DOI:
10.1039/C5RA12951F
(Paper)
RSC Adv., 2015,
5, 67979-67987
Luminescence and energy transfer of co-doped Sr5MgLa2(BO3)6:Ce3+,Mn2+
Received
3rd July 2015
, Accepted 28th July 2015
First published on 12th August 2015
Abstract
In this work the photoluminescence (PL) of co-doped Sr5MgLa2(BO3)6:Ce3+,Mn2+ as well as the energy transfer (ET) from Ce3+ to Mn2+ was examined. To this end, powder samples with different Mn2+ concentrations were synthesised via high temperature solid state reaction. Phase purity of prepared samples was investigated by X-ray powder diffraction. To elucidate the PL properties of Sr5MgLa2(BO3)6:Ce3+,Mn2+, emission and excitation spectra along with diffuse reflectance spectra were recorded. Additionally, fluorescence lifetime measurements were performed. To study the temperature behaviour of the emission of Sr5MgLa2(BO3)6:Ce3+,Mn2+, PL and lifetime measurements were conducted from 100 to 500 K. Furthermore, external quantum efficiencies were determined and chromaticity coordinates were calculated. It was found that co-doped Sr5MgLa2(BO3)6:Ce3+,Mn2+ possesses two emission bands located in the violet/blue and deep red range of the electromagnetic spectrum. Moreover, it turned out that ET from Ce3+ to Mn2+ in Sr5MgLa2(BO3)6:Ce3+,Mn2+ takes place via an exchange interaction mechanism. Temperature dependent PLD measurements reveal that thermal quenching of emission is mainly caused by the Mn2+ ions. Finally, quantum efficiency of Ce3+ doped Sr5MgLa2(BO3)6:Ce3+ was found to be close to unity.
1. Introduction
In the last two decades light emitting diodes (LEDs) have more and more replaced conventional light sources like incandescent or fluorescent lamps. This is due to the benefits LEDs provide to lighting installations over traditional light sources. Commonly, LEDs provide longer lifetime, higher wall plug efficiency, as well as a higher colour rendering.1 Nowadays most of the white emitting LEDs consist of a blue emitting (In,Ga)N-chip combined with a green-yellow emitting phosphor, e.g. Y3Al5O12:Ce3+.2 These light sources provide high luminous efficiency due to intense emission in the green spectral range. On the other site, due to the lack of red emission these systems suffer from a high colour temperature and low colour rendering index (CRI) which is unintended for domestic lighting.3 On that account an alternative approach to generate warm white light with appropriate CRI is the combination of a blue, green, and red phosphor excited by an ultraviolet (UV) emitting LED. In this way LEDs with excellent colour temperature as well as convenient CRI can be designed.4 Unfortunately, these packages incur a loss in blue emission due to reabsorption by the green and red phosphors. This is why many groups try to develop single phase white emitting phosphors which convert UV radiation into white light.5 One strategy to realize such a phosphor is to use the ion couple Ce3+ and Mn2+.6 In many host materials Ce3+ and Mn2+ possess broad emission bands in the blue and red spectral range, respectively.7 Due to additive colour mixing these emission bands can complement each other to white light. Further, the broad excitation band of the spin and parity allowed [Xe]4f1–[Xe]5d1 transition in Ce3+ is suitable for pumping with UV LEDs. Since all transitions in Mn2+ are fully forbidden, the blue emission of Ce3+ can also be used for sensitizing the [Ar]3d5–[Ar]3d5 excitation transition via energy transfer (ET).
To investigate the photoluminescence (PL) properties as well as the ET from Ce3+ to Mn2+ in co-activated Sr5MgLa2(BO3)6:Ce3+,Mn2+ a series of powder samples with various Mn2+ contents was prepared. Sr5MgLa2(BO3)6 belongs to a class of ortho-borates, firstly described by Schaffers et al. in 1994.8 Further, Sankar and Rao reported on the PL of different compositions of said material with numerous activators.9,10 Sr5MgLa2(BO3)6 crystalizes in a high-symmetry trigonal structure with space group R
. Sr5MgLa2(BO3)6 comprises a 9-fold coordinated site as well as two different octahedrally coordinated sites. The 9-fold coordinated sites are shared by Sr2+ and La3+ ions in the ratio 0.67 to 0.33. The octahedrally coordinated sites differ in average bond length of the surrounding oxygen ions. The larger sites exhibit an average bond length of 0.2298 Å and are occupied by Sr2+ ions whereas the smaller octahedral sites with a mean distance of 0.2092 Å are occupied by Mg2+ ions. The ionic radii of La3+ and Sr2+ for 9-fold coordination are 1.216 and 1.13 Å. Sr2+ and Mg2+ exhibit ionic radii of 1.18 and 0.72 Å in an octahedral coordination. The ionic radii of Ce3+ for 9-fold and 6-fold coordination are 1.01 and 1.196 Å. 6-fold coordinated Mn2+ has an ionic radius of 0.83 Å.11 Considering the ionic radii as well as charge balancing, it is assumed that the Ce3+ ions primarily going to occupy the 9-fold coordinated La3+ sites whereas Mn2+ ions tend to occupy the octahedral Mg2+ sites.
