DOI:
10.1039/C5RA13360B
(Paper)
RSC Adv., 2015,
5, 71462-71471
Improved saccharification of pilot-scale acid pretreated wheat straw by exploiting the synergistic behavior of lignocellulose degrading enzymes
Received
8th July 2015
, Accepted 17th August 2015
First published on 17th August 2015
Abstract
Requirement of high enzyme dosage for lignocellulosic biomass hydrolysis is one of the challenges for the viability of the second generation bioethanol technology. Here, an optimal enzyme mixture was developed by partially replacing the cellulase proportion with accessory enzymes (β-glucosidase, xylanase, pectinase, laccase) and its hydrolytic performance was compared with different commercial counterparts for the saccharification of pretreated wheat straw (PWS) using a 250 kg per day continuous pilot plant. Maximum degree of synergism was observed with xylanase followed by pectinase, laccase, and β-glucosidase. The statistically optimized enzyme mixture enhanced hydrolysis by 51.23% and 40.66% in 6 h and 24 h, respectively. This study elucidates that presence of even small amount of oligomers and cellobiose pose a strong inhibition for the enzymes. Therefore, development of an optimal enzyme formulation is a sustainable approach to reduce overall enzyme loading for biomass saccharification.
1. Introduction
Lignocellulosic biomasses (LCB) are considered as clean and renewable sources of energy. They have significant potential to reduce our fossil fuel dependence. LCB have complex structure, mainly constituted of cellulose, hemicellulose (including xylose, arabinose, glucose, galactose, rhamnose and mannose) and lignin. Because of its recalcitrant nature, pretreatment at high temperature and pressure is required to break down the lignin and hemicellulose. Dilute acid pretreatment is among the most studied pretreatment methods which could be applied to a variety of feedstocks (hardwood, softwood, agricultural residues etc.).1 Apart from this, wet explosion (WEx) is another promising method for thermochemical pretreatment of LCB where, additional features of oxygen supplementation and explosive decompression have been incorporated to adjust with different biomass feedstocks and subsequent bio-catalytic and microbial processes.2 After LCB pretreatment, enzymes could easily access the polysaccharides and hydrolyze them into monomeric sugars.
Usually a complex of secreted enzymes from filamentous fungi (particularly Trichoderma sp.) is used for LCB hydrolysis. Such enzyme complexes generally contain lower amounts of accessory enzymes and β-glucosidases responsible for degrading non-cellulosic polysaccharides and cellobiose. As cellulose is the predominant polysaccharide in LCB, therefore; significant research has been carried out to understand and improve its hydrolysis using cellulases. It has been recently recognized that the hydrolytic efficiency of fungal cellulase complexes determined by using a model cellulosic substrates (like filter paper, carboxy methyl cellulose (CMC) or avicel) cannot provide a reliable indication of its performance on pretreated biomass. This is due to the fact that, biomass substrates are composed of lignin and a number of mutually entangled and chemically bonded carbohydrate polymers that require multiple enzymes working together synergistically for complete hydrolysis. Therefore, enzyme mixtures with similar cellulase activity may show differences in pretreated biomass hydrolysis.
Enzymatic hydrolysis of biomass involves synergistic action of a group of functionally different enzymes. In general, endoglucanases (EC 3.2.1.4) and exoglucanases (cellobiohydrolases; CBHs) break down cellulose at solid–liquid interface,3 whereas accessory enzymes such as hemicellulases, acetyl xylan esterase, arabinofuranosidase, feruloyl esterase and p-coumaroyl esterase help in cleaving the physical shields that cover cellulose microfibrils.4,5 Therefore, the accessibility of the cellulose surface to cellulases and the subsequent efficacy of these enzymes have been identified as important factors that determine the hydrolysis yield. Lignin and hemicellulose act as a physical barrier for the enzymes thereby limiting the cellulose accessibility. Furthermore, enzymes bind non-specifically/non-productively with the lignin by hydrophobic interactions. Therefore, removal of hemicellulose and lignin during pretreatment may improve the enzymatic accessibility and LCB hydrolysis yield.7,8 Xylose and soluble xylo-oligomers released from hemicelluloses during enzymatic hydrolysis pose as additional barrier to enzymatic action by competitively inhibiting the cellulase activity.9 Therefore, significantly high enzyme doses are required to obtain reasonable biomass hydrolysis. Although, the costs of enzyme preparations from Genencor, USA and Novozymes, Denmark have been reduced significantly (20-fold) over the past decade,10 still biomass hydrolysis remains a key cost barrier and further cost reduction is essential for the commercial viability of the process.
There are significant quantitative and qualitative variations in non-cellulosic polysaccharide components of biomass derived from agricultural residues (such as wheat straw, rice straw, cotton stalks) and other purpose grown crops (like switchgrass, poplar, corn and softwoods such as spruce and pine). In addition, pretreatment methodology also introduces other structural and compositional differences in pretreated biomass. Therefore, development of customized enzyme solutions based on feedstock and pretreatment rationale may help in achieving the optimum biomass hydrolysis. Although, a number of studies have investigated the synergy between enzymes on LCB but degree of synergism has been sparingly determined.11 Moreover, synergy was assessed between a limited set of enzymes like; cellulases and xylanases or β-glucosidases or pectinases but laccases have been largely ignored.12 In this study, degree of synergism between cellulases and different accessory enzymes has been determined to improve the wheat straw hydrolysis at high solid loadings with minimum protein concentration.
