interactions,
ρWL(
T) is the contribution due to weak localization,
ρph(
T) is the phonon contribution and
ρmag(
T) is the magnetic contribution.
So the expression for the resistivity can be explicitly written as:
where
B and
C1 are constants. Here we have observed −
T3/2 dependence due to the WL effect which has been confirmed by the field dependence of magnetization and magnetoresistance and will be discussed later on. As a result the third term in the right hand side of the last equation includes the contribution due to both the WL and magnetic effects.
We have fitted the resistivity data at the three different aforesaid temperature regimes to the last equation. The fitted curve is shown in Fig. 9(b)–(d), which reflects the good agreement of the observed resistivity minimum with the theoretical description. The details of the fitting parameters for the three different temperature regimes are given in Table 2.
Table 2 Fitting parameters obtained from the resistivity data analysis in three different temperature regimes
T (K) |
ρ0 (μΩ cm) |
B (μΩ cm K−1/2) |
C1 (μΩ cm K−3/2) |
A (μΩ cm) |
T < Tf |
196.8 |
−0.004 |
−0.008 |
3.5 |
Tf < T < 2Tf |
212.1 |
−3.5 |
0.02 |
20 |
T > 2Tf |
191.6 |
−0.09 |
0.000001 |
34.1 |
The most important point to be noted is the value of C1 which reflects the competition between the contribution due to WL and the magnetic CG behavior. In the low temperature region (T < Tf), the contribution due to WL is greater than the magnetic contribution and as a result the value of C1 is negative. Around the minimum (Tf < T < 2Tf) the effect due to WL is smaller with respect to the effect due to the contribution from the CG magnetic behavior and C1 takes a positive value. Around the minimum the negative contribution from the e–e interactions is also considerable and gives the observed minimum in the resistivity behavior.
To confirm the presence of WL we have analyzed the magnetic field dependence of magnetization and magnetoresistance [MR = R(H) − R(0)/R(0)] measured in the lower temperature region. For the CG alloys with no long range ordering, the isotropic MR and magnetization (M) are expected to satisfy the empirical relation36 MR(H) ∝ −M2. The magnetic field dependence of magnetization and MR measured at T = 2 K up to a 35 kOe magnetic field is shown in Fig. 10. M(H) is positive, following a M ∝ Hn dependence in this field region with n = 1.64. However the field dependence of MR shows interesting behavior. In the lower field region with increasing magnetic field, MR increases at first with a positive value. Then, showing a broad maximum, it decreases and above a certain value of the field it becomes negative. The field dependence of MR does not just follow H2n behavior and there is certainly some positive contribution from MR present which competes with the negative contribution due to the magnetic contribution, and is responsible for the positive value of the MR in the low field region.
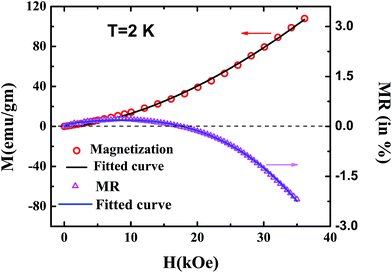 |
| Fig. 10 Magnetization (M) and magnetoresistance (MR) as a function of magnetic field measured at T = 2 K. The solid lines are fitted to the functions as discussed in the text. | |
We have fitted MR(H) with the function MR(H) = −A × Hβ + B × Hλ. The best fit yielded β = 3.1 and λ = 0.48 with the values of A and B respectively as 4.5 × 10−5 and 0.08. The positive contribution from MR(H) guarantees the presence of a quantum interference effect in Dy5Pd2 because, according to Lee et al., a
dependence37 is expected for 3D disordered systems due to weak localization.
In conclusion, the low temperature region transport phenomena in Dy5Pd2 are mainly contributed to by electron–electron interactions, weak localization and magnetic contributions.
