DOI:
10.1039/C5RA13782A
(Paper)
RSC Adv., 2015,
5, 74629-74637
A fluorescence on–off sensor for Cu2+ and its resultant complex as an off–on sensor for Cr3+ in aqueous media†
Received
14th July 2015
, Accepted 27th August 2015
First published on 28th August 2015
1. Introduction
The development of selective fluorescent chemosensors for detecting biologically or environmentally important metal ions is of great importance.1–3 Serving as the third most abundant transition metal in the biological process, copper(II) has a key influence on copper-binding enzyme activities, including cytochrome c oxidase, catechol oxidase, amine oxidase and so on, both as a catalytic co-factor and as an allosteric component of cuproenzymes. Insufficient intake of Cu2+ often causes anemia, bone abnormality or neutropenia.4 However, excessive accumulation of copper(II) is toxic to the human body and leads to oxidative stress and neurodegenerative diseases.5 Consequently, developing fluorescent chemosensors of the selective recognition and quantification of Cu2+ is considerably significant in the biological and environmental process.6–14 Trivalent chromium, as an essential micronutrient for humans, plays a critical role in preventing negatively affects in the metabolism of glucose and lipids, therefore chromium(III) deficiency may cause glucose and lipid metabolism disorder,15 resulting in diabetes and cardiovascular diseases, while a high concentration level of Cr3+ may cause genotoxic effect and destroy cellular structure.16 Thus, the development of reliable, selective and sensitive fluorescent chemosensors for Cr3+ recognition has attracted immense interest in biological and environmental areas.17–22
Recently, a variety of fluorescent chemosensors for the selective recognition of Cu2+ or Cr3+ have been reported which demonstrate high selectivities for the target Cu2+ or Cr3+ over other competitive metal ions based on the enhancement or quenching of fluorescence,16–25 but most of them focus on single sensors for the selective recognition of only one target metal ion. The development of single sensors for the selective recognition of multiple metal ions, which would reduce analytic time, increase analysis speed and cut the cost, has received considerable attention. Single chemosensors for the recognition of two metal ions have been reported, such as Fe3+/Hg2+,26 Zn2+/Cu2+,23–25 Zn2+/Cd2+,27,28 Zn2+/Al3+ 29 and Hg2+/Au3+.30 In contrast, single chemosensors to detect Cu2+/Cr3+ are still rare.
In this study, we developed a coumarin–quinoline based sensor 1 (Scheme 1) for detecting of two metal ions Cu2+ and Cr3+ in aqueous media and living breast cancer MCF-7 cells. When the addition of excess Cu2+, the sensor 1 undergone fluorescence quenching and a 10 nm red-shift of the maximum absorption wavelength. The 1
:
1 binding stoichiometry was determined using the spectroscopic titration and Job's plot. The resultant 1–Cu2+ complex could detect the paramagnetic Cr3+ ion by fluorescence enhancement though 1
:
1 metal ion replacement approach. The sensor 1 showed excellent cell-membrane permeability and effectively distinguished Cu2+ and Cr3+ in living cells though fluorescence “on–off–on” signals.
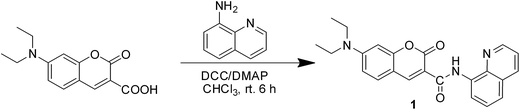 |
| Scheme 1 Synthesis of the sensor 1. | |
2. Materials and methods
2.1. Instruments and reagents
All solvents and reagents were obtained from commercial sources (Sigma-Aldrich, TCI or Aladdin) and used without further purification. MCF-7 (breast cancer) cells used for the fluorescence imaging were purchased from American Type Culture Collection. Fluorescence spectra were recorded on a FluoroMax4 spectrometer with a set of excitation and emission slits both at 3.0 nm. Absorption spectra were recorded on a UV-2550 UV-vis spectrometer. FT-IR spectra were obtained on a Nicolet 6700 Fourier-transform infrared spectrometer. The pH titration was conducted on a Leici PHS-25 pH meter. NMR spectra were obtained on an AVANCE III 500 MHz spectrometer with TMS as an internal standard. Mass spectra (MS) were recorded with an Accurate-Mass TOF LC/MS spectrometer. XPS were recorded on a Kratos AXIS Ultra DLD spectrometer. The cells were imaged by an Olympus FV1100 laser confocal fluorescence microscopy.
2.2. UV-vis and fluorescence spectrometric determination
2.2.1 Preparation of test solutions for fluorescent studies. Stock solutions (1 mM) of nitrate salts of K+, Ca2+, Na+, Mg2+, Al3+, Zn2+, Fe3+, Pb2+, Hg2+, Ag+, Co2+, Ni2+, Cd2+, Cu2+ and Cr3+ were prepared in ultrapure water. 1 mM of the stock solution of the sensor 1 was prepared in DMSO. The test solution was prepared by appropriately diluting the stock solution of the sensor 1 to 0.1 M HEPES–DMSO (9
:
1, v/v, pH = 7.2) solution and then adding the appropriate amount of each metal ion stock solution. The fluorescence quantum yield was calculated using quinine sulfate (Φ = 0.51) in 0.5 M H2SO4 as the standard.
2.2.2 Calculation of the binding stoichiometry and association constant. The binding stoichiometry of the metal ion to the sensor 1 was determined using Job plot experiments.31 The association constant (Ka) of the metal ion binding to the sensor 1 was determined from the fluorescence titration data based on the reported Benesi–Hildebrand equation.32,33
2.3. Quantum chemical calculation
Quantum chemical calculations were performed using Gaussian 09 program.34 The ground state structure of the sensor 1 was optimized based on density functional theory (DFT) using B3LYP functional35,36 and 6-311G(d,p) basis set. Frequency calculations were carried out on all optimized geometries to ensure that the obtained structures represent local minima without imaginary frequencies. Time-dependent (TD) DFT calculations were also performed using the same basis set as those in the geometry optimization.