2. Materials and methods
2.1 Enzyme preparations and chemicals
Celluclast 1.5L (cellulase from Trichoderma reesei ATCC 26921), Novozyme 188 (β-glucosidase from Aspergillus niger), xylanase (from Thermomyces lanuginosus), pectinase (from Aspergillus aculeatus) and laccase (from Trametes versicolor) were procured from Sigma Aldrich, India. CL, Accellerase, Sacchari-SEB-C6, Bioconvert L1 and Bioconvert P 10 (commercial enzyme mixtures) were either purchased or kindly provided as samples by Novozymes (Denmark), Genencor Dupont (USA), Advanced Enzyme (Mumbai, India), Noor Enzymes (West Bengal, India). All other standards and chemicals such as cellobiose, glucose, xylose, arabinose, furfural, hydroxy methyl furfural, acetic acid and BCA-1 kit were of analytical grade and procured from Sigma Aldrich (India).
2.2 Biomass material
Wheat straw (WS, Triticum aestivum) was used as the lignocellulosic substrate for enzyme and was procured from local market in Faridabad, Haryana, India. Faridabad (28.43° N 77.32° E) is located on the plains of the Yamuna river. It has a tropical climate with hot summers (up to 44 °C), and cold and foggy winters with temperature dipping to 5 °C. Wheat straw was air dried and grounded to 1–2 mm size using high speed cutting mill (Texol, Pune, India) and stored in sealed plastic bags at 30 °C.
2.3 Dilute acid pretreatment in pilot plant
A 250 kg per day continuous pilot-scale pretreatment reactor system was used for wheat straw (WS) pretreatment using dilute sulfuric acid. It includes a size reduction mill, high temperature and pressure reactor, flash tank, hydraulic press and a weight loss type feed hopper. The milled WS was presoaked in the acid solution for 30 minutes followed by pressing in a hydraulic press to remove excess liquid. The WS was fed to the feed hopper, which maintains the desired feed rate of 10 kg h−1. Material exits through a conveyor belt that delivers it to a plug mill that compresses the material into a strong solid plug that is then pushed into the pretreatment reactor. This unique arrangement helps to maintain the steam pressure in the reactor while continually injecting the feed into the reactor. After passing through the screw type pretreatment reactor, the pretreated material reaches to a flash tank. Pretreatment was carried out under previously optimized conditions (data not shown here) i.e. at 160 °C temperature, 10 min residence period and 0.5% (v/v) sulfuric acid. The pretreated biomass slurry (containing cellulose, hemicelluloses and lignin) was collected in the slurry tank. This was transferred through a pump to a high speed centrifuge for separating solids (mainly cellulose and lignin) and liquid (mainly pentoses).13
2.4 Analytical methods
Compositional analysis was carried out by following the Laboratory Analytical Procedure (LAP) of National Renewable Energy Laboratory (NREL)14 to determine the glucan, xylan, lignin, ash and extractives content in pretreated or untreated wheat straw. Various sugars (glucose, xylose, galactose, arabinose, mannose) and inhibitors (furfural, hydroxymethylfurfural (HMF), acetic acid, glycerol, levulinic acid, and formic acid) found in pretreatment slurry, were analyzed by high performance liquid chromatography (HPLC; Waters, Germany) equipped with a BioRAD AMINEX HPX-87H column (Biorad, Hercules, CA) at 50 °C, 0.008 N H2SO4 at a flow rate of 0.6 ml min−1 as mobile phase. Sugars were detected on a refractive index detector (RID) while inhibitors were detected on UV-detector. Oligomeric sugars in hydrolysate were analyzed by NREL LAP protocol.15,16 The oligomeric sugar concentration was determined by subtracting the monomeric sugar concentration of the non hydrolyzed sample from the total sugar concentration of acid hydrolysate after complete hydrolysis using 4% sulphuric acid. Total reducing sugar was determined by DNS method.17 All analyses were conducted in duplicate and average was calculated.
2.5 Enzyme assays and composition of enzyme preparations
β-Glucosidase activity (BGL) was determined as described previously by Agrawal, et al.18 One unit (U) of β-glucosidase was defined as the amount of the enzyme which would produce one μmol p-nitrophenol per min under the standard assay conditions and the specific activity was defined as the number of units per milligram (mg) of protein. Filter paper units (FPU) and endoglucanase (CMCase) activity were analyzed according to the method described by Ghose and Bisaria.19 Xylanase activity was determined as described by Gaur et al. (1992) using oat-spelt xylan as substrate.20 Pectinase assay was carried out by measuring reducing sugars release from pectin hydrolyzation. One unit of enzyme activity was defined as the amount of enzyme that catalyzes the release of 1 μmol of galacturonic acid per ml per minute under assay conditions.21 Laccase assay was carried out using 2,2′-azinobis(3-ethylbenzothiazoline-6-sulfonic acid) diammonium salt (ABTS) as the substrate.22 Protein concentrations of all enzyme preparations were estimated by bicinchoninic acid (BCA) method using BCA-1 kit (Sigma, USA).23
2.6 Enzymatic hydrolysis (supplementation and optimization)
After pretreatment, the solid residue was washed several times with distilled water to remove all soluble components (like free sugars and phenolics) and filtered to remove excess moisture. The washed pretreated wheat straw (PWS) was stored in sealed plastic bags at 4 °C (up to 2 weeks or at −18 °C if storage for a longer period) till further use. The PWS (5 g on dry weight basis) was taken in 500 ml Erlenmeyer flasks at 10% (w/w) solid loading, in a total reaction volume of 50 ml maintained by 0.05 M sodium citrate buffer (4.8 pH) and cellulase preparation (Celluclast 1.5L), either alone at different doses (10 to 125 mg protein per g biomass) or supplemented with various combinations of four different accessory enzyme preparations (Novozyme 188, xylanase, pectinase and laccase). Optimization of the enzyme mixture (Celluclast 1.5L and accessory enzyme), for achieving the maximum hydrolysis from PWS, was carried out by response surface methodology (RSM) using a factorial, central composite design (CCD) with replicates at the centre point and star points. The response value is the average of triplicates and statistical software package Design-Expert (Stat-Ease, Inc., Minneapolis, USA) was used for regression analysis of experimental data and to plot response surface.24
2.7 Enzymatic hydrolysis of PWS with different commercial enzymes
Hydrolysis of PWS was evaluated with different commercial enzyme preparations (Celluclast, CL, Accellerase, Sacchari-SEB-C6, Bioconvert L1 and Bioconvert P10) and in-house developed enzyme mixture (OptEMix) at same dosage (49 mg protein per g biomass) under identical conditions i.e. 10% biomass loading, 50 °C temperature and 4.8 pH maintained by 0.05 M citrate buffer.
