DOI:
10.1039/C5RA12806D
(Paper)
RSC Adv., 2015,
5, 75589-75599
3D welan gum–graphene oxide composite hydrogels with efficient dye adsorption capacity†
Received
2nd July 2015
, Accepted 26th August 2015
First published on 26th August 2015
Abstract
As a renewable material, welan gum is a kind of both biocompatible and biodegradable microbial polysaccharide and can be used to form polysaccharide/inorganic material composites. In this article, welan gum–graphene oxide (GO) composite hydrogels were prepared by simple self-assembly of both components in aqueous media and the effects of GO on the gelation of welan gum were systematically studied. The welan gum–GO hybrid hydrogels have been thoroughly characterized using transmission electron microscopy (TEM), atomic force microscopy (AFM), field emission scanning electron microscopy (FE-SEM), Fourier transform infrared (FT-IR) spectroscopy, X-ray diffraction (XRD), differential scanning calorimetry (DSC) and rheological measurements. It can be observed that GO is dispersed on a molecular scale in the welan gum matrix and the interactions such as hydrophobic interaction, electrostatic interaction and hydrogen bonding occur between the welan gum matrix and graphene oxide sheets. The addition of GO which can act as a physical cross-linker in the hydrogel and effectively promote the gelation of welan gum, can be reflected by a decrease of the critical gelation concentration (CGC). Additionally, the welan gum–GO hybrid hydrogels exhibited good adsorption properties for water-soluble dyes such as methylene blue (MB), methyl violet (MV), amido black 10B (AB10B), rhodamine 6G (R6G) and chrome azurol S (CAS). Thus, it is expected that the GO-based composite hydrogels can act as adsorbents and have promising applications in the field of waste water treatment.
1. Introduction
Polymer hydrogels (PHGs) are materials with viscoelastic properties and network structures caused by a cross-linker and solvent, respectively. Recently, PHG materials have been pervaded in daily life in different forms and have a wide array of applications such as soap, shampoo, toothpaste, hair gel and contact lenses.1–3 Moreover, there are also some advance industrial applications of PHGs such as oil recovery, pharmaceuticals, agriculture, textiles, and water treatment.4–6 Among various polymers, natural polysaccharide and its hydrogels display unique properties such as biodegradability, biocompatibility, stimuli-responsive characteristics and biological functions making them a material of choice for diverse applications.7–15 Furthermore, microbial polysaccharide is a kind of polysaccharide produced by microorganisms in metabolic processes. The presence of microbial polysaccharide can significantly change the nature of aqueous systems in terms of thickening, emulsifying, stabilizing, suspensions and gel formations due to its special physical and chemical properties. Welan gum, a new microbial polysaccharide produced by Alcaligenes sp., consists of a pentasaccharide repeating unit, β-1,3-D-glucopyranosyl, β-1,4-D-glucuronopyranosyl, β-1,4-D-glucopyranosyl, x-1,4 L-rhamnopyranosyl, and a single monosaccharide side-chain at O-3 of the 4-linked glucopyranosyl.16–18 As a renewable material, welan gum is both biocompatible and biodegradable and it has potential industrial applications in food, concrete, petroleum, ink and other industries.19 Especially in the oil industry, welan gum is expected to become a novel oil recovery agent due to its excellent rheological properties.20
However, the relatively weak mechanical properties of hydrogels remain a major drawback for their applications in industry and biological medicine.21,22 Recently, a lot of research work has been devoted to doping nanofillers such as silica, clay, and carbon nanotubes which is considered to be an effective way to enhance the mechanical strength of the hydrogels, to construct the composite hydrogels and improve the mechanical performance of the hydrogels.23,24 As a new kind of carbon material, graphene oxide (GO), having a large number of oxygen-containing groups, such as hydroxyl, epoxide and carboxyl groups on the basal planes and edges, is one of the most important derivatives of graphene.25,26 As a result, it makes GO behave like an amphiphilic macromolecule with hydrophilic edges and a more hydrophobic basal plane, and possess special hydrophilic properties which can be dispersible in water as well as other solvents.27 These properties also make GO an attractive building block for the construction of various self-assembly architectures and can be easily modified to be compatible with polymers for enhancing the mechanical performance of hydrogels.28–30 Moreover, the single layer structure endows GO sheets with an ultra-large specific surface area and a highly negative-charged surface. These chemical properties confer GO with ideal characteristics of an excellent adsorbent.31–33
It can be expected that if welan gum and graphene oxide can form composite materials, they may have more excellent properties and applications. In this article, the effects of GO on the gelation of welan gum were systematically studied and welan gum–GO hybrid hydrogels were prepared. We characterized their performance through phase behavior observation, transmission electron microscopy (TEM), atomic force microscopy (AFM), field emission scanning electron microscopy (FE-SEM), Fourier transform infrared (FT-IR) spectroscopy, X-ray diffraction (XRD), differential scanning calorimetry (DSC) and rheological measurements. Moreover, dye adsorption experiments were also done to investigate whether toxic dye, i.e., methylene blue (MB), methyl violet (MV), amido black 10B (AB10B), rhodamine 6G (R6G), chrome azurol S (CAS) and methyl orange (MO) can be efficiently entrapped in the welan gum–GO hybrid hydrogels. This work is an extension of work in our laboratory and it provides another example for the preparation of high-performance polymer nanocomposites by using GO as a nanofiller. It can be expected that welan gum–GO hydrogels with largely improved mechanical properties may play an important role in water treatment, biochemical and electrochemical applications.