2.4. Cell culture and fluorescence imaging
The cell lines were cultured in RPMI-1640 medium supplemented with 10% (v/v) calf serum, penicillin (100 U mL−1), and streptomycin (100 mg mL−1). The cells were maintained at 37 °C in a humidified atmosphere containing 5% CO2.
To investigate the influence of Cu2+ and Cr3+ on the fluorescence intensity of the sensor 1 in cell level, MCF-7 cells were seeded in 12 laser confocal fluorescence microscopy culture dishes with a density of 3 × 105 cells per well and subsequently incubated at 37 °C. After 24 h of cell attachment, the 12 dishes were separated into 4 groups randomly. The cells in first group without treatment with any samples were utilized as the contrast. The cells in the second group were treated with 40 μL of the sensor 1 (10 μM) in a HEPES–DMSO (9
:
1) solution and then incubated for 30 min at 37 °C. Phosphate-buffered saline (PBS) was utilized to wash the cells for three times. Following the experimental steps for the second group, the cells in the third group were incubated with 20 μL of the Cu2+ solution (30 μM) for 30 min at 37 °C. The cells in the fourth group were further treated with 20 μL of the Cr3+ solution (30 μM) for 30 min at 37 °C. After washing three times with PBS, the cells were imaged using a laser confocal fluorescence microscopy.
2.5. Synthesis of the sensor 1
7-Diethylaminocoumarin-3-carboxylic acid (10 mmol, 2.61 g) and 8-aminoquinoline (10 mmol, 1.44 g) were dissolved in 100 mL chloroform including dicyclohexyl carbodiimide (DCC) (10 mmol, 2.06 g) and 4-dimethylaminopyridine (DMAP) (50 mg). The solution was stirred for 6 hours at room temperature, and then the white precipitate dicyclohexylurea was filtered to obtain the clarified yellow solution which was extracted with water three times and dried with anhydrous sodium sulfate. Subsequently, the solvent was removed using the rotary evaporation to obtain a crude product which was further purified by the silica gel column chromatography (ethyl acetate
:
dichloromethane = 1
:
10, v/v) to give 1 as a yellow solid in 61.5% yield. mp 222.5–224.3 °C; 1H NMR (500 MHz, CDCl3): δ (ppm): 1.25 (t, 6H, J = 7.2 Hz, –CH3), 3.47 (q, 4H, J = 7.2 Hz, –CH2–), 6.55 (s, 1H, Ar–H), 6.67 (d, 1H, J = 9.0 Hz, Ar–H), 7.45–7.48 (m, 2H, Ar–H), 7.53–7.59 (m, 2H, Ar–H), 8.17 (d, 1H, J = 8.25 Hz, Ar–H), 8.84 (s, 1H, Ar–H), 8.99–9.01 (m, 2H, Ar–H), 12.76 (s, 1H, N–H); 13C NMR (125 MHz, CDCl3): δ (ppm): 162.5, 161.6, 157.9, 152.7, 148.9, 148.4, 139.6, 136.1, 135.6, 131.2, 128.1, 127.2, 121.9, 121.5, 117.7, 111.1, 109.9, 108.6, 96.8, 45.1, 12.5; FTIR (cm−1) 2971.5, 2925.2, 1710.9, 1650.5, 1612.8, 1570.7, 1506.9, 1420.7, 1350.2, 1263.4, 1188.3, 1133.7, 1076.9, 821.5, 788.6; HRMS calcd 388.1661, found 388.1657.
3. Results and discussion
3.1. Synthesis and characterization of the sensor 1
The sensor 1 was obtained by the reaction of 7-diethylaminocoumarin-3-carboxylic acid and 8-aminoquinoline where a new amide bond was formed between the coumarin and quinoline moiety (Scheme 1). To elucidate the structure of the sensor 1, the DFT calculations were carried out and the lowest energy structure of the sensor 1 is presented in Fig. 1. The sensor 1 forms a large coplanar structure over quinoline and coumarin moieties and shows a cis conformer though the amide bond. In addition, the shortened Namine–C bond length (1.37516 Å) and Calkyl–Namine–Calkyl or Caryl–Namine–Caryl angle of almost 120° suggest the lone pairs of amine N are delocalized and a p–π conjugated system is hence formed. Consequently, the sensor 1 presents a greater π conjugate system of electronic delocalization than its precursor coumarin.
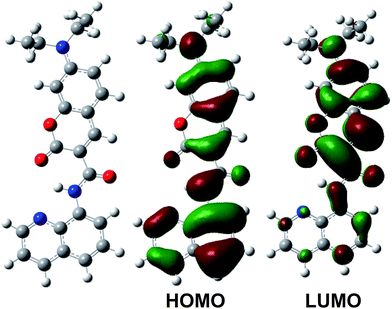 |
| Fig. 1 The optimized geometry (left) and significant frontier molecular orbitals (right) of the sensor 1 using B3LYP functional and 6-311G(d,p) basis set (blue atom, N; red atom, O). | |
The synthesized yellow compound shows a maximum absorption band at 445 nm which undergoes a red-shift compared to its precursor,37 indicating a greater electronic delocalization effect on the sensor 1 in good agreement with the above DFT-data. TD-DFT calculations predict that the experimentally observed maximum absorption band at 445 nm is predominately originating from the HOMO to LUMO transition (Fig. 1), where the HOMO delocalizes over the entire π system, whereas the LUMO has a significant contribution from the coumairn moiety. Accordingly, the maximum absorption of the sensor 1 presents a charge transfer π–π* transition from the occupied quinoline to unoccupied coumarin orbitals, which may cause the red shift of the absorption band. The quantum yield of the sensor 1 was 0.109 determined in a HEPES–DMSO buffer solution (0.1 M) with the emission band at 491 nm (Fig. 2).