In this contribution, synthesis, optical properties, and ET from Ce3+ to Mn2+ in co-doped Sr5MgLa2(BO3)6:Ce3+,Mn2+ are discussed. Therefore, samples with different doping concentrations were prepared via high temperature solid state synthesis. Phase purity was validated using X-ray powder diffractometry (XRD). Diffuse reflectance (DR) spectra were recorded to examine the body colour of Sr5MgLa2(BO3)6:Ce3+,Mn2+. PL properties were investigated by recording PL spectra and photoluminescence excitation spectra (PLE). Temperature behaviour of the PL was examined by recording PL spectra from 100 to 500 K. In addition, photoluminescence decay (PLD) measurements were performed from 100 to 500 K to study the origin of thermal quenching. To monitor the ET from Ce3+ to Mn2+ PLD measurements were performed on samples with different Mn2+ concentrations. Furthermore, external quantum efficiencies ηext were determined and chromaticity coordinates according to Commission International de l'Eclairage 1931 (CIE) were calculated.
2. Experimental
All investigated Sr5MgLa2(BO3)6:Ce3+,Mn2+ samples were prepared by high temperature solid state synthesis. To this end, high purity educts SrCO3 (Aldrich, 99.9%), MgO (Merck KGaA, p.a.), La2O3 (Treibacher Industrie AG, 99.995%), H3BO3 (Merck KGaA, Ph. Eur.), CeO2 (Atomergic Chemetals Co., 99.9%), and MnC2O4·2H2O (Dr Paul Lohmann, chem. pure) were weighted in stoichiometric amounts. Though, an excess of 5% of boric acid was used to compensate evaporation. The starting materials were thoroughly blended in acetone in an agate mortar. After drying at ambient temperatures, the obtained powder blends were heated at 650 °C for 1 h in air to decompose the boric acid. Afterwards, the samples were ground and calcined at 1200 °C for 6 h in a corundum crucible imbedded in active carbon. Obtained white sinter bodies were subsequently ground to a fine μ-powder.
Phase purity of the synthesized Sr5MgLa2(BO3)6:Ce3+,Mn2+ samples was investigated using XRD. XRD patterns were collected on a Rigaku MiniFlex II diffractometer working in Bragg–Brentano geometry using Cu Kα radiation. Step width and integration time were set to 0.02° and 1 s, respectively.
PL spectra as well as PLE spectra were recorded on an Edinburgh Instruments FSL900 spectrometer equipped with a Xe arc lamp (450 W) and a cooled (−20 °C) single-photon counting photomultiplier (Hamamatsu R2658P). Obtained PL spectra were corrected by applying a correction file obtained from a tungsten incandescent lamp certified by the National Physics Laboratory U.K.
For PLD measurements on Ce3+, a picosecond pulsed LED (40 μW, pulse width = 912.6 ps, λem = 267 nm) was attached to the spectrometer. For PLD measurements on Mn2+ a microsecond pulsed Xe lamp (100 W, pulse width = 1 μs) was used.
PL measurements at 2.9 K were performed using the closed cycle cryocooler OptistatAC-V12 from Oxford Instruments. Helium was used as a cooling agent. Temperature dependent PL measurements from 100 to 500 K were performed using the Oxford Instruments cryostat MicrostatN2. Liquid nitrogen was used as a cooling agent. Temperature stabilization time was 60 s and tolerance was set to ±3 K.
DR spectra were recorded on an Edinburgh Instruments FS900 spectrometer equipped with a Xe arc lamp (450 W), a cooled (−20 °C) single-photon counting photomultiplier (Hamamatsu R928) as well as a Teflon-coated integration sphere. BaSO4 (99.998%, Sigma-Aldrich) was used as a reflectance standard.
External quantum efficiencies ηext were determined using the approach of Kawamura et al.12 Therefore, PL spectra of the samples as well as of the excitation source were recorded in a Teflon-coated integration sphere. From this, ηext can be calculated using the following relation:
|
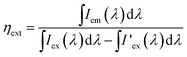 | (1) |
here,
Iem(
λ) is the emission intensity of the sample,
Iex(
λ) is the intensity of the excitation lamp in absence of the sample and
I'
ex(
λ) is the intensity of the excitation lamp in presence of the sample.
3. Results and discussion
To investigate phase purity of the synthesised Sr5MgLa2(BO3)6 samples XRD patterns were recorded. Fig. 1 shows the collected XRD patterns of doped and undoped Sr5MgLa2(BO3)6 as well as the ICDD reference card of Sr5MgLa2(BO3)6. The diffractograms indicate the formation of the trigonal Sr5MgLa2(BO3)6 phase. No change in the host structure can be observed with increasing doping concentration. This observation confirms the suitability of the utilized synthesis route.
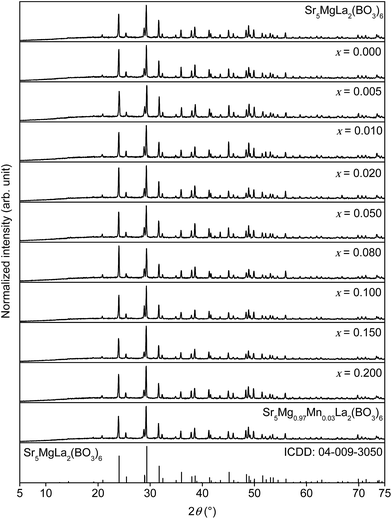 |
| Fig. 1 XRD patterns of Sr5MgLa2(BO3)6, Sr5Mg1−xMnx(La0.99Ce0.01)2(BO3)6, Sr5Mg0.97Mn0.03La2(BO3)6, and ICDD reference card. | |
Fig. 2 depicts the DR spectra of undoped Sr5MgLa2(BO3)6 as well as of Sr5Mg(La0.99Ce0.01)2(BO3)6 and Sr5Mg0.97Mn0.03La2(BO3)6. The undoped Sr5MgLa2(BO3)6 sample shows an absorption band in the UV range below 400 nm. This band is tentatively assigned to band-to-band absorption of the host structure.9 The absorption in the DR spectrum of Sr5Mg(La0.99Ce0.01)2(BO3)6 is assigned to the spin and parity allowed [Xe]4f1–[Xe]5d1 interconfigurational electric-dipole transition in Ce3+. The DR spectrum of Sr5Mg0.97Mn0.03La2(BO3)6 shows slight absorption at about 470 nm which is ascribed to the transition from the 6A1(6S) ground state to the 4T1(4G) excited state in Mn2+.