2. Experimental
Materials
Welan gum was supplied by Hebei Hengbiao Bio-technology Co., Ltd, China. The molecular weight is about 6.6 × 105 to 9.7 × 105 g mol−1, and the intrinsic viscosity is 4479 mL g−1. Graphene oxide (diameter: 0.5–5 μm; thickness: 0.8–1.2 nm; single layer ratio: ∼99%; purity: ∼99%) was obtained from Nanjing XFNANO Materials Tech Co., Ltd and was used as received. The structures of welan gum and GO are displayed in Fig. 1A and B. Dyes (p.a. quality) were purchased from Sinopharm Chemical Reagent Co., Ltd (Shanghai, China). Water used in the experiment was triply distilled by a quartz water purification system.
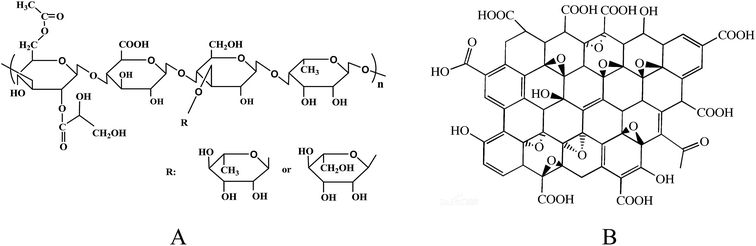 |
| Fig. 1 The structures of welan gum (A) and GO (B). | |
Preparation of the samples
Preparation of the welan gum solution: a certain amount of welan gum was added to deionized water, swelled for one day and stirred continuously at 25 °C until dissolved. Then the samples were kept at 20 °C for two weeks before characterization.
Preparation of the GO dispersion: the desired amount of dried GO was exfoliated and dispersed in deionized water by sonication for 4 h. GO sponge was fabricated by employing a freeze-drying method with 7 mg mL−1 GO colloidal suspension, and used as the control sample to compare the adsorption capacities of various hazardous molecules.
Preparation of the welan gum–GO composite hydrogels: the GO dispersion and welan gum solution were mixed together at a certain ratio. The mixture was shaken violently for 50 s. The composite gels were kept at 20 °C for two weeks before characterization. Finally, the hydrogels were freeze-dried to obtain the welan gum–GO composite gel.
Methods and characterization
TEM observations were carried out on a JEOL JEM-100 CXII (Japan) at an accelerating voltage of 80 kV. Samples were prepared by directly dipping an ultrathin carbon-coated copper grid into the sample, which was then dried by using an NIR lamp before observations. FE-SEM observations were carried out on a JSM-6700F. Atomic force microscopy (IIIa AFM) was used to observe the morphologies of GO dispersion. The samples for AFM measurements were prepared by adding the GO dispersion dropwise onto a freshly cleaved silica piece. Subsequently, the samples were air-dried.
UV-vis measurements were carried out on a computer-manipulated spectrometer (UV-vis 4100, Hitachi, Japan). FT-IR spectra were recorded on a VERTEX-70/70v spectrometer (Bruker Optics, Germany). XRD patterns of the freeze-dried samples were obtained between 5° and 90° in the 2θ scan mode (2.5° min−1) using a Rigaku D/Max 2200 PC diffractometer with Cu Kα radiation (λ = 0.15418 nm) and a graphite monochromator at room temperature. Gel–sol transition temperatures were obtained from a DSC8500 (PerkinElmer, Waltham, MA, USA). The heating rate was set as 3 °C min−1. TGA data were collected using a Universal V3.6 TA Thermal Analysis Q5000 system. The sample was placed in a platinum crucible and heated under a flow of N2 from ∼50 °C to 800 °C at a heating rate of 10 °C min−1.