3. Results and discussion
3.1 Pretreatment and chemical composition
The dilute sulfuric acid pretreatment hydrolyze hemicellulose into monomeric sugars (xylose arabinose, galactose, glucose, and mannose) and oligomers. A small amount of lignin is also depolymerized during acid pretreatment and it re-condensed and forms an altered lignin polymer.25 According to Kumar, et al.,24 with the removal of hemicellulose, surface area and pore volume of the substrate increases and it enhances the yield and rate of enzymatic hydrolysis. The pretreated WS slurry was centrifuged at 5000 rpm for 30 min to separate solid and liquid fractions. After centrifugation, most of the soluble monomeric and/or oligomeric sugars and inhibitors like furfural, hydroxyl-methyl furfurals and acetic acid remained in the liquid hydrolysate. The pretreated solids were washed thoroughly with distilled water to remove free sugars and inhibitors before further experiments. The chemical compositions of untreated wheat straw and PWS, as determined by NREL protocol, are shown in Fig. 1. The cellulose content after pretreatment increased from 36.6% to 69.8% and hemicellulose was hydrolyzed to 3.8%, resulting in to apparent increase in lignin content upon pretreatment from 22.2% to 26.4%. Saha, et al.26 have reported that untreated wheat straw contains 34.4% cellulose, 24.7% hemicellulose, 18.4% lignin, and 7.4% ash on dry basis. The high ash content found in this wheat straw sample may be due to silica present or adhered in the biomass. This type of extractable ash is usually present in the form of inorganic material that gets removed during dilute acid pretreatment process.
 |
| Fig. 1 Chemical composition of untreated and pretreated wheat straw. Others include water and ethanol extractives and protein content in WS. The values reported in graph are the average values of two independent experiments. | |
3.2 Enzyme activities
The activities of different enzyme preparations were determined and compared. All enzyme preparations demonstrated substantial differences in their protein content activities towards model substrates (Table 1). Celluclast contained quite high total cellulase activity (FPU) and endoglucanase activity (CMCase) in comparison to other accessory enzymes. The pectinase (from Aspergillus niger) and laccase (from Trametes versicolor) contained 3785 U ml−1 of pectinase and 83 U g−1 of laccase activity, respectively. Analysis indicates that accessory enzymes have low saccharification potential for cellulose.
Table 1 The activities of the different enzyme preparationsa
S. no. |
Enzymes |
Protein |
FPU |
CMCase |
BGL |
Xylanase |
The values reported (enzyme activities) here are the average values of two independent experiments. N.D. means not detected. |
1 |
Celluclast 1.5L |
52.08 (mg ml−1) |
99.60 (U ml−1) |
42.77 (U ml−1) |
11.52 (U ml−1) |
238.06 (U ml−1) |
2 |
Novozyme 188 (BGL) |
47.92 (mg ml−1) |
36.27 (U ml−1) |
22.79 (U ml−1) |
46.54 (U ml−1) |
255 (U ml−1) |
3 |
Xylanase (from Thermomyces lanuginosus) |
18.2 (mg g−1) |
2.93 (U g−1) |
1.06 (U g−1) |
2.21 (U g−1) |
685.23 (U g−1) |
4 |
Pectinase (from Aspergillus niger) |
7.52 (mg ml−1) |
4.72 (U ml−1) |
4.68 (U ml−1) |
32.14 (U ml−1) |
175.07 (U ml−1) |
5 |
Laccase (from Trametes versicolor) |
122 (mg g−1) |
12.13 (U g−1) |
0.82 (U g−1) |
N.D. |
13.27 (U g−1) |
3.3 Enzymatic hydrolysis with cellulase
Enzymatic hydrolysis of PWS was carried out by using Celluclast alone and after its supplementation with accessory enzymes. The contributions of sugars inherently present in all of these commercial enzymes have been adjusted while calculating the hydrolysis yield. Results indicate that PWS hydrolysis increased (Fig. 2) with increasing the Celluclast dosage (5 to 125 mg protein per g biomass). As approximately 60% of hydrolysis was achieved by Celluclast at a dosage of 10 mg protein per g biomass after 24 and 48 h respectively; therefore, this Celluclast dosage was selected for further supplementation studies with four accessory enzymes (Novozyme 188, xylanase, pectinase and laccase). Pierre, et al.27 have found that hydrolysis of thermo-mechanically (mechanical pretreatment followed by steam explosion pretreatment in a specially designed reactor) pretreated wheat straw was increased from 35% to 91% with increasing Celluclast dosage (0.38 mg to 9 mg g−1 biomass). Hu, et al.28 have also reported that cellulose hydrolysis increased with increasing cellulase loading and reached to a stationary phase at Celluclast loading of 35 mg g−1 cellulose with 70% hydrolysis of steam pretreated corn stover. A further increase in cellulase loading beyond 35 mg g−1 cellulose resulted in only marginally improved hydrolysis yields.
 |
| Fig. 2 Enzymatic hydrolysis of dilute acid pretreated wheat straw with Celluclast at different doses (5 mg to 125 mg protein per g biomass). The values reported in graph are the average values of two independent experiments. | |
3.4 Supplementation of cellulase with accessory enzymes
Hemicellulose, pectin and lignin act as physical barrier for enzymatic accessibility of cellulose and enzymes bind non-specifically with lignin.29 Moreover, cellulases from T. reesei are naturally deficient in β-glucosidase. Hence, supplementation of cellulases with these accessory enzymes (xylanases, β-glucosidase, pectinases and laccases) was taken into account for developing the enzyme cocktail to maximize the hydrolysis efficiency.