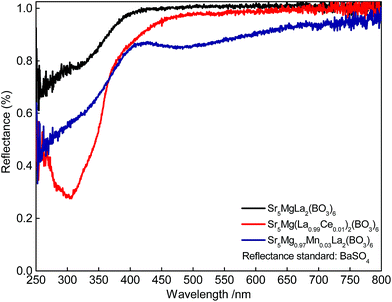 |
| Fig. 2 DR spectra of Sr5MgLa2(BO3)6, Sr5Mg(La0.99Ce0.01)2(BO3)6, and Sr5Mg0.97Mn0.03La2(BO3)6. | |
Room temperature PLE and PL spectra of singly doped Sr5Mg(La0.99Ce0.01)2(BO3)6 and Sr5Mg0.97Mn0.03La2(BO3)6 as well as of co-doped Sr5Mg0.90Mn0.10(La0.99Ce0.01)2(BO3)6 are depicted in Fig. 3a–c. The PLE spectrum of Sr5Mg(La0.99Ce0.01)2(BO3)6 (Fig. 3a) was recorded monitoring the 400 nm-emission and shows a broad band ranging from about 240 to 370 nm. This band consists of various unresolved bands due to excitation from the 2F7/2,5/2 ground state to the [Xe]5d1 multiplet of Ce3+. The PL spectrum of Sr5Mg(La0.99Ce0.01)2(BO3)6 was recorded using an excitation wavelength of λex = 300 nm. The spectrum exhibits a broad band peaking at about 397 nm and a tailing towards lower energy of the electromagnetic range. This emission band occurs due to radiative relaxation from the excited 2Dj state of the [Xe]5d1 configuration to the 2F7/2,5/2 ground state of Ce3+. The tailing is caused by Ce3+ ions occupying different lattice sites and will be discussed later in the article in more detail. Fig. 3b illustrates the PLE and PL spectra of Sr5Mg0.97Mn0.03La2(BO3)6. Monitoring the 715 nm-emission of Mn2+, the obtained PLE spectrum exhibits various bands at about 305, 319, 354, 378, 398, 415 and 474 nm. These bands were attributed to transitions from the 6A1(6S) ground state to 4T1(4F), 4A2(4F), 4T1(4P), 4E(4D), 4T2(4D), [4E(4G)4A1(4G)], and 4T1(4G), respectively. The PL spectrum of Sr5Mg0.97Mn0.03La2(BO3)6 shows a broad band with a maximum at about 714 nm and a tailing to the long wavelength range. This emission is due radiative relaxation from the 4T1(4G) excited state to the 6A1(6S) ground state in Mn2+. PLE and PL spectra of Sr5Mg0.90Mn0.10(La0.99Ce0.01)2(BO3)6 are depicted in Fig. 3c. PLE spectra were recorded monitoring the emission of Mn2+ and Ce3+ at 715 and 400 nm, respectively. The obtained PLE spectra appear similar in shape indicating the occurrence of ET from Ce3+ ions to Mn2+ ions. The PL spectrum was recorded upon excitation with a wavelength of λex = 300 nm and shows two bands peaking at about 403 and 713 nm. These bands originate from radiative relaxation from [Xe]5d1 to 2F7/2,5/2 in Ce3+ and from 4T1(4G) to 6A1(6S) in Mn2+.
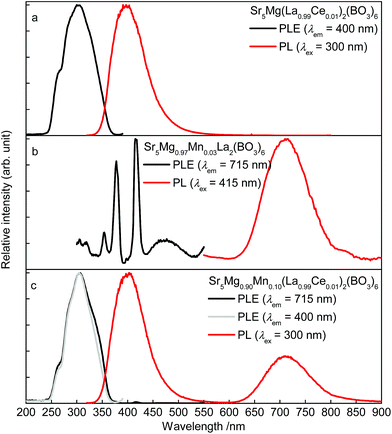 |
| Fig. 3 Room temperature PLE and PL spectra of Sr5Mg(La0.99Ce0.01)2(BO3)6 (a), Sr5Mg0.97Mn0.03La2(BO3)6 (b), Sr5Mg0.90Mn0.10(La0.99Ce0.01)2(BO3)6 (c). | |
For further investigations on the origin of the tailing of the Ce3+ emission band, a PL spectrum of Sr5Mg(La0.99Ce0.01)2(BO3)6 was recorded at 2.9 K. This spectrum is illustrated in Fig. 4. Commonly, Ce3+ emission consists of a doublet band due to spin–orbit-splitting of the 2F state ([Xe]4f1 configuration) into the 2F7/2 and 2F5/2 states. The energetic difference between the 2F7/2 and 2F5/2 states usually amounts to about 2000 cm−1.13 Therefore, it should be possible to fit the present Ce3+ emission band with two Gaussian curves with an approximate distance of 2000 cm−1. However, fitting the current PL spectrum with two Gaussian curves leads to an approximate distance of about 1399 cm−1. This indicates the occupation of more than one distinct crystallographic site. This is thoroughly possible since Sr5MgLa2(BO3)6 provides also 6-fold coordinated Sr2+ sites aside from the 9-fold coordinated site. Against this, fitting the Ce3+ emission band with a sum of four Gaussian curves A, B, C, and D results in approximate distances of 2018 and 1979 cm−1. Based on this result, it is assumed that the Ce3+ ions also occupy the 6-fold coordinated Sr2+ sites. Since crystal field splitting of the 5d states decreases with increasing coordination number, the energetic difference between d and f states increases. Therefore, it is concluded that curves A and B belong to Ce3+ occupying a 6-fold coordinated Sr2+ site (Ce2) whereas curves C and D were assigned to Ce3+ on a 9-fold coordinated site (Ce1). The occupation of two different crystallographic sites is also reflected in the PLE spectra depicted in the inset of Fig. 4. Monitoring the emission of Ce3+ at 345, 380, and 415 nm results in PLE spectra looking significantly different in shape. Shifting the monitored wavelength from 345 to 415 nm, the obtained PLE spectrum gets broader and a new band arises. This observation also indicates PL from different crystallographic sites.