The rheological measurements were carried out on a HAAKE RS75 rheometer with a cone–plate system (Ti, diameter, 35 mm; cone angle, 1°). For the shear-dependent behavior, the viscosity measurements were carried out at shear rates ranging from 0 to 1000 s−1. For oscillatory measurements, an amplitude sweep at a fixed frequency of 1 Hz was performed prior to the following frequency sweep in order to ensure that the selected stress was in the linear viscoelastic region. The viscoelastic properties of the samples were determined using oscillatory measurements in the frequency range of 0.01–10 Hz. The samples were measured at 20.0 ± 0.1 °C with the help of a circulating water bath.
3. Results and discussion
Dispersion of GO
First, because of the hydrophilicity of GO, it can be dispersed directly in water through an ultrasonication process and can stay stable for several months without precipitation. TEM (Fig. 2A) and AFM (Fig. 2B) identified the 2D single-layer of the dispersions of GO. It can be seen that the morphology of GO is consistent by using these two different microscopes and the images clearly exhibit flat sheets with some wrinkles. The thickness, determined from the height profile of the AFM image is around 1.4 nm, verifying the characteristic single-layered 2D structure of GO nanosheets. Moreover, there are also some places that the height of the GO is nearly a multiple of 1.4 nm, indicating that there maybe several layers of GO superimposed together. Thus, these results demonstrated that most of the GO was exfoliated into individual sheets by ultrasonic treatment, which can be attributed to the various oxygen-containing functional groups, such as –COOH and –OH on the surface of the GO sheet.34,35
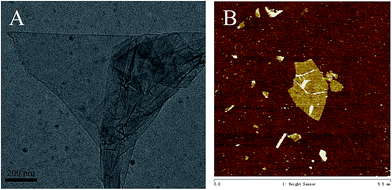 |
| Fig. 2 TEM (A) and AFM (B) images for GO on freshly cleaved mica. | |
Phase behavior of the welan gum–GO mixed system
Then, the phase behavior of the welan gum–GO mixed system was investigated to determine the effects of GO on the gelation of welan gum. It can be seen from Fig. 3A that with the increase of the concentration of welan gum, the welan gum solution will transform into a sol and finally to the hydrogel phase both in the absence and presence of GO. The pure welan gum solution can form a hydrogel at cwelan gum = 7 mg mL−1 without the addition of GO. When GO was added and the concentration of GO (cGO) is fixed at 1 mg mL−1, 3 mg mL−1 and 5 mg mL−1, the hydrogels formed at cwelan gum = 6 mg mL−1, 5 mg mL−1, 3 mg mL−1, respectively (Fig. 3B). Thus, it can be concluded that cGO is crucial to determine the critical gelation concentration (CGC) of the welan gum–GO mixed system. Moreover, it can be revealed that GO can function as an efficient crosslinking reagent which promotes the formation of welan gum–GO composite hydrogels and the photographs of the welan gum–GO mixed system are shown in Fig. 3C–E. Next, in this work, we focus on the properties of the hydrogel phase of the welan gum–GO mixed system, including the gelation ability, the microstructure, and the mechanical strength to further study the effects of GO on the gelation of welan gum.