The supplementation of Celluclast with accessory enzyme was carried out to decipher the saturation limits of each accessory enzyme so that critical dosage of each enzyme could be determined. Supplementation of Celluclast with 20 mg protein per g biomass of Novozyme 188 enhanced the biomass hydrolysis by 20–30% after 6 and 24 h, respectively (Fig. 3a and b). While, xylanase supplementation low (1 mg protein per g biomass) level improved the biomass hydrolysis by 50–70% after 6 and 24 h, respectively (Fig. 3c and d). Similarly, Celluclast supplementation with pectinase and laccase improved hydrolysis approximately by 50%. Hu, et al.28 and Li, et al.30 have also found that hydrolysis yield was increased by approx. 16% and 30.5% with xylanase supplementation using steam pretreated corn stover and steam pretreated sugarcane baggase, respectively. Gonçalves, et al.31 reported that about 2 fold increase in pretreated bagasse hydrolysis was observed when cellulase was supplemented with xylanase. Kumar and Wyman32 reported that reason for improved hydrolysis with supplementation of xylanases is not only the residual xylose and cellulose–xylan interactions but other effects may be involved, such as the presence of acetyl groups on the xylan which will reduce the efficiency of the depolymerising endo-xylanase. Berlin, et al.33 have reported that hydrolysis of dilute acid pretreated corn stover was improved by approx. 37% by supplementing Celluclast with Novozyme 188. Recently, hydrolysis of date palm lignocellulosic waste was improved to 60% by synergistic effect of cellulase, xylanase and laccase in comparison of cellulase alone.34 After determining the saturation limits for each of the four enzyme supplements, optimization of the enzyme mixture was performed by response surface methodology.
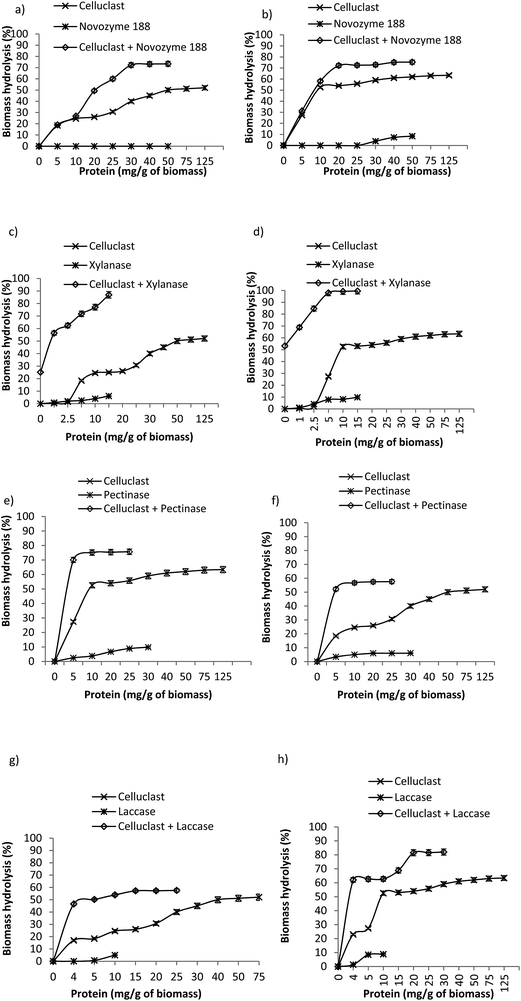 |
| Fig. 3 Supplementation of Celluclast with Novozyme 188 (a and b), xylanase (c and d), pectinase (e and f) and laccase (g and h). The corresponding hydrolysis time for (a), (c), (e) and (g) is 6 h and for (b), (d), (f) and (h) is 24 h, respectively. The values reported in graphs are the average values of two independent experiments. | |
3.5 Synergism between cellulase and accessory enzymes
LCB has a complex structure, where cellulose, hemicellulose and lignin are closely interlinked with each other. The variation in structure between different biomasses and the effect of different pretreatments further increase its complexity. Therefore, multiple enzymes are required to work together synergistically for complete hydrolysis of LCB. Synergism means that cooperation of different types of enzymes enhances the product yield.35 For supplementation system, degree of synergism (DS) may be determined as the ratio of the product yield released by the enzyme cocktail to the sum of the product yield released by the individual enzymes when used separately in the same amounts as in the mixture.30 Thus, DS was calculated as the ratio of the maximum hydrolysis yield achieved (product yield) at the optimal concentrations (protein content in mg) of different enzymes in combinations and the hydrolysis yield achieved at exactly the same amounts of enzymes when used individually/separately. The relationship between DS and hydrolysis time was determined after supplementation of Celluclast with accessory enzymes. Celluclast exhibited highest degree of synergism with xylanase (2.97) followed by pectinase (2.18), laccase (2.07), and Novozyme 188 (1.6) (Fig. 4). The numbers in parentheses indicate the degree of synergism between Celluclast and the selected enzyme quantitatively. The value of DS is directly proportional to synergism between enzymes. Li et al.,30 have found that the DS for Celluclast and xylanase are in the range of 1.05 to 1.19 with steam exploded sugarcane bagasse.