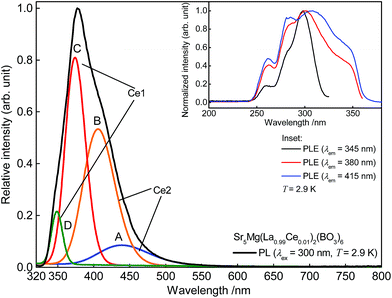 |
| Fig. 4 Low temperature PL spectra of Sr5Mg(La0.99Ce0.01)2(BO3)6 and Gaussian curves A, B, C, and D. Inset: low temperature PLE spectra of Sr5Mg(La0.99Ce0.01)2(BO3)6. | |
Fig. 5 depicts PL spectra of Sr5Mg1−xMnx(La0.99Ce0.01)2(BO3)6 with x = 0.000, 0.005, 0.010, 0.020, 0.050, 0.080, 0.100, 0.150, and 0.200. Up to a Mn2+ content of x = 0.100 PL intensity of Mn2+ increases. At higher Mn2+ contents, the PL intensity of Mn2+ starts to decrease due to concentration quenching. Furthermore, with increasing Mn2+ content the Ce3+ PL intensity decreases implying the occurrence of ET from Ce3+ to Mn2+.
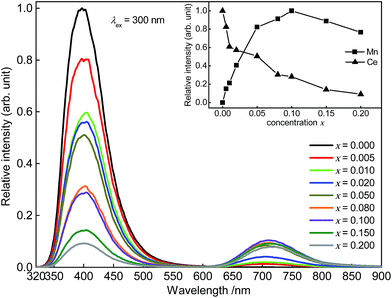 |
| Fig. 5 PL spectra of Sr5Mg1−xMnx(La0.99Ce0.01)2(BO3)6. Inset: integrated PL intensity in dependence of the Ce3+ and Mn2+ concentration. | |
Further, fluorescence lifetime measurements were performed monitoring the Ce3+ emission of co-doped Sr5Mg1−xMnx(La0.99Ce0.01)2(BO3)6. The obtained PLD curves are plotted in Fig. 6 and can be best fitted with a bi-exponential function using the following equation:
|
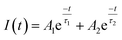 | (2) |
here,
I is the PL intensity at the time
t,
A1 and
A2 are fitting parameters, and
τ1 and
τ2 are the partial fluorescence lifetimes of the exponential components. The bi-exponential behaviour of the PLD curves reflects PL of two different crystallographic sites as detailed above. The average fluorescence lifetimes
τ of the Ce
3+ emission were obtained applying
eqn (3):
|
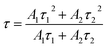 | (3) |
Calculated average fluorescence lifetimes
τ as well as the emission fractions frac
1 and frac
2 are summarized in
Table 1. It turned out that with increasing Mn
2+ content fluorescence lifetimes
τ of the Ce
3+ emission decreases. Since ET processes are magnitudes faster than radiative transitions, this behaviour indicates the occurrence of ET from Ce
3+ to Mn
2+. The ET efficiency
ηT can be calculated using the following equation:
14 |
 | (4) |
here,
τS and
τS0 are the fluorescence lifetimes of Ce
3+ in presence and absence of Mn
2+. The calculated values for
ηT are summarized in
Table 1 and also plotted in the inset of
Fig. 6. It can be seen that the values for
ηT show a continuous growth with increasing Mn
2+ content.