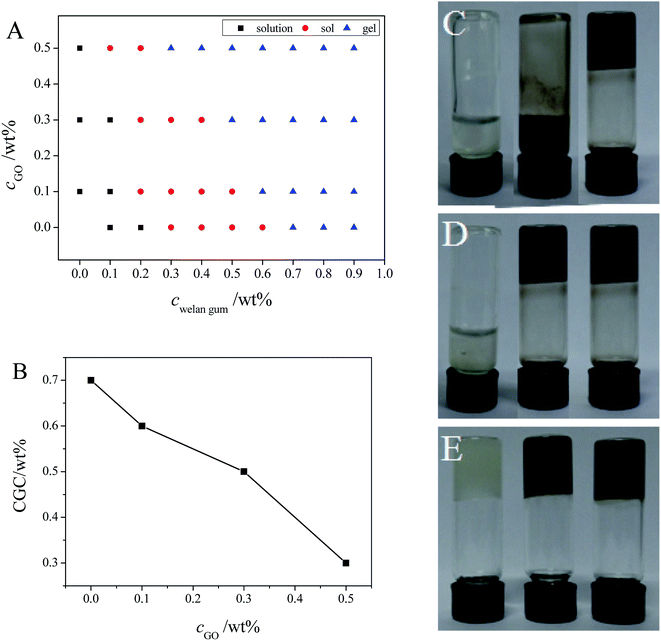 |
| Fig. 3 (A) Phase behavior of the welan gum–GO mixed system after being equilibrated at 20.0 ± 0.1 °C for at least 2 weeks. (B) The variation of the CGC of the welan gum–GO mixed system as a function of cGO. Photographs of 5 mg L−1 (C), 6 mg L−1 (D), and 8 mg L−1 (E) of welan gum mixed with different concentrations of GO. cGO is (from left to right) 0, 1 and 5 mg mL−1, respectively. | |
Microstructures of the welan gum–GO hydrogels
TEM and FE-SEM images were used to characterize the structure of the welan gum–GO composite hydrogels. From the TEM results, it can be seen that there are only some welan gum aggregates for the 8 mg mL−1 pure welan gum hydrogel (Fig. 4A). With the addition of 5 mg mL−1 GO, it can be clearly seen that welan gum and GO coexist in a mixed system. So, it can be inferred that GO can act as crosslinking reagents and form welan gum/GO complexes and can promote the formation of welan gum–GO (Fig. 4B), and the schematic representation of the network structure of the welan gum–GO hybrid hydrogels is shown in Scheme 1. Moreover, if the concentration of welan gum increased, it can be observed that the welan gum aggregates also increased, therefore it can be said that the increase of the concentration of welan gum or GO is beneficial for the formation of strong hydrogels (Fig. 4C).
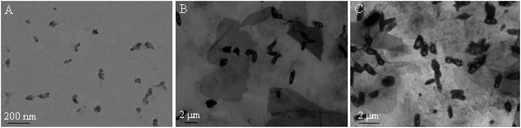 |
| Fig. 4 TEM images of the hydrogels: (A) 8 mg mL−1 welan gum hydrogel, (B) 5 mg mL−1 welan gum/5 mg mL−1 GO hydrogel, (C) 8 mg mL−1 welan gum/5 mg mL−1 GO hydrogel. | |
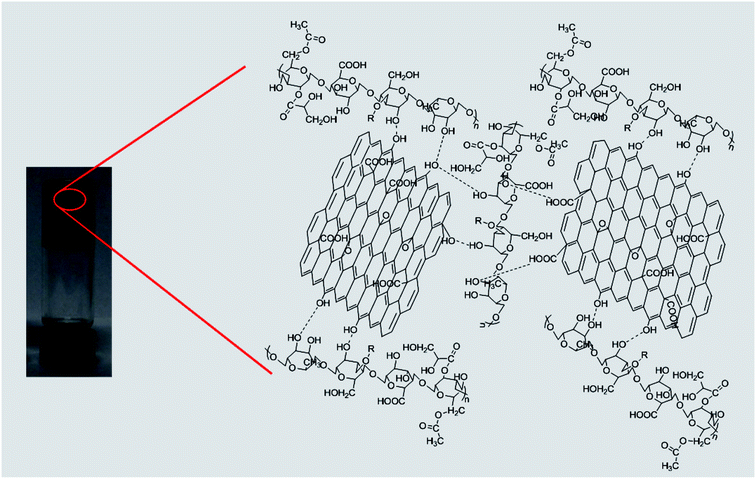 |
| Scheme 1 Schematic representation of the network structure of the welan gum–GO hybrid hydrogels. | |
For the SEM results, firstly, pristine GO sponge without microbial polysaccharide welan gum was prepared by direct freeze-drying for comparison. As shown in Fig. 5A, the GO sponge exhibits a loose 3D structure consisting of 2D GO sheets. For the freeze-dried 8 mg mL−1 welan gum hydrogel, it is composed of a lot of fibers to form the network structure (Fig. 5B). After introduction of GO, the morphologies of the welan gum hydrogels were changed. No obvious welan gum or GO aggregations are found in the FE-SEM images for these hybrid hydrogels, indicating a uniform distribution of the welan gum chains with GO sheets. It can be seen that GO was well inserted into networks of the hydrogel and resulted in the interconnected structure of networks and with the increase in the concentration of GO (Fig. 5C), the morphology of the welan gum–GO composite hydrogels becomes larger and tends to form a graphene oxide structure. However, if we fixed the concentration of GO at 5 mg mL−1 and changed the concentration of welan gum from 5 mg mL−1 to 8 mg mL−1 (Fig. 5D and E), it can be seen that the morphology of the welan gum–graphene oxide composite hydrogels becomes smaller and tends to form a welan gum structure (Fig. 5F).