 |
| Fig. 4 Degree of synergism between Celluclast, Novozyme 188, xylanase, pectinase and laccase. The values reported in graph are the average values of two independent experiments. | |
3.6 Optimization of the enzyme mixture
The experimental design and results of 30 experiments for the enzyme mixture optimization are tabulated in Table 2. The responses of the CCD design were fitted with a second order polynomial equation (eqn (1) and (2)). It describes the correlation between the significant variables and the response (hydrolysis in 6 h and 24 h) in terms of coded value when using the model. The overall second-order polynomial equation, in terms of coded factors, can be written as follows: |
Hydrolysis (6 h) = 75.32 + 2.07*A + 7.72*B + 0.18*C + 0.31*D − 0.17*AB + 0.63*AC + 0.44*AD − 0.10*BC − 0.48*BD + 0.72*CD − 0.37*A2 + 0.41*B2 + 0.26*C2 + 0.14*D2
| (1) |
|
Hydrolysis (24 h) = 83.82 + 1.61*A + 7.96*B − 0.68*C − 0.93*D
| (2) |
Table 2 Experimental set up based on central composite design for PWS hydrolysis
Run |
Novozyme 188 (mg g−1) |
Xylanase (mg g−1) |
Pectinase (mg g−1) |
Laccase (mg g−1) |
Hydrolysis after 6 h (%) |
Hydrolysis after 24 h (%) |
1 |
20 |
3 |
5.5 |
20 |
76.52 |
81.36 |
2 |
25 |
4 |
3.25 |
16 |
85.45 |
94.7 |
3 |
20 |
3 |
5.5 |
12 |
72.32 |
83.35 |
4 |
25 |
4 |
7.75 |
16 |
85.34 |
96.48 |
5 |
15 |
2 |
3.25 |
8 |
66.92 |
74.67 |
6 |
10 |
3 |
5.5 |
12 |
69.8 |
80.12 |
7 |
25 |
4 |
3.25 |
8 |
86.25 |
97.35 |
8 |
25 |
2 |
7.75 |
16 |
76.11 |
63.64 |
9 |
25 |
2 |
7.75 |
8 |
66.41 |
79.61 |
10 |
20 |
3 |
5.5 |
12 |
74.92 |
86.43 |
11 |
20 |
5 |
5.5 |
12 |
90.13 |
98.8 |
12 |
25 |
2 |
3.25 |
16 |
67.33 |
79.58 |
13 |
20 |
1 |
5.5 |
12 |
63.91 |
73.25 |
14 |
20 |
3 |
5.5 |
12 |
75.15 |
82.57 |
15 |
20 |
3 |
1 |
12 |
76.19 |
87.34 |
16 |
15 |
2 |
7.75 |
8 |
64.31 |
73.51 |
17 |
20 |
3 |
5.5 |
4 |
75.4 |
85.61 |
18 |
15 |
4 |
7.75 |
8 |
82.14 |
90.47 |
19 |
25 |
4 |
7.75 |
8 |
86.71 |
95.45 |
20 |
15 |
4 |
3.25 |
16 |
82.29 |
87.83 |
21 |
25 |
2 |
3.25 |
8 |
68.8 |
75.61 |
22 |
20 |
3 |
5.5 |
12 |
77.221 |
84.17 |
23 |
15 |
4 |
3.25 |
8 |
81.63 |
88.72 |
24 |
20 |
3 |
5.5 |
12 |
75.67 |
80.19 |
25 |
15 |
4 |
7.75 |
16 |
82.35 |
87.43 |
26 |
15 |
2 |
3.25 |
16 |
65.33 |
76.38 |
27 |
20 |
3 |
5.5 |
12 |
76.63 |
83.49 |
28 |
20 |
3 |
10 |
12 |
76.64 |
85.61 |
29 |
30 |
3 |
5.5 |
12 |
78 |
85.48 |
30 |
15 |
2 |
7.75 |
16 |
64.08 |
75.43 |
The Prob > F value for the model was <0.0001, which indicated that the model was statistically significant. ANOVA was performed for the evaluation of the effects of the variables and their possible interactions for hydrolysis after 6 h (Table 3a) and 24 h (Table 3b).