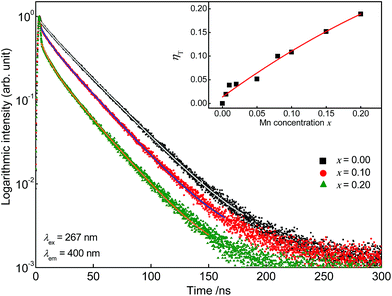 |
| Fig. 6 PLD curves of Sr5Mg1−xMnx(La0.99Ce0.01)2(BO3)6. Inset: ET transfer efficiency ηT in dependence of the Mn2+ concentration. | |
Table 1 Fluorescence lifetimes τ as well as partial lifetimes τ1 and τ2 and the emission fractions frac1 and frac2 of Ce3+ and ET efficiency ηT in Sr5Mg1−xMnx(La0.99Ce0.01)2(BO3)6
Sample (x) |
frac1 (%) |
τ1 (ns) |
frac2 (%) |
τ2 (ns) |
τ(ns) |
ηT |
0.000 |
4 |
9.3 |
96 |
31.0 |
30 |
0 |
0.005 |
5 |
9.4 |
95 |
30.7 |
30 |
0.02 |
0.010 |
5 |
8.9 |
95 |
30.1 |
29 |
0.04 |
0.020 |
4 |
7.5 |
96 |
30.0 |
29 |
0.04 |
0.050 |
6 |
8.5 |
94 |
29.9 |
29 |
0.05 |
0.080 |
7 |
6.9 |
93 |
28.7 |
27 |
0.10 |
0.100 |
7 |
6.3 |
93 |
28.5 |
27 |
0.11 |
0.150 |
11 |
7.6 |
89 |
27.8 |
26 |
0.15 |
0.200 |
12 |
6.7 |
88 |
27.0 |
25 |
0.19 |
To identify the nature of the ET from Ce3+ to Mn2+ in co-doped Sr5La2Mg(BO3)6:Ce3+,Mn2+ Dexter's approach of exchange interactions and multipolar interactions was used.
On the basis of Dexter's ET formula and Reisfeld's approximation the following relations are obtained:15
|
 | (5) |
|
 | (6) |
In these equations
η0 and
η are the internal luminescence quantum efficiencies of Ce
3+ in the absence and presence of Mn
2+.
C is the concentration of Mn
2+ in the samples.
Eqn (5) corresponds to exchange interaction whereas
eqn (6) with
α = 6, 8, and 10 corresponds to dipole–dipole, dipole–quadrupole, and quadrupole–quadrupole interaction, respectively.
η0 and
η can approximately be estimated by the fluorescence lifetimes
τ0 and
τ.
Fig. 7 shows the plots of relation (5) and (6). A linear behaviour is only observed for relation (5) and suggests ET
via exchange interaction.
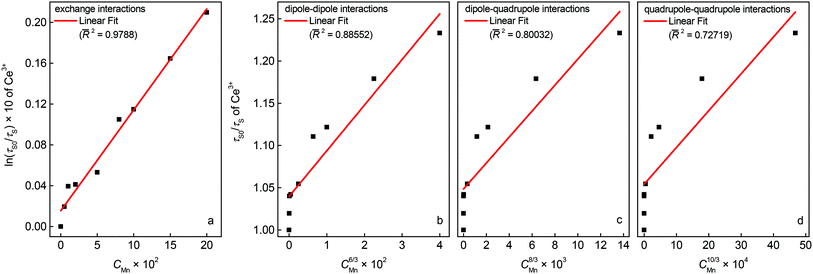 |
| Fig. 7 Dependence of ln(τ0/τ) on CMn (a) and τ0/τ on C6/3 (b), C8/3 (c), and C10/3 (d). | |
To validate this result, the integrated PL intensities of the Ce3+ and Mn2+ emission were investigated using van Uitert's approach:16
|
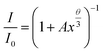 | (7) |
here,
I and
I0 are the luminescence Intensities in the absence and presence of Mn
2+,
A is a constant, and
x is the concentration of Mn
2+ ions.
θ = 3, 6, 8, and 10 represents exchange interaction, dipole–dipole, dipole–quadrupole, and quadrupole–quadrupole interactions, respectively.
17 Experimental data is plotted in
Fig. 8 and best fitting is obtained when plugging in 3 for
θ. This also suggests that ET from Ce
3+ to Mn
2+ in Sr
5MgLa
2(BO
3)
6:Ce
3+,Mn
2+ occurs
via exchange interaction. This observation is rather uncommon for the ET from Ce
3+ and Mn
2+. Since exchange interactions proceed only over short distances this result implies a non-statistical distribution of the Ce
3+ and Mn
2+ ions. To estimate the critical distance between Ce
3+ and Mn
2+ further investigations were carried out using the Inokuti–Hirayama equation for exchange interaction:
18 |
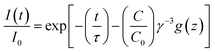 | (8) |
In this equation
I(
t) is the intensity at a certain time and
I0 is the intensity after excitation.
t is the time and
τ is the intrinsic fluorescence lifetime of Ce
3+ in absence of Mn
2+.
C is the concentration of Mn
2+ ions per unit volume and
C0 is the critical concentration.
γ is the transfer volume and
g(
z) is defined as
|
 | (9) |
here,
z and
y are defined as
|
 | (10) |
|
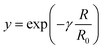 | (11) |
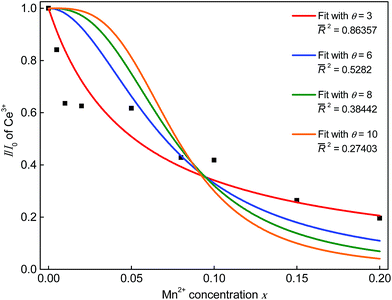 |
| Fig. 8 Dependence of I/I0 on the Mn2+ concentration. | |
Eqn (9) can be expressed by a Taylor series as described by Inokuti and Hirayama which converges. This leads to the following formula:
|
 | (12) |
For the coefficients
h1,
h2, and
h3 the values 1.73164699, 5.93433597, and 5.44487446, respectively, given by Inokuti and Hirayama were plugged in. Fitting the PLD curves of the investigated samples with
eqn (12) yields the
C/
C0 values.
C/
C0 values are plotted against 3/(4π
Rc3) in
Fig. 9. Since
C0 = 3/(4π
Rc3), the slope of a linear fit through the data points is equal to
Rc3.
18,19 Therefore, the critical distance
Rc for ET from Ce
3+ to Mn
2+ was calculated to be 4.6 Å.