4 Conclusions
The comprehensive studies on Dy5Pd2 by means of the results obtained from temperature dependent XRD, dc and ac magnetization, heat capacity and electrical resistivity measurements strongly suggest the absence of long range magnetic ordering and the formation of a magnetic cluster glass state at low temperature. The magnetic relaxation behavior can be described by the stretched exponential function which reflects that the system evolves through a number of intermediate states. Memory effects have clearly been observed in both temperature and time dependence by the cycling temperature and magnetic field. A T3/2 temperature dependence in specific heat below the cluster glass freezing temperature has been confirmed. A distinct minimum has been observed in the temperature dependence of resistivity in the concentrated Dy5Pd2 alloy. A T3/2 contribution to the resistivity is observed at low temperature (T < Tf) and around the minimum. The analysis of the resistivity data reflects the simultaneous presence of the T3/2 type of magnetic contribution due to the spin diffusive modes in cluster glasses and weak localization along with
dependence from the e–e interaction effects. The contribution from WL effects to resistivity has been confirmed from the field dependence of magnetization and magnetoresistance. A
dependence in MR(H) has been observed, in excess of −M2 dependence, due to WL effects. To conclude, the resistivity minimum in the concentrated intermetallic compound Dy5Pd2 has been analyzed quantitatively in terms of electron–electron interactions, weak localization effects and magnetic contributions due to the cluster glass behavior.
Acknowledgements
Tapas acknowledges the financial assistance from DAE, India. We thank Dr Tapas Samanta for helping to prepare the sample. We thank Prof. R. Ranganathan for providing experimental facilities for ac susceptibility measurements. We acknowledge Mr Sanjib Banik for helping to write programs using Mathematica.
References
- J. Kondo, Prog. Theor. Phys., 1964, 32, 37 CrossRef CAS.
- J. S. Dugdale, The Structures and Properties of Solids 5-The Electrical Properties of Metals and Alloys, Edward Arnold, London, 1977 Search PubMed.
- A. S. Edelstein, Phys. Lett. A, 1968, 27, 614 CrossRef CAS.
- R. W. Cochrane and J. O. Storm-Olsen, Phys. Rev. B: Condens. Matter Mater. Phys., 1984, 29, 1088 CrossRef CAS.
- A. Das and A. K. Majumdar, Phys. Rev. B: Condens. Matter Mater. Phys., 1991, 43, 6042 CrossRef CAS.
- E. Rozenberg, M. Auslender, I. Felner and G. Gorodetsky, J. Appl. Phys., 2000, 88, 2578 CrossRef CAS PubMed.
- M. Ziese, Phys. Rev. B: Condens. Matter Mater. Phys., 2003, 68, 132411 CrossRef.
- Y. Jin, X. P. Cui, W. H. Han, S. X. Cao, Y. Z. Gao and J. C. Zhang, Phys Chem Chem Phys, 2015, 17, 12826 RSC.
- T. Okuda, T. Kimura and Y. Tokura, Phys. Rev. B: Condens. Matter Mater. Phys., 1999, 60, 3370 CrossRef CAS.
- Y. Jin, X. L. Qian, B. Lu, S. X. Cao and J. C. Zhang, RSC Adv., 2015, 5, 2354 RSC.
- K. Das, B. Satpati and I. Das, RSC Adv., 2015, 5, 27338 RSC.
- Y. Matsushita, H. Bluhm, T. H. Geballe and I. R. Fisher, Phys. Rev. Lett., 2005, 94, 157002 CrossRef CAS.
- S. Banerjee and A. K. Roychowdhury, Solid State Commun., 1992, 83, 1047 CrossRef CAS.
- S. Chakraborty and A. K. Majumdar, Phys. Rev. B: Condens. Matter Mater. Phys., 1996, 53, 6235 CrossRef CAS.
- D. X. Li, S. Nimori, Y. Shiokawa, Y. Haga, E. Yamamoto and Y. Onuki, Phys. Rev. B: Condens. Matter Mater. Phys., 2003, 68, 172405 CrossRef.