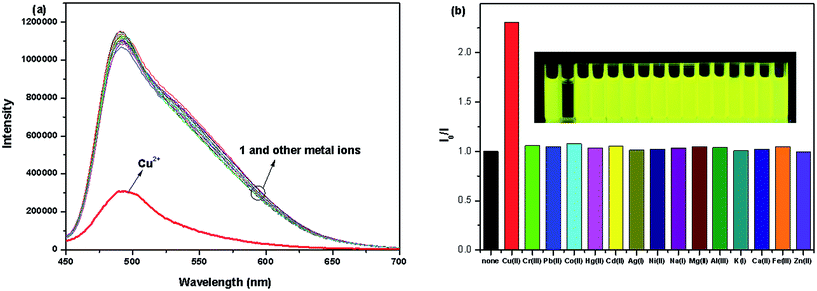 |
| Fig. 2 (a) Fluorescence changes of the sensor 1 (10 μM) upon addition of 3.0 equiv. of individual metal ions in 0.1 M HEPES–DMSO (9 : 1, v/v, pH = 7.2) solution. (b) The relative fluorescent intensity of I0/I at 491 nm, where I0 and I are the fluorescent intensity of the sensor 1 (10 μM) in the absence and presence of metal ions, respectively. Inset: a picture of fluorescence changes of the sensor 1 (10 μM) upon addition of 3.0 equiv. of individual metal ions in 0.1 M HEPES–DMSO (9 : 1, v/v, pH = 7.2) solution. | |
3.2. Spectroscopic properties
The recognition ability of the sensor 1 was obtained by mixing it with various metal ions including K+, Ca2+, Na+, Mg2+, Al3+, Zn2+, Fe3+, Pb2+, Hg2+, Ag+, Co2+, Ni2+, Cd2+, Cu2+ and Cr3+ in aqueous solution using the fluorescence and UV-vis spectrophotometric titration. When excited at 437 nm, only Cu2+ caused a significant quenching (Φ = 0.024) in the fluorescence intensity of the sensor 1 which could be attributed to the coordination of the paramagnetic Cu2+ ions (Fig. 2). Upon incremental addition of Cu2+, the fluorescence intensity at 491 nm decreased gradually and then reached the minimum after adding 5 equivalents of Cu2+ (Fig. 3). The linear relationship of the fluorescence titration (Fig. 3) and Job's plot (Fig. 4) suggest the stoichiometric ratio of the sensor 1 with Cu2+ appears to be 1 to 1. The association constant Ka of the sensor 1 for Cu2+ was 3.65 × 103 M−1 (Fig. S1†) and the detection limit of the sensor 1 for Cu2+ was 2.5 μM which was reasonable for the detection of the copper ion in the blood (15.7–23.6 μM)38,39 and drinking water (∼30 μM).40 After binding with Cu2+, the absorbance at 445 nm increased and the maximum absorption band was shifted to 455 nm which led the color change from light yellow to yellow (Fig. 5 and S2†). The fluorescence changes of the sensor 1 in the absence and presence of Cu2+ in different pH values were evaluated. As shown in Fig. S3,† Cu2+ caused the fluorescence quenching over a wide pH range of 4.5–7.5. This indicates that the sensor 1 can be potentially applicable as a selective sensor for Cu2+ in near neutral and acidic medium, which is promising in both the environmental and biological application.
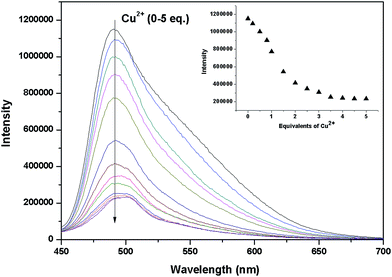 |
| Fig. 3 Fluorescence response of the sensor 1 (10 μM) to different equivalents of Cu2+ (0, 0.2, 0.5, 0.8, 1.0, 1.5, 2.0, 2.5, 3.0, 3.5, 4.0, 4.5, 5.0 equiv.) in 0.1 M HEPES–DMSO (9 : 1, v/v, pH = 7.2) solution. | |
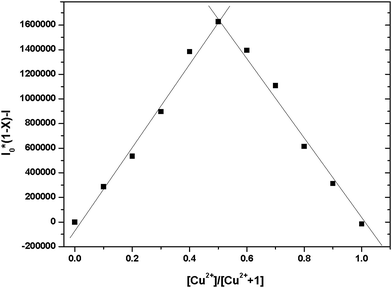 |
| Fig. 4 Job's plot for the sensor 1 vs. Cu2+ in 0.1 M HEPES–DMSO (9 : 1, v/v, pH = 7.2) solution. The total concentration of the sensor 1 and Cu2+ was 30 μM. | |
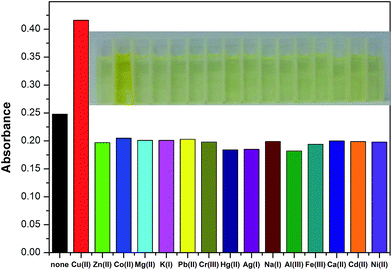 |
| Fig. 5 Absorption changes of the sensor 1 (10 μM) upon addition of 3.0 equiv. of individual metal ions in 0.1 M HEPES–DMSO (9 : 1, v/v, pH = 7.2) solution; inset: a picture of absorption changes of the sensor 1 (10 μM) upon addition of 3.0 equiv. of individual metal ions in 0.1 M HEPES–DMSO (9 : 1, v/v, pH = 7.2) solution. | |
To further explore the influence of other metal ions on the 1–Cu2+ complex, the competitive experiments were carried out with other metal ions (30 μM) in the presence of Cu2+ (30 μM) and the sensor 1 (10 μM) (Fig. S4†). The observed fluorescence spectra of K+, Ca2+, Na+, Mg2+, Al3+, Zn2+, Fe3+, Pb2+, Hg2+, Ag+, Co2+, Ni2+ and Cd2+ in the presence of the 1–Cu2+ complex were similar to that caused with the 1–Cu2+ complex alone. Attractively, the addition of Cr3+ caused a 3.8-fold enhancement of the fluorescence intensity of the 1–Cu2+ complex (Fig. 6, 7 and S5†). The quantum yield of the emission band was increased to 0.064.