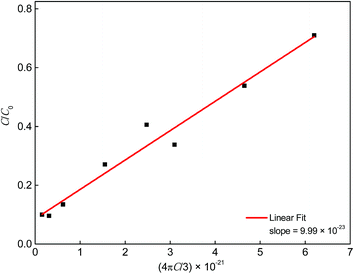 |
| Fig. 9 Dependence of C/C0 on 4πC/3. | |
To investigate the temperature behaviour of the PL of Sr5Mg0.90Mn0.10(La0.99Ce0.01)2(BO3)6 PL spectra from 100 to 500 K were recorded. The obtained PL spectra are illustrated in Fig. 10. Due to thermal quenching, the PL intensity of Ce3+ and Mn2+ decreases with increasing temperature. In addition, the maximum of the Mn2+ emission band shows a shift towards higher energy of the electromagnetic spectrum. Since the equilibrium distance between the Mn2+ ions and its oxygen ligands extends with increasing temperature, the energy difference of the 4T1(4G) → 6A1(6S) transition increases.20 Therefore, the maximum of the Mn2+ emission is shifted towards the blue range. The inset of Fig. 10 depicts the PL integrals of the Ce3+ and Mn2+ emission bands in dependence of temperature. By fitting the data points with a Fermi–Dirac distribution activation energy EA for thermal quenching can be calculated.
|
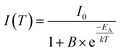 | (13) |
here,
I(
T) is the integrated PL intensity at a certain temperature and
I0 is the PL intensity at zero Kelvin.
B is the frequency factor for thermal quenching,
T is the temperature and
k is the Boltzmann constant (
k = 8.617 × 10
−5 eV K
−1). The values of the parameters
I0 and
B were derived from the fitting function and are about 0.95 and 333, respectively. Fitting the data points with this equation yields an activation energy of
EA = 0.18 eV. From this the
T1/2 value can be derived by using the following relation:
|
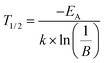 | (14) |
T1/2 describes the temperature at which PL intensity of a luminescent centre is decreased to one-half of its maximum intensity. For the investigated Sr
5Mg
0.90Mn
0.10(La
0.99Ce
0.01)
2(BO
3)
6 sample
T1/2 was calculated to be 355 K.
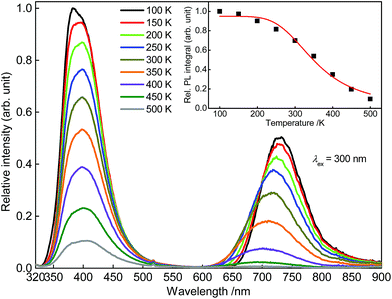 |
| Fig. 10 PL spectra of Sr5Mg0.90Mn0.10(La0.99Ce0.01)2(BO3)6 from 100 to 500. Inset: integrated PL intensity in dependence of temperature. | |
For further investigations on the origin of thermal quenching in Sr5Mg0.90Mn0.10(La0.99Ce0.01)2(BO3)6 fluorescence lifetime measurements were performed. Therefore, PLD curves of the Ce3+ as well as of the Mn2+ emission were recorded from 100 to 500 K. Fig. 11 depicts the obtained PLD curves monitoring the 400 nm-emission of Ce3+. The PLD curves show a bi-exponential behaviour reflecting PL from two distinct crystallographic sites as mentioned above. The fluorescence lifetimes of Ce3+ are illustrated in the inset of Fig. 11 and are summarized in Table 2. From this finding it can be derived that the fluorescence lifetimes remain stable up to a temperature of 300 K. At 350 K the fluorescence lifetimes start to decrease indicating a decline of radiative transitions due to thermal quenching. Fig. 12 shows the PLD curves of Mn2+ monitoring the emission at 725 nm. It is obvious from the inset that the Mn2+ PL lifetimes decrease continuously over the complete temperature range. Fitting the fluorescence lifetimes of Ce3+ and Mn2+ with eqn (13) yields the T1/2 values for the Ce3+ (T1/2,Ce) and Mn2+ (T1/2,Mn) emission. T1/2,Ce and T1/2,Mn were calculated to be 492 and 323 K, respectively. This result indicates that the overall thermal quenching of the PL in Sr5Mg0.90Mn0.10(La0.99Ce0.01)2(BO3)6 is mainly caused by the presence of Mn2+.