- M. Szlawska, D. Gnida and D. Kaczorowski, Phys. Rev. B: Condens. Matter Mater. Phys., 2011, 84, 134410 CrossRef.
- M. Klimczak, E. Talik, A. Winiarski and R. Troć, J. Alloys Compd., 2006, 423, 62–65 CrossRef CAS PubMed.
- A. F. Gubkin, E. A. Sherstobitova, P. B. Terentyev, A. Hoser and N. V. Baranov, J. Phys.: Condens. Matter, 2013, 25, 236003 CrossRef CAS PubMed.
- T. Samanta, I. Das and S. Banerjee, Appl. Phys. Lett., 2007, 91, 082511 CrossRef PubMed.
- S. Toyoizumi, H. Kitazawa, Y. Kawamura, H. Mamiya, N. Terada, R. Tamura, A. Dönni, K. Morita and A. Tamaki, J. Appl. Phys., 2015, 117, 17D101 CrossRef PubMed.
- T. Paramanik, T. Samanta, R. Ranganathan and I. Das, RSC Adv., 2015, 5, 47860 RSC.
- N. Khan, P. Mandal and D. Prabhakaran, Phys. Rev. B: Condens. Matter Mater. Phys., 2014, 90, 024421 CrossRef.
- M. L. Fornasini and A. Palenzona, J. Less-Common Met., 1974, 38, 77–82 CrossRef CAS.
- H. Sim, D. C. Peets, S. Lee, S. Lee, T. Kamiyama, K. Ikeda, T. Otomo, S.-W. Cheong and J.-G. Park, Phys. Rev. B: Condens. Matter Mater. Phys., 2014, 90, 214438 CrossRef.
- D. Chu, G. G. Kenning and R. Orbach, Phys. Rev. Lett., 1994, 72, 3270 CrossRef CAS.
- R. S. Freitas, L. Ghivelder, F. Damay, F. Dias and L. F. Cohen, Phys. Rev. B: Condens. Matter Mater. Phys., 2001, 64, 144404 CrossRef.
- Y. Sun, M. B. Salamon, K. Garnier and R. S. Averback, Phys. Rev. Lett., 2003, 91, 167206 CrossRef.
- A. Bhattacharyya, S. Giri and S. Majumdar, Phys. Rev. B, 2011, 83, 134427 CrossRef.
- E. Vincent, J. Hammann, M. Ocio, J.-P. Bouchaud, and L. F. Cugliandolo, in Complex Behaviour of Glassy Systems, ed. M. Rubi and C. Perez-Vicente, Springer-Verlag Lecture Notes in Physics, vol. 492 Springer-Verlag, Berlin, 1997, p. 184 Search PubMed.
- E. Svanidze and E. Morosan, Phys. Rev. B: Condens. Matter Mater. Phys., 2013, 88, 064412 CrossRef.
- J. A. Alonso, J. L. Martiínez, M. J. Martínez-Lope and M. T. Casais, Phys. Rev. Lett., 1998, 82, 189 CrossRef.
- H. Shinaoka, Y. Tomita and Y. Motome, Phys. Rev. Lett., 2011, 107, 047204 CrossRef.
- V. K. Anand, D. T. Adroja and A. D. Hillier, Phys. Rev. B: Condens. Matter Mater. Phys., 2012, 85, 014418 CrossRef.
- J. O. Thomson and J. R. Thompson, J. Phys. F: Met. Phys., 1981, 11, 247 CrossRef CAS PubMed.
- J. Hrebik and B. R. Coles, Physica B+C, 1977, 86, 169 CrossRef.
- T. K. Nath and A. K. Majumdar, Phys. Rev. B: Condens. Matter Mater. Phys., 1998, 56, 10655 CrossRef.
- P. A. Lee and T. V. Ramakrishnan, Phys. Rev. B: Condens. Matter Mater. Phys., 1982, 26, 4009 CrossRef.
|
This journal is © The Royal Society of Chemistry 2015 |