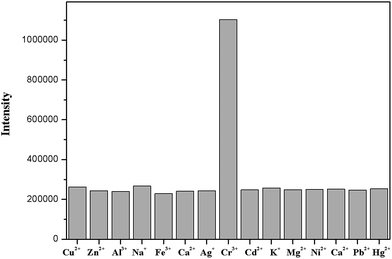 |
| Fig. 6 Fluorescence response of the sensor 1 (10 μM) and Cu2+ (30 μM) in the presence of other different metal ions (30 μM) in 0.1 M HEPES–DMSO (9 : 1, v/v, pH = 7.2) solution. | |
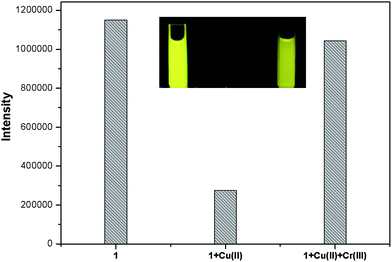 |
| Fig. 7 Fluorescence response of the sensor 1 (10 μM) and Cu2+ (30 μM) in the presence of Cr3+ (30 μM) in 0.1 M HEPES–DMSO (9 : 1, v/v, pH = 7.2) solution. | |
Fluorescence titration experiments were performed to further evaluate the sensing ability of 1–Cu2+ (10 μM) to Cr3+ (Fig. 8). The fluorescence intensity of the 1–Cu2+ solution increased gradually with the addition of Cr3+ and remained fairly constant until 3.0 equivalents of Cr3+ were added. According to the Benesi–Hildebrand equation, the association constant of 1–Cu2+ with Cr3+ was calculated to be 5.4 × 104 M−1 from the fluorescence titration curve (Fig. S6†). The association constant of 1–Cu2+ for Cr3+ is ca. 14.8-fold of that for the sensor 1 with Cu2+, suggesting the increased fluorescence may originate from the replacement of Cu2+ by Cr3+. The linear relationship of the fluorescence titration of 1–Cu2+ also indicated a 1
:
1 binding mode for 1–Cu2+ with Cr3+ which was further supported by the UV-vis titration of Cr3+ to 1–Cu2+ (Fig. S7†). The above observations suggest that Cu2+ in the 1–Cu2+ complex could be replaced by only Cr3+, indicating the 1–Cu2+ complex has a good selectivity for Cr3+ and could be an efficient “off–on” sensor for Cr3+. A schematic for the analysis of the mixed metal ions using the sensor 1 is shown in Scheme 2. When the resultant 1–Cu2+ complex in situ is used to as the sensor, existence of Cr3+ would turn on the fluorescence of the 1–Cu2+ complex. In contrast, other metal ions including Cu2+ would have no obvious influence on the fluorescence intensity of the 1–Cu2+ complex. The sensor 1 can detect the Cu2+ ion from the solution of the mixed metal ions without Cr3+ based on the fluorescence quenching.
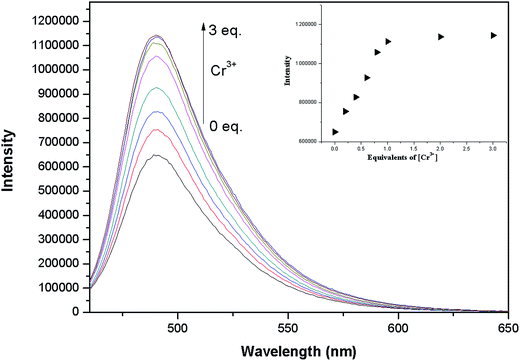 |
| Fig. 8 Fluorescence changes of 1-Cu2+ (10 μM) on addition of different equivalents of Cr3+ in 0.1 M HEPES–DMSO (9 : 1, v/v, pH = 7.2) solution. Inset: the fluorescence intensity of 1–Cu2+ (10 μM) at 491 nm as a function of equivalents of Cr3+ in 0.1 M HEPES–DMSO (9 : 1, v/v, pH = 7.2) solution. | |
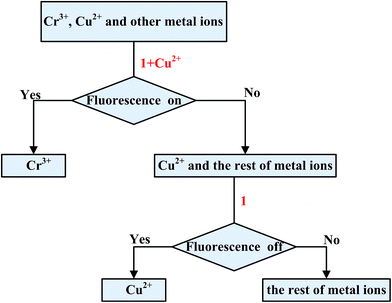 |
| Scheme 2 Schematic for the analysis of the mixed metal ions using the sensor 1. | |
3.3. Possible sensing mechanism
To get insight of the binding mode of the sensor 1 with Cu2+, IR, NMR and MS were first employed. IR spectra of the sensor 1 and 1–Cu2+ are shown in Fig. 9. The sensor 1 exhibits a band of the medium intensity at around 3440 cm−1. This band can be assigned to v NH which is also be observed in the spectrum of 1–Cu2+. Two bands appearing at 1701.9 and 1650.5 cm−1 are the C
O stretching vibration of the ester carbonyl and amide carbonyl groups, respectively. In the spectrum of 1–Cu2+, the C
O vibration band of the ester carbonyl is shifted to the lower frequency by ca. 48 cm−1 suggesting the binding of the oxygen of the ester carbonyl group with Cu2+, whereas there are not obvious differences in the C
O stretching band of the amide carbonyl group before and after the addition of Cu2+.