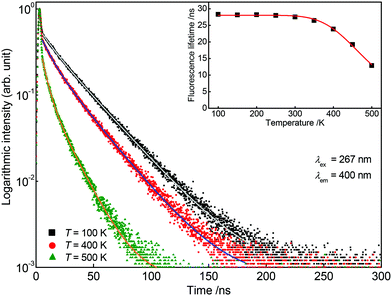 |
| Fig. 11 Obtained PLD curves of Sr5Mg0.90Mn0.10(La0.99Ce0.01)2(BO3)6 at 100, 400, and 500 K monitoring the PL of Ce3+. Inset: Fluorescence lifetimes of the Ce3+ emission in dependence of temperature. | |
Table 2 Fluorescence lifetimes τ as well as partial lifetimes τ1 and τ2 and the emission fractions frac1 and frac2 of Ce3+ and Mn2+ in Sr5Mg1−xMnx(La0.99Ce0.01)2(BO3)6 from 100 to 500 K
T (K) |
Ce3+ |
Mn2+ |
τ1 (ns) |
frac1 (%) |
τ2 (ns) |
frac2 (%) |
τ (ns) |
τ1 (ms) |
frac1 (%) |
τ2 (ms) |
frac2 (%) |
τ (ms) |
100 |
10.6 |
8 |
29.7 |
92 |
28 |
14.8 |
6 |
48.2 |
94 |
46 |
150 |
9.6 |
7 |
29.6 |
93 |
28 |
13.1 |
5 |
45.9 |
95 |
44 |
200 |
10.2 |
9 |
30.0 |
91 |
28 |
16.5 |
9 |
42.9 |
91 |
41 |
250 |
8.7 |
7 |
29.5 |
93 |
28 |
16.8 |
13 |
37.9 |
87 |
35 |
300 |
8.7 |
8 |
29.2 |
92 |
28 |
7.8 |
8 |
28.9 |
92 |
27 |
350 |
8.9 |
11 |
28.5 |
89 |
26 |
5.8 |
15 |
20.7 |
85 |
18 |
400 |
9.1 |
17 |
26.8 |
83 |
24 |
3.5 |
27 |
13.8 |
73 |
11 |
450 |
7.2 |
21 |
22.4 |
79 |
19 |
1.7 |
30 |
6.5 |
70 |
5 |
500 |
4.8 |
29 |
16.2 |
71 |
13 |
0.3 |
12 |
1.7 |
88 |
2 |
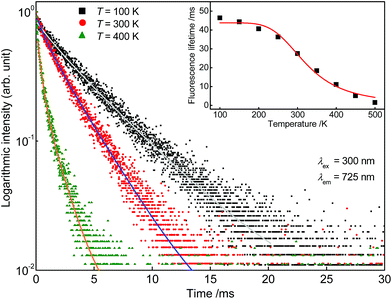 |
| Fig. 12 Obtained PLD curves of Sr5Mg0.90Mn0.10(La0.99Ce0.01)2(BO3)6 at 100, 300, and 400 K monitoring the PL of Mn2+. Inset: Fluorescence lifetimes of the Mn2+ emission in dependence of temperature. | |
The efficiency of the prepared Sr5MgLa2(BO3)6:Ce3+,Mn2+ samples was examined by determining external quantum efficiency ηext as mentioned above. To our understanding, ηext is defined as the ratio between the number of emitted photons Nemission and the number of absorbed photons Nabsorption:
|
 | (15) |
The calculated external quantum efficiencies are summarized in Table 3. It turned out that with increasing Mn2+ concentration ηext continuously decreases. This is probably caused by concentration quenching due to ET between the Mn2+ ions.
Table 3 External quantum efficiencies ηext and CIE colour coordinates of Sr5Mg1−xMnx(La0.99Ce0.01)2(BO3)6
Sample (x) |
ηext |
CIE (x|y) |
0.000 |
0.97 |
(0.160|0.046) |
0.005 |
0.72 |
(0.160|0.046) |
0.010 |
0.53 |
(0.161|0.046) |
0.020 |
0.57 |
(0.164|0.048) |
0.050 |
0.53 |
(0.169|0.050) |
0.080 |
0.33 |
(0.175|0.052) |
0.100 |
0.33 |
(0.178|0.052) |
0.150 |
0.20 |
(0.188|0.059) |
0.200 |
0.18 |
(0.194|0.062) |
To illustrate the colour of the emission, CIE chromaticity coordinates were calculated according to the relevant PL spectra. The obtained colour points are depicted in Fig. 13. All colour points are located in the violet region of the chromaticity coordinates diagram. With increasing Mn2+ content the colour points show a shift towards the red region. Since the Mn2+ emission band is located in the deep red range of the visible spectrum where the eye sensitivity is rather low, the observed shift of the colour points is rather low too. A larger shift into the red region of the chromaticity coordinates diagram using co-doped Sr5MgLa2(BO3)6:Ce3+,Mn2+ could be obtained using a lower Ce3+ content. In this way the Mn2+ emission would be more intense compared to the Ce3+ emission band resulting in a larger shift.
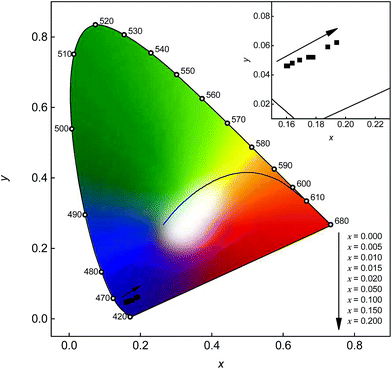 |
| Fig. 13 CIE chromaticity diagram of Sr5Mg1−xMnx(La0.99Ce0.01)2(BO3)6 with different Mn2+ concentrations. Inset: Enlarged section of the relevant range. | |
4. Conclusions
To investigate the PL properties and the ET between Ce3+ and Mn2+ of co-doped Sr5MgLa2(BO3)6:Ce3+,Mn2+ a series of powder samples with various Mn2+ contents was prepared. Sr5MgLa2(BO3)6:Ce3+,Mn2+ shows two emission bands located at 403 and 713 nm upon excitation at λex = 300 nm. Low temperature PL measurement on the Ce3+ emission reveals that the Ce3+ ions mainly occupy the 9-fold coordinated sites as well as the 6-fold coordinated Sr2+ sites. This statement is backed by the bi-exponential PLD curves of Sr5Mg(La0.99Ce0.01)2(BO3)6. The highest PL intensity was found for a Mn2+ content of x = 0.100. Furthermore, it was demonstrated that the ET from Ce3+ to Mn2+ occurs via an exchange interaction mechanism. Critical distance Rc was determined to be 4.6 Å. Temperature depended PL measurements prove a T1/2 value of 355 K for Sr5Mg0.90Mn0.10(La0.99Ce0.01)2(BO3)6. Furthermore, temperature dependent fluorescence lifetimes revealed that thermal quenching is mainly caused by the Mn2+ ions. Finally, the external quantum efficiencies ηext were determined to be close to unity of the Ce3+ doped samples. With increasing Mn2+ ηext continuously decreases. Calculated chromaticity coordinates are all located in the violet region of the CIE diagram. Therefore, co-doped Sr5MgLa2(BO3)6:Ce3+,Mn2+ with the herein investigated doping levels is not suitable as a white or red emitting phosphor.