Table 3 (a) ANOVA for response surface model for hydrolysis in 6 h, (b) ANOVA for response surface model for hydrolysis in 24 h
(a) |
Source |
Sum of squares |
Degree of freedom |
F value |
P-value (Prob > F) |
Model |
1570.85 |
14 |
23.32 |
<0.0001 |
A-Novozyme 188 |
103.13 |
1 |
21.44 |
0.0003 |
B-xylanase |
1430.82 |
1 |
297.41 |
<0.0001 |
C-pectinase |
0.79 |
1 |
0.16 |
0.6913 |
D-laccase |
2.25 |
1 |
0.47 |
0.5044 |
AB |
0.45 |
1 |
0.093 |
0.7651 |
AC |
6.29 |
1 |
1.31 |
0.2709 |
AD |
3.07 |
1 |
0.64 |
0.4368 |
BC |
0.16 |
1 |
0.034 |
0.8569 |
BD |
3.72 |
1 |
0.77 |
0.3934 |
CD |
8.28 |
1 |
1.72 |
0.2093 |
A2 |
3.81 |
1 |
0.79 |
0.3876 |
B2 |
4.55 |
1 |
0.95 |
0.3461 |
C2 |
1.80 |
1 |
0.37 |
0.5500 |
D2 |
0.56 |
1 |
0.12 |
0.7386 |
Residual |
72.16 |
15 |
|
|
Lack of fit |
57.52 |
10 |
1.96 |
0.2362 |
Pure error |
14.64 |
5 |
|
|
Cor total |
1643.02 |
29 |
|
|
R2 = 0.9561; adjusted R2 = 0.9151 |
(b) |
Source |
Sum of squares |
Degree of freedom |
F value |
P-value (Prob > F) |
Model |
1616.02 |
4 |
33.24 |
<0.0001 |
A-Novoyme 188 |
62.40 |
1 |
5.14 |
0.0324 |
B-xylanase |
1521.63 |
1 |
125.21 |
<0.0001 |
C-pectinase |
11.04 |
1 |
0.91 |
0.3496 |
D-laccase |
20.94 |
1 |
1.72 |
0.2012 |
Residual |
303.82 |
25 |
|
|
Lack of fit |
283.04 |
20 |
3.41 |
0.0891 |
Pure error |
20.77 |
5 |
|
|
Cor total |
1919.84 |
29 |
|
|
R2 = 0.8417; adjusted R2 = 0.8164 |
Values of “Prob > F” less than 0.0500 indicate model terms are significant. In both the cases (hydrolysis after 6 h and 24 h) A and B are significant model terms. The “Pred R-Squared” values of 6 h (0.7855) and 24 h (0.7584) is in reasonable agreement with the “Adj R-Squared” values of 0.9151 and 0.8164, respectively. “Adeq Precision” measures the signal to noise ratio. A ratio greater than 4 is desirable. Our ratio of 19.914 and 22.380 after 6 h and 24 h, respectively indicates an adequate signal. Therefore, both of these models can be used to navigate the design space (Table 3a and b).
Based on the model prediction and experimental validation, the optimized enzyme mixture containing following dosages (protein per g of biomass) of Celluclast (10 mg), Novozyme 188 (25 mg), xylanase (4 mg), pectinase (3.25 mg) and laccase (8 mg) were evaluated for the saccharification of PWS. The results showed that by partially replacing Celluclast proportion with accessory enzymes enhanced the PWS hydrolysis (>95% hydrolysis), at low protein loadings (Fig. 2 and Table 2). However, even with high dosage of Celluclast (>100 mg protein per g biomass) only a maximum of 70% of PWS hydrolysis was obtained. Qing and Wyman6 reported that supplementation of cellulase (Spezyme) at the dosage of 16 mg protein per g biomass with double quantity of Novozyme 188 (32 mg protein per g biomass) enhanced the PWS hydrolysis from 50% to 80%. Moreover, addition of xylanase enhanced the hydrolysis yields by 13% in comparison with just cellulase and Novozyme 188.
The cellobiose (1.93%) and oligomer (0.48%) concentration was found to be quite high with Celluclast alone in comparison to OptEMix after 6 h (Fig. 5). These residual sugars were either completely removed or present in minute quantity with OptEMix (Fig. 5). The oligomers and cellobiose have been reported to act as inhibitors for enzyme causing feedback inhibition. Qing, et al.9 found that even low concentration of oligomers like 0.16–1.25% causes 15–38% reduction in hydrolysis yield.
 |
| Fig. 5 Enzymatic hydrolysis of dilute acid pretreated wheat straw with Celluclast and OptEMix. The values reported in graph are the average values of two independent experiments. | |
3.7 Assessment of enzyme activities after supplementation
In order to find out the effect of synergism on enzyme activities and the reason for increased hydrolysis, different enzyme combinations prepared by supplementing Celluclast (at a dosage of 10 mg protein per g biomass) with accessory enzymes were evaluated for FPU, CMCase, BGL and xylanase activities and compared with the sum of individual enzymes activities (Table 4). The analysis showed that all enzyme activities (FPU, CMCase, BGL and xylanase) were more than the sum of activities of individual enzymes at selected dosage. This confirms that increase in enzyme activities is not an additive effect but synergistic effect. The maximum synergism was observed in OptEmix where, FPU, CMCase, BGL and xylanase were enhanced by 2.85, 4.0, 12.01 and 5.13 folds, respectively in comparison to the sum of the enzyme quantities actually added. Recently, Adsul, et al.13 have reported that FPU and CMCase activities were enhanced by 1.3 and 2 times, the sum of their individual activities added in the mixture, respectively. The synergism was much higher in case of enzyme mixture containing three enzymes in comparison of two enzymes from different sources.