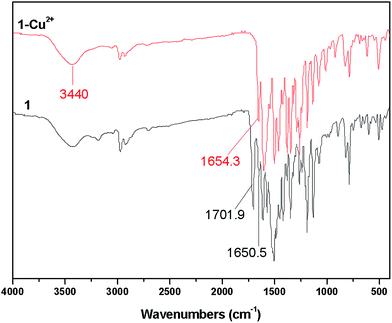 |
| Fig. 9 FT-IR spectra of the sensor 1 and 1–Cu2+ with the KBR compression method. | |
The 1H NMR spectrum of the sensor 1 with the addition of Cu2+ reveals that the amide hydrogen (NH) at 12.6 ppm (Fig. S8†) is still held which is in good agreement with the IR observation. Due to the paramagnetic Cu2+ ion, the signals of 1H NMR are broadened and hence distinct shifts of proton signals are not observed. As discussed above, the formation of 1–Cu2+ is further confirmed using HRMS (Fig. S9†) where the peak at m/z 449.0808 corresponds to [1 − Cu − H]+ and moreover a peak at m/z 527.0953 corresponding to [1 − Cu + DMSO − H]+ is also observed, indicating one solvent involves in the coordination to 1–Cu2+.
To more clearly understand the structure of 1–Cu2+, XPS spectra were carried out and the standard samples, coumarin 120 and 8-aminoquinoline, were also analyzed using XPS to help further assign the O and N groups. The results are presented in Fig. S10† and the corresponding assignments are listed in Table 1. When Cu2+ is bound to the sensor 1, the binding energy of the oxygen of the ester carbonyl group in the sensor 1 (528.33 eV) is shifted to the higher energy by 0.6 eV, while those of nitrogen atoms of amide and aromatic groups undergo the lower energy shift by 0.55 eV and 0.19 eV in 1–Cu2+, respectively, which suggest these atoms have involved in the coordination to Cu2+, in good agreement with the above IR and NMR analyses.
Table 1 XPS results of the sensor 1 and 1–Cu2+a
Sample |
1 |
1–Cu2+ |
Coumarin 120 |
8-Aminoquinoline |
Assignment |
Asterisk (*) presents the assigned atom. |
N1s(FWHM) |
397.31(1.10) |
396.76(1.01) |
— |
— |
–HN*–C O |
396.68(0.78) |
396.49(0.92) |
— |
396.80(1.47) |
Aromatic N* |
396.06(0.94) |
395.96(0.98) |
396.19(1.08) |
395.96(1.02) |
*NH2 or *NEt2 |
O1s(FWHM) |
530.95(1.58) |
531.05(1.52) |
530.89(1.72) |
— |
–O*–C O |
529.36(1.55) |
529.47(0.88) |
— |
— |
–HN–C O* |
528.33(1.52) |
528.93(1.0) |
528.39(1.24) |
— |
–O–C O* |
— |
529.66(1.89) |
— |
— |
NO3* |
Based on the analysis of the fluorescence and UV-vis titration, Job's plot, IR, NMR, HRMS and XPS, the possible binding mode of the sensor 1 with Cu2+ in solvent media is proposed as shown in Fig. 10, where a four-coordination copper(II) complex is formed with three coordination sites in the sensor 1 and one coordination site from the solvent.
 |
| Fig. 10 Possible sensing mechanism of the sensor 1 for the recognition of Cu2+ and Cr3+. | |
To explore the possible mechanism of the fluorescence recovery of the 1–Cu2+ complex after the addition of Cr3+, NMR and IR were also carried out as presented in Fig. S8 and S11.† Similar to the 1H NMR spectrum of 1–Cu2+, the paramagnetic Cr3+ ion also causes the broader proton signals, hence no significant shifts of proton signals are observed with the held amide hydrogen at 12.6 ppm. Different from the IR characteristics of the 1–Cu2+ complex, IR spectrum of 1–Cu2+ with Cr3+ shows that the C
O stretching vibration of the ester carbonyl group in the ligand is shifted back from ca. 1654 cm−1 to ca. 1701 cm−1 which distinctly suggests that copper(II) may be replaced by chromium(III). In addition, individual chromium(III) ions were also added to the sensor 1 and its IR spectrum shows the same band at ca. 1702 cm−1 which can be assigned as the C
O stretching vibration of the ester carbonyl group in the ligand. The above observations indicate that the oxygen of the ester carbonyl group in the ligand may not participate in the coordination to chromium(III). IR spectra of 1–Cr3+ and 1–Cu2+ in DMSO were also recorded and shown in Fig. S11† where the bands of NO3 in 1–Cr3+ complex are different from those in 1–Cu2+ complex with three bands at ca. 1437, 1408, 1318 cm−1 vs. ca. 1350 cm−1 for 1–Cr3+ and 1–Cu2+, respectively. As the analysis for the four-coordination 1–Cu2+ complex in the solution, one solvent with the sensor 1 is bound with copper(II) with the free NO3 groups in good agreement with its IR observation in DMSO, whereas NO3 groups may involve in the binding with chromium(III) in 1–Cr3+.