Acknowledgements
The authors are grateful to Merck KGaA Darmstadt, Germany for generous financial support.
References
-
(a) C. Feldmann, T. Jüstel, C. R. Ronda and P. J. Schmidt, Adv. Funct. Mater., 2003, 13, 511–516 CrossRef CAS PubMed;
(b) E. F. Schubert and J. K. Kim, Science, 2005, 308, 1274–1278 CrossRef CAS PubMed.
- S. Nakamura, P. Stephen and F. Gerhard, The Blue Laser Diode: The Complete Story, Springer-Verlag, Berlin/Heidelberg, 1997 Search PubMed.
- H. S. Jang, W. B. Im, D. C. Lee, D. Y. Jeon and S. S. Kim, J. Lumin., 2007, 126, 371–377 CrossRef CAS PubMed.
- Y. Uchida and T. Taguchi, Opt. Eng., 2005, 44, 124003 CrossRef PubMed.
- M. Shang, C. Li and J. Lin, Chem. Soc. Rev., 2014, 43, 1372 RSC.
-
(a) M. Müller and T. Jüstel, J. Lumin., 2014, 155, 398–404 CrossRef PubMed;
(b) T. Jia, Z. Ci, Q. Wu, G. Zhu, C. Wang and Y. Wang, ECS J. Solid State Sci. Technol., 2015, 4, R78–R82 CrossRef CAS PubMed.
-
(a) R. Yu, H. Li, H. Ma, C. Wang, H. Wang and J. McKittrick, J. Am. Ceram. Soc., 2014, 97, 1151–1156 CrossRef CAS PubMed;
(b) Q. Liu, Y. Liu, Y. Ding, Z. Peng, Q. Yu, X. Tian and G. Dong, J. Sol-Gel Sci. Technol., 2014, 71, 276–282 CrossRef CAS;
(c) Y. Shi, Y. Wen, M. Que, G. Zhu and Y. Wang, Dalton Trans., 2014, 2418–2423 RSC;
(d) L. Wu, B. Wang, Y. Zhang, L. Li, H. R. Wang, H. Yi, Y. F. Kong and J. J. Xu, Dalton Trans., 2014, 13845 RSC.
- K. I. Schaffers, P. D. Thompson, T. Alekel III, J. R. Cox and D. A. Keszler, Chem. Mater., 1994, 6, 2014–2022 CrossRef CAS.
- R. Sankar and G. Subba Rao, J. Alloys Compd., 1998, 281, 126–136 CrossRef CAS.
- R. Sankar, Solid State Sci., 2008, 10, 1864–1874 CrossRef CAS PubMed.
- R. D. Shannon, Acta Crystallogr., Sect. A: Cryst. Phys., Diffr., Theor. Gen. Crystallogr., 1976, 32, 751–767 CrossRef.
- Y. Kawamura, H. Sasabe and C. Adachi, Jpn. J. Appl. Phys., 2004, 43, 7729–7730 CrossRef CAS.
-
(a) F. A. Kröger and J. Bakker, Physica, 1941, 8, 628–646 CrossRef;
(b) G. Blasse and A. Bril, J. Chem. Phys., 1967, 47, 5139–5145 CrossRef CAS PubMed.
-
(a) H. C. Kandpal and H. B. Tripathi, Indian J. Pure Appl. Phys., 1979, 17, 587–589 CAS;
(b) P. Paulose, G. Jose, V. Thomas, N. Unnikrishnan and M. Warrier, J. Phys. Chem. Solids, 2003, 64, 841–846 CrossRef CAS.
-
(a) D. L. Dexter, J. Chem. Phys., 1953, 21, 836 CrossRef CAS PubMed;
(b) D. L. Dexter and J. H. Schulman, J. Chem. Phys., 1954, 22, 1063 CrossRef CAS PubMed;
(c) R. Reisfeld, E. Greenberg, R. Velapoldi and B. Barnett, J. Chem. Phys., 1972, 56, 1698 CrossRef CAS PubMed.
- L. G. van Uitert, J. Electrochem. Soc., 1967, 114, 1048 CrossRef CAS PubMed.
-
(a) L. Zhang, Z. Lu, P. Han, L. Wang and Q. Zhang, J. Nanomater., 2012, 2012, 1–7 Search PubMed;
(b) F. Zhang and W. Tang, Luminescence, 2015, 30, 216–220 CrossRef CAS.
- M. Inokuti and F. Hirayama, J. Chem. Phys., 1965, 43, 1978 CrossRef CAS PubMed.
- J. S. Lee, S. Unithrattil and W. B. Im, J. Alloys Compd., 2013, 555, 297–303 CrossRef CAS PubMed.
- Y. Tanabe and S. Sugano, J. Phys. Soc. Jpn., 1954, 9, 766–779 CrossRef CAS.
|
This journal is © The Royal Society of Chemistry 2015 |