Table 4 Enzyme activity analysis in different Celluclast supplements
S. no. |
Enzymes |
FPU |
CMCase |
BGL |
Xylanase |
The synergistic values as found in reaction mixture (rxn) are reported as U ml−1 of rxn while, the theoretical additive values corresponding to the protein are depicted within brackets. The values (enzyme activities) reported here are the average values of two independent experiments. |
1 |
Celluclast (10 mg) + Novozyme 188 (25 mg)a |
6.21 (3.77) (U ml−1 of rxn) |
2.86 (1.99) (U ml−1 of rxn) |
3.56 (2.64) (U ml−1 of rxn) |
23.00 (17.79) (U ml−1 of rxn) |
2 |
Celluclast (10 mg) + Novozyme 188 (50 mg)a |
7.70 (5.66) (U ml−1 of rxn) |
4.29 (3.18) (U ml−1 of rxn) |
6.69 (5.06) (U ml−1 of rxn) |
50.00 (31.07) (U ml−1 of rxn) |
3 |
Celluclast (10 mg) + xylanase (10 mg)a |
3.71 (0.14) (U ml−1 of rxn) |
2.16 (0.05) (U ml−1 of rxn) |
0.66 (0.32) (U ml−1 of rxn) |
45.66 (37.41) (U ml−1 of rxn) |
4 |
Celluclast (10 mg) + xylanase (30 mg)a |
3.94 (2.31) (U ml−1 of rxn) |
2.31 (0.97) (U ml−1 of rxn) |
0.84 (0.54) (U ml−1 of rxn) |
119.62 (103.20) (U ml−1 of rxn) |
5 |
Celluclast (10 mg) + pectinase (20 mg)a |
3.57 (3.22) (U ml−1 of rxn) |
2.87 (2.13) (U ml−1 of rxn) |
10.21 (9.28) (U ml−1 of rxn) |
153.10 (121.24) (U ml−1 of rxn) |
6 |
Celluclast (10 mg) + pectinase (30 mg)a |
4.35 (3.89) (U ml−1 of rxn) |
2.95 (2.79) (U ml−1 of rxn) |
15.96 (13.81) (U ml−1 of rxn) |
247.64 (108.67) (U ml−1 of rxn) |
7 |
Celluclast (10 mg) + laccase (10 mg)a |
2.37 (2.00) (U ml−1 of rxn) |
2.09 (0.82) (U ml−1 of rxn) |
0.31 (0.22) (U ml−1 of rxn) |
61.65 (8.95) (U ml−1 of rxn) |
8 |
Celluclast (10 mg) + laccase (20 mg)a |
2.60 (2.11) (U ml−1 of rxn) |
2.05 (0.83) (U ml−1 of rxn) |
0.56 (0.22) (U ml−1 of rxn) |
83.48 (13.37) (U ml−1 of rxn) |
9 |
OptEmixa |
6.41 (2.25) (U ml−1 of rxn) |
4.26 (1.05) (U ml−1 of rxn) |
49.87 (4.15) (U ml−1 of rxn) |
206.34 (40.19) (U ml−1 of rxn) |
3.8 Enzymatic hydrolysis of PWS with different commercial enzymes
In order to compare the hydrolytic potential of OptEMix with other commercial enzymes on a common feedstock under identical conditions, all enzymes were evaluated for PWS hydrolysis at same protein loading (54.75 mg protein per g biomass) as in OptEMix (Fig. 6). Results showed that maximum hydrolysis yield (>95%) was achieved with OptEMix and CL enzyme. A substantial difference in hydrolysis was found between OptEMix and its parent enzyme/Celluclast and at same dosage. Alvira, et al.36 have found that approx. 91% hydrolysis yield achieved after 72 h with 36 mg protein per g biomass of Accellerase using steam exploded wheat straw. Cannella and Jørgensen37 have reported that Cellic enzyme at a dosage of 10 mg protein per g biomass resulted in to about 85% hydrolysis with steam exploded wheat straw. Pierre, et al.27 achieved 91% hydrolysis of thermo-mechanically pretreated wheat straw with Celluclast in a specially designed reactor. Singhania, et al.38 found that about 51% hydrolysis of dilute acid pretreated wheat straw was achieved with 7.34 mg protein per g of biomass using Sacchari-SEB-C6 enzyme. Recently, Agrawal, et al.39 have reported that 90% PWS hydrolysis was obtained with CL enzyme after 48 h at 30 mg protein per g biomass.
 |
| Fig. 6 Enzymatic hydrolysis of dilute acid pretreated wheat straw with different commercial cellulase preparations. All enzymes were evaluated at same protein loading (50.25 mg protein per g biomass). The values reported in graph are the average values of two independent experiments. | |
4. Conclusions
It is apparent from this study that the overall enzyme loading required to achieve fast, nearly complete hydrolysis of dilute acid pretreated wheat straw could be substantially reduced by making use of the synergistic interaction between cellulases and accessory enzymes. It is likely that the accessory enzymes helped in solubilizing/removing of oligomers which subsequently reduce the cellulase inhibition. Thus, development of enzyme cocktail offers considerable potential to improve pretreated biomass hydrolysis.
Acknowledgements
The samples of enzymes provided by all reputed companies are gratefully acknowledged. Authors are thankful to IOCL R&D for providing all infrastructural, analytical and financial assistance. All support from DBT, Govt. of India is duly acknowledged.
References
- J. K. Saini, R. Agrawal, A. Satlewal, R. Saini, M. Anshu, R. P. Gupta and D. Tuli, RSC Adv., 2015, 5, 37485–37494 RSC.
- R. Biswas, H. Uellendahl and B. Ahring, BioEnergy Res., 2015, 1–16, DOI:10.1007/s12155-015-9590-5.
- R. Agrawal, A. Satlewal and A. Verma, 3 Biotech, 2013, 3, 381–388 CrossRef.
- M. Selig, E. Knoshaug, W. Adney, M. Himmel and S. Decker, Bioresour. Technol., 2008, 99, 4997–5005 CrossRef CAS PubMed.
- Z. Xiao, X. Zhang, D. Gregg and J. Saddler, Appl. Biochem. Biotechnol., 2004, 113, 1115–1126 CrossRef PubMed.
- Q. Qing and C. Wyman, Biotechnol. Biofuels, 2011, 4, 18–30 CrossRef CAS PubMed.
- M. A. Kabel, G. Bos, J. Zeevalking, A. G. Voragen and H. A. Schols, Bioresour. Technol., 2007, 98, 2034–2042 CrossRef CAS PubMed.
- L. Kumar, V. Arantes, R. Chandra and J. Saddler, Bioresour. Technol., 2012, 103, 201–208 CrossRef CAS PubMed.
- Q. Qing, B. Yang and C. Wyman, Bioresour. Technol., 2010, 101, 9624–9630 CrossRef CAS PubMed.
- J. D. McMillan, E. W. Jennings, A. Mohagheghi and M. Zuccarello, Biotechnol. Biofuels, 2011, 4, 29–46 CrossRef CAS PubMed.
- J. S. van Dyk and B. I. Pletschke, Biotechnol. Adv., 2012, 30, 1458–1480 CrossRef CAS PubMed.