Overall, referring to the analysis of the fluorescence, UV-vis titration and the larger association constant of 1–Cu2+ for Cr3+ vs. the sensor 1 for Cu2+, the possible sensing mechanism in solvent media is proposed that Cr3+ replaced Cu2+ and then was bound to the sensor 1 as shown in Fig. 10.
3.4. Living cell imaging
To further demonstrate the practical application of the sensor 1 for the detection of Cu2+ and Cr3+ in biological samples, the fluorescence imaging was carried out in MCF-7 breast cancer living cells as showed in Fig. 11. MCF-7 cells themselves have not showed obvious fluorescence. When the cells were treated with 10 μM of the sensor 1 for 30 min at 37 °C, the intracellular bright green fluorescence was observed in MCF-7 cells. Subsequently, upon treating above cells with an excessive amount of Cu2+ (30 μM) for 30 min at 37 °C, the fluorescence intensity in MCF-7 cells was significantly quenched. Finally, when 30 μM of the Cr3+ solution was further added, the intracellular fluorescence was easily recovered and showed a green fluorescence imaging. The bright field images also confirmed that cells were visible before and after the addition of the sensor 1, Cu2+ and Cr3+. These results indicate that the sensor 1 has excellent cell-membrane permeability. Moreover, the sensor 1 is applicable for the recognition of Cu2+ and Cr3+ in living cells.
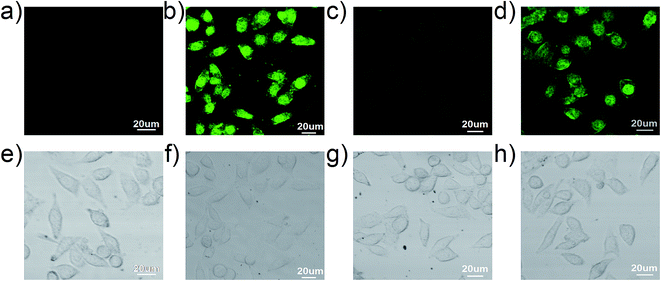 |
| Fig. 11 Confocal fluorescence images in MCF-7 cells. (a) Cells untreated with any samples as blank test; (b) cells incubated with 10 μM of the sensor 1 for 30 min at 37 °C; (c) cells further incubated with 30 μM of the Cu2+ solution for another 30 min at 37 °C; (d) cells incubated with 10 μM of the sensor 1 and 30 μM of the Cu2+ solution, and further incubated with 30 μM of the Cr3+ solution for 30 min at 37 °C; (e) bright field image of cells in panel a; (f) bright field image of cells in panel b; (g) bright field image of cells in panel c; (h) bright field image of cells in panel d. | |
4. Conclusion
In conclusion, a new fluorescence sensor 1 has been synthesized, which showed the selective recognition for Cu2+ by fluorescence quenching. The resultant 1–Cu2+ complex can serve as a fluorescent turn-on sensor for Cr3+ via the replacement of Cu2+. The binding mode of the sensor 1 with Cu2+ or Cr3+ was 1
:
1 to form the four-coordination 1–Cu2+ complex or six-coordination 1–Cr3+ complex.
This “on–off–on” switching sensor was successfully applied in the live cell fluorescence imaging and therefore further demonstrates its greatly potential applicability for the recognition of Cu2+ and Cr3+ in living cells.
Acknowledgements
Our work was supported by Zhejiang Provincial Natural Science Foundation of China (Grant No. LY15B070004 and LY12B07010) and National Natural Science Foundation of China (Grant No. 20807037 and 81371684).
References
- Y. Jeong and J. Yoon, Recent progress on fluorescent chemosensors for metal ions, Inorg. Chim. Acta, 2012, 381, 2–14 CrossRef CAS PubMed.
- L. Prodi, F. Bolletta, M. Montalti and N. Zaccheroni, Luminescent chemosensors for transition metal ions, Coord. Chem. Rev., 2000, 205, 59–83 CrossRef CAS.
- J. Du, M. Hu, J. Fan and X. Peng, Fluorescent chemodosimeters using “mild” chemical events for the detection of small anions and cations in biological and environmental media, Chem. Soc. Rev., 2012, 41, 4511–4535 RSC.
- R. Uauy, M. Olivares and M. Gonzalez, Essentiality of copper in humans, Am. J. Clin. Nutr., 1998, 67, 952S–959S CAS.
- K. J. Barnham, C. L. Masters and A. I. Bush, Neurodegenerative diseases and oxidative stress, Nat. Rev. Drug Discovery, 2004, 3, 205–214 CrossRef CAS PubMed.
- S. P. Wu, T. H. Wang and S. R. Liu, A highly selective turn-on fluorescent chemosensor for copper(II) ion, Tetrahedron, 2010, 66, 9655–9658 CrossRef CAS PubMed.
- N. Aksuner, E. Henden, I. Yilmaz and A. Cukurovali, A highly sensitive and selective fluorescent sensor for the determination of copper(II) based on a Schiff base, Dyes Pigm., 2009, 83, 211–217 CrossRef CAS PubMed.
- X. R. He, H. B. Liu, Y. L. Li, S. Wang, Y. J. Li, N. Wang, J. C. Xiao, X. Xun and D. B. Zhu, Gold Nanoparticle-Based Fluorometric and Colorimetric Sensing of Copper(II) Ions, Adv. Mater., 2005, 17, 2811–2815 CrossRef CAS PubMed.
- S. Pu, L. Ma, G. Liu, H. Ding and B. Chen, A multiple switching diarylethene with a phenyl-linked rhodamine B unit and its application as chemosensor for Cu2+, Dyes Pigm., 2015, 113, 70–77 CrossRef CAS PubMed.
- H. F. Wang and S. P. Wu, A pyrene-based highly selective turn-on fluorescent sensor for copper(II) ions and its application in living cell imaging, Sens. Actuators, B, 2013, 181, 743–748 CrossRef CAS PubMed.
- M. Xu, C. Yin, F. Huo, Y. Zhang and J. Chao, A highly sensitive “ON–OFF–ON” fluorescent probe with three binding sites to sense copper ion and its application for cell imaging, Sens. Actuators, B, 2014, 204, 18–23 CrossRef CAS PubMed.
- A. Kumar, V. Kumar, U. Diwan and K. K. Upadhyay, Highly sensitive and selective naked-eye detection of Cu2+ in aqueous medium by a ninhydrin–quinoxaline derivative, Sens. Actuators, B, 2013, 176, 420–427 CrossRef CAS PubMed.
- A. Kumar, V. Vanita, A. Walia and S. Kumar, N,N–dimethylaminoethylaminoanthrone A chromofluorogenic chemosensor for estimation of Cu2+ in aqueous medium and HeLa cells imaging, Sens. Actuators, B, 2013, 177, 904–912 CrossRef CAS PubMed.
- X. Q. Chen, M. J. Jou, H. Lee, S. Z. Kou, J. Lim, S. W. Nam, S. Park, K. M. Kim and J. Yoon, New fluorescent and colorimetric chemosensors bearing rhodamine and binaphthyl groups for the detection of Cu2+, Sens. Actuators, B, 2009, 137, 597–602 CrossRef CAS PubMed.
- M. Zayed and T. Norman, Chromium in the environment: factors affecting biological remediation, Plant Soil, 2003, 249, 139–156 CrossRef.
- S. A. Katz and H. Salem, The biological and environmental chemistry of chromium, VCH Publishers, New York, 1994 Search PubMed.
- S. Samanta, S. Goswami, A. Ramesh and G. Das, A new fluorogenic probe for solution and intra-cellular sensing of trivalent cations in model human cells, Sens. Actuators, B, 2014, 194, 120–126 CrossRef CAS PubMed.
- S. Wu, K. Zhang, Y. Wang, D. Mao, X. Liu, J. Yu and L. Wang, A novel Cr3+ turn-on probe based on naphthalimide and BINOL framework, Tetrahedron Lett., 2014, 55, 351–353 CrossRef CAS PubMed.
- P. Xie, F. Guo, Y. Xiao, Q. Jin, D. Yao and Z. Huang, A fluorescent chemosensor based on rhodamine for Cr3+ in red spectral region in aqueous solutions and living cells, J. Lumin., 2013, 140, 45–50 CrossRef CAS PubMed.
- M. Wang, J. Wang, W. Xue and A. Wu, A benzimidazole-based ratiometric fluorescent sensor for Cr3+ and Fe3+ in aqueous solution, Dyes Pigm., 2013, 97, 475–480 CrossRef CAS PubMed.
- F. Hu, B. Zheng, D. Wang, M. Liu, J. Du and D. Xiao, A novel dual-switch fluorescent probe for Cr(III) ion based on PET–FRET processes, Analyst, 2014, 139, 3607–3613 RSC.
- J. G. Zhang, L. Zhang, Y. L. Wei, J. B. Chao, S. B. Wang, S. M. Shuang, Z. W. Cai and C. Dong, A selective carbazole-based fluorescent probe for chromium(III), Anal. Methods, 2013, 5, 5549–5554 RSC.
- L. Tang, J. Zhao, M. Cai, P. Zhou, K. Zhong, S. Hou and Y. Bian, An efficient sensor for relay recognition of Zn2+ and Cu2+ through fluorescence ‘off–on–off’ functionality, Tetrahedron Lett., 2013, 54, 6105–6109 CrossRef CAS PubMed.
- Y. M. Liu, Q. Fei, H. Y. Shan, M. H. Cui, Q. Liu, G. D. Feng and Y. F. Huan, A novel fluorescent ‘off–on–off’ probe for relay recognition of Zn2+ and Cu2+ derived from N,N-bis(2-pyridylmethyl)amine, Analyst, 2014, 139, 1868–1875 RSC.
- Z. Li, L. Zhang, L. Wang, Y. Guo, L. Cai, M. Yu and L. Wei, Highly sensitive and selective fluorescent sensor for Zn2+/Cu2+ and new approach for sensing Cu2+ by central metal displacement, Chem. Commun., 2011, 47, 5798–5800 RSC.
- Y. Y. Yan, Z. P. Che, X. Yu, X. Y. Zhi, J. J. Wang and H. Xu, Fluorescence ‘on–off–on’ chemosensor for sequential recognition of Fe3+ and Hg2+ in water based on tetraphenylethylene motif, Bioorg. Med. Chem., 2013, 21, 508–513 CrossRef CAS PubMed.