- L. Ji, J. Yang, H. Fan, Y. Yang, B. Li, X. Yu, N. Zhu and H. Yuan, Biotechnol. Biofuels, 2014, 7, 130 CrossRef PubMed.
- M. Adsul, B. Sharma, R. R. Singhania, J. K. Saini, A. Sharma, A. Mathur, R. Gupta and D. K. Tuli, RSC Adv., 2014, 4, 44726–44732 RSC.
- A. Sluiter, B. Hames, R. Ruiz, C. Scarlata, J. Sluiter, D. Templeton and D. Crocker, Technique report, NREL/TP-510-42618, 2008 Search PubMed.
- A. Sluiter, B. Hames, R. Ruiz, C. Scarlata, J. Sluiter and D. Templeton, Determination of Sugars, Byproducts, and Degradation Products in Liquid Fraction Process Samples, Laboratory Analytical Procedure (LAP), Technical Report NREL/TP-510-42623, National Renewable Energy Laboratory, Colorado, 2008.
- A. Sluiter, B. Hames, R. Ruiz, C. Scarlata, J. Sluiter, D. Templeton and D. Crocker, Determination of Structural Carbohydrates and Lignin in Biomass, Laboratory Analytical Procedure (LAP), Technical Report NREL/TP-510-42618, National Renewable Energy Laboratory, Colorado, 2011.
- G. L. Miller, Anal. Chem., 1959, 31, 426–428 CrossRef CAS.
- R. Agrawal, A. Satlewal, A. S. Mathur, R. P. Gupta, T. Raj, R. Kumar and D. K. Tuli, Environ. Eng. Manage. J., 2014 Search PubMed , http://omicron.ch.tuiasi.ro/EEMJ/pdfs/accepted/468_453_Agrawal_14.pdf, in press.
- T. Ghose and V. Bisaria, Biotechnol. Bioeng., 1979, 21, 131–146 CrossRef CAS PubMed.
- R. Gaur, R. Lata and S. K. Khare, World J. Microbiol. Biotechnol., 2005, 21, 1123–1128 CrossRef CAS.
- D. Ibrahim, H. Weloosamy and L. Sheh-Hong, Process Biochem., 2014, 49, 660–667 CrossRef CAS.
- S. Zhao, C. B. Rong, C. Kong, Y. Liu, F. Xu, Q. J. Miao, S. X. Wang, H. X. Wang and G. Q. Zhang, BioMed Res. Int., 2014, 2014, 417–461 Search PubMed.
- J. M. Walker, Methods Mol. Biol., 1994, 32, 5–8 CAS.
- R. Kumar, A. Satlewal, S. Sharma, V. Kagdiyal, R. P. Gupta, D. K. Tuli and R. K. Malhotra, J. Renewable Sustainable Energy, 2014, 6, 0331181–03311811 Search PubMed.
- B. S. Donohoe, S. R. Decker, M. P. Tucker, M. E. Himmel and T. B. Vinzant, Biotechnol. Bioeng., 2008, 101, 913–925 CrossRef CAS PubMed.
- B. C. Saha, N. N. Nichols, N. Qureshi, G. J. Kennedy, L. B. Iten and M. A. Cotta, Bioresour. Technol., 2015, 175, 17–22 CrossRef CAS PubMed.
- G. Pierre, Z. Maache-Rezzoug, F. Sannier, S.-A. Rezzoug and T. Maugard, Process Biochem., 2011, 46, 2194–2200 CrossRef CAS.
- J. Hu, V. Arantes and J. N. Saddler, Biotechnol. Biofuels, 2011, 4, 36–49 CrossRef CAS PubMed.
- J. Zhang, A. Pakarinen and L. Viikari, Bioresour. Technol., 2013, 129, 302–307 CrossRef CAS PubMed.
- J. Li, P. Zhou, H. Liu, C. Xiong, J. Lin, W. Xiao, Y. Gong and Z. Liu, Bioresour. Technol., 2014, 155, 258–265 CrossRef CAS PubMed.
- G. A. L. Gonçalves, Y. Takasugi, L. Jia, Y. Mori, S. Noda, T. Tanaka, H. Ichinose and N. Kamiya, Enzyme Microb. Technol., 2015, 72, 16–24 CrossRef PubMed.
- R. Kumar and C. E. Wyman, Bioresour. Technol., 2009, 100, 4203–4213 CrossRef CAS PubMed.
- A. Berlin, V. Maximenko, N. Gilkes and J. Saddler, Biotechnol. Bioeng., 2007, 97, 287–296 CrossRef CAS PubMed.
- S. Al-Zuhair, K. Ahmed, A. Abdulrazak and M. H. El-Naas, J. Ind. Eng. Chem., 2013, 19, 413–415 CrossRef CAS.
- M. Kostylev and D. Wilson, Biofuels, 2012, 3, 61–70 CrossRef CAS.
- P. Alvira, M. J. Negro, F. Sáez and M. Ballesteros, J. Chem. Technol. Biotechnol., 2010, 85, 1291–1297 CrossRef CAS.
- D. Cannella and H. Jørgensen, Biotechnol. Bioeng., 2014, 111, 59–68 CrossRef CAS PubMed.
- R. R. Singhania, J. K. Saini, R. Saini, M. Adsul, A. Mathur, R. Gupta and D. K. Tuli, Bioresour. Technol., 2014, 169, 490–495 CrossRef CAS PubMed.
- R. Agrawal, A. Satlewal, R. Gaur, A. Mathur, R. Kumar, R. P. Gupta and D. K. Tuli, Biochem. Eng. J., 2015, 102, 54–61 CrossRef CAS.
|
This journal is © The Royal Society of Chemistry 2015 |