- L. Xue, Q. Liu and H. Jiang, Ratiometric Zn2+ fluorescent sensor and new approach for sensing Cd2+ by ratiometric displacement, Org. Lett., 2009, 11, 3454–3457 CrossRef CAS PubMed.
- J. Wang, W. Lin and W. Li, Single fluorescent probe displays a distinct response to Zn2+ and Cd2+, Chem.–Eur. J., 2012, 18, 13629–13632 CrossRef CAS PubMed.
- D. Maity and T. Govindaraju, A differentially selective sensor with fluorescence turn-on response to Zn2+ and dual-mode ratiometric response to Al3+ in aqueous media, Chem. Commun., 2012, 48, 1039–1041 RSC.
- E. Karakuş, M. Üçüncü and M. Emrullahoğlu, A rhodamine/BODIPY-based fluorescent probe for the differential detection of Hg(II) and Au(III), Chem. Commun., 2014, 50, 1119–1121 RSC.
- A. Senthilvelan, I. Ho, K. C. Chang, G. H. Lee, Y. H. Liu and W. S. Chung, Cooperative recognition of a copper cation and cnion by a calix [4] arene substituted at the lower rim by a β-amino-α, β-unsaturated ketone, Chem.–Eur. J., 2009, 15, 6152–6160 CrossRef CAS PubMed.
- H. A. Benesi and J. H. Hildebrand, A spectrophotometric investigation of the interaction of iodine with aromatic hydrocarbons, J. Am. Chem. Soc., 1949, 71, 2703–2707 CrossRef CAS.
- R. B. Singh, S. Mahanta and N. Guchhait, Spectral modulation of charge transfer fluorescence probe encapsulated inside aqueous and non-aqueous β-cyclodextrin nanocavities, J. Mol. Struct., 2010, 963, 92–97 CrossRef CAS PubMed.
- M. J. Frisch, G. W. Trucks, H. B. Schlegel, G. E. Scuseria, M. A. Robb, J. R. Cheeseman, G. Scalmani, V. Barone, B. Mennucci, G. A. Petersson, H. Nakatsuji, M. Caricato, X. Li, H. P. Hratchian, A. F. Izmaylov, J. Bloino, G. Zheng, J. L. Sonnenberg, M. Hada, M. Ehara, K. Toyota, R. Fukuda, J. Hasegawa, M. Ishida, T. Nakajima, Y. Honda, O. Kitao, H. Nakai, T. Vreven, J. A. Montgomery Jr, J. E. Peralta, F. Ogliaro, M. Bearpark, J. J. Heyd, E. Brothers, K. N. Kudin, V. N. Staroverov, R. Kobayashi, J. Normand, K. Raghavachari, A. Rendell, J. C. Burant, S. S. Iyengar, J. Tomasi, M. Cossi, N. Rega, J. M. Millam, M. Klene, J. E. Knox, J. B. Cross, V. Bakken, C. Adamo, J. Jaramillo, R. Gomperts, R. E. Stratmann, O. Yazyev, A. J. Austin, R. Cammi, C. Pomelli, J. W. Ochterski, R. L. Martin, K. Morokuma, V. G. Zakrzewski, G. A. Voth, P. Salvador, J. J. Dannenberg, S. Dapprich, A. D. Daniels, O. Farkas, J. B. Foresman, J. V. Ortiz, J. Cioslowski and D. J. Fox, Gaussian 09, Gaussian Inc., Wallingford, CT 2009 Search PubMed.
- A. D. Becke, Density-functional exchange-energy approximation with correct asymptotic behavior, Phys. Rev. A, 1988, 38, 3098–3100 CrossRef CAS.
- J. P. Perdew, Density functional approximation for the correlation energy of the inhomogeneous electron gas, Phys. Rev. B: Condens. Matter Mater. Phys., 1986, 33, 8822–8824 CrossRef.
- H. Zhang, T. Yu, Y. Zhao, D. Fan, L. Chen, Y. Qiu and C. Yang, Crystal structure and photoluminescence of 7-(N,N′-diethylamino)-coumarin-3-carboxylic acid, Spectrochim. Acta, Part A, 2008, 69, 1136–1139 CrossRef PubMed.
- J. Wang, L. Long, D. Xie and X. Song, Cu2+-selective “Off-On” chemsensor based on the rhodamine derivative bearing 8-hydroxyquinoline moiety and its application in live cell imaging, Sens. Actuators, B, 2013, 177, 27–33 CrossRef CAS PubMed.
- H. S. Jung, P. S. Kwon, J. S. Kim, C. S. Hong, J. W. Kim, S. Yan, J. Y. Lee, J. H. Lee, T. Joo and J. S. Kim, Coumarin-derived Cu2+-selective fluorescence sensor: synthesis, mechanisms, and applications in living cells, J. Am. Chem. Soc., 2009, 131, 2008–2012 CrossRef CAS PubMed.
- T. G. Jo, Y. J. Na, J. J Lee, M. M. Lee, S. Y. Lee and C. Kim, A diaminomaleonitrile based selective colorimetric chemosensor for copper(II) and fluoride ions, New J. Chem., 2015, 39, 2580–2587 RSC.
Footnote |
† Electronic supplementary information (ESI) available: NMR spectra, FT-IR spectra and XPS spectra; Benesi–Hildebrand plot; pH titration curves; UV-vis and fluorescence spectra and DFT-optimized structure. See DOI: 10.1039/c5ra13782a |
|
This journal is © The Royal Society of Chemistry 2015 |