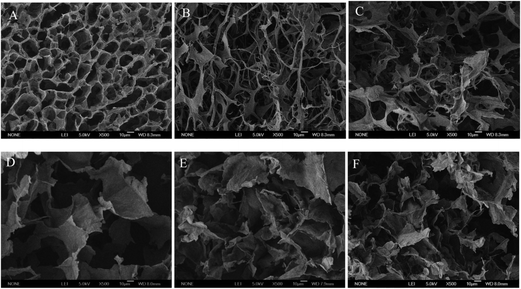 |
| Fig. 5 SEM images of the hydrogels: (A) freeze-dried 5 mg mL−1 GO solution, (B) freeze dried 8 mg mL−1 welan gum hydrogel, (C) freeze dried 8 mg mL−1 welan gum/1 mg mL−1 GO hydrogel, (D) freeze dried 5 mg mL−1 welan gum/5 mg mL−1 GO hydrogel, (E) freeze dried 7 mg mL−1 welan gum/5 mg mL−1 GO hydrogel, (F) freeze dried 8 mg mL−1 welan gum/5 mg mL−1 GO hydrogel. | |
The interconnected structure of the networks enables the diffusion of solute through the hydrogel, which is crucial for further applications in toxic molecule or heavy metal ion adsorption33 and it can be expected that these different compositions and morphologies of the welan gum–GO composite hydrogels can have great different effects on the adsorption capacity. The detailed results are shown in dye adsorption of welan gum–GO hybrid hydrogels part.
Rheological properties of the welan gum–GO hydrogels
The rheological properties of the welan gum–graphene oxide composite hydrogels were investigated at different concentrations and temperatures. When the samples are sheared under an increasing stress, the solid network structure of the hydrogels will break suddenly at a critical shear stress, τ*, which is also called “yield stress” and exhibit a Newtonian-like behavior, reflecting the strength of the network structures of the hydrogels.36 The amplitude sweep (f = 1 Hz) in Fig. 6A indicates the weak dependence of the elastic modulus (G′, 6.83 Pa) and viscous modulus (G′′, 2.44 Pa) on the applied stress, until τ* (6.34 Pa) is reached for the 8 mg mL−1 welan gum hydrogel. When GO was doped, the mechanical intensity of the composite hydrogels can be greatly enhanced. For example, the G′ and G′′ values increase to 29.36 and 10.28 Pa, respectively, when the concentration of GO is 5 mg mL−1. Meanwhile, τ* is also improved to 12.29 Pa. This indicated that the strength of the network structures of the hydrogels was improved after the addition of GO. The frequency sweeping results also demonstrated the reinforcing effect of GO nanosheets on welan gum hydrogels. It can be seen that both the elastic modulus (G′) and viscous modulus (G′′) increased (Fig. 6B) in the frequency range from 0.01 to 1 Hz after the introduction of GO nanosheets, and G′ is higher than G′′ over the investigated oscillating frequency range and η* decreases with the increase of frequency (Fig. 6C) which is typical gel-like behavior. At 0.1 Hz, the G′ of 8 mg mL−1 welan gum is 4.37 Pa, and it increases to 4.97 and 7.36 Pa, respectively, as the concentration of GO is 1 mg mL−1 and 5 mg mL−1. Thus, the enhanced dynamic viscoelastic properties of the welan gum–GO composite hydrogels further suggest the excellent reinforcing effect of GO nanosheets in welan gum hydrogels. Moreover, it can be seen from Fig. 6D that as the shear rate is increased, the viscosity is decreased, indicating the shear-thinning behavior of the system. As discussed above, the oxygen-containing groups on the GO surface can interact effectively with welan gum through hydrogen bonding. The compatibility and strong interaction between GO and the welan gum matrix greatly enhances the dispersion of the GO sheets at the molecular scale in the welan gum matrix and then significantly increases the mechanical properties of the composite hydrogels.37 A similar experimental conclusion can be obtained if we fixed the concentration of GO and changed the concentration of welan gum, the mechanical properties of the composite hydrogels increased as the concentration of welan gum increased and the results are shown in Fig. S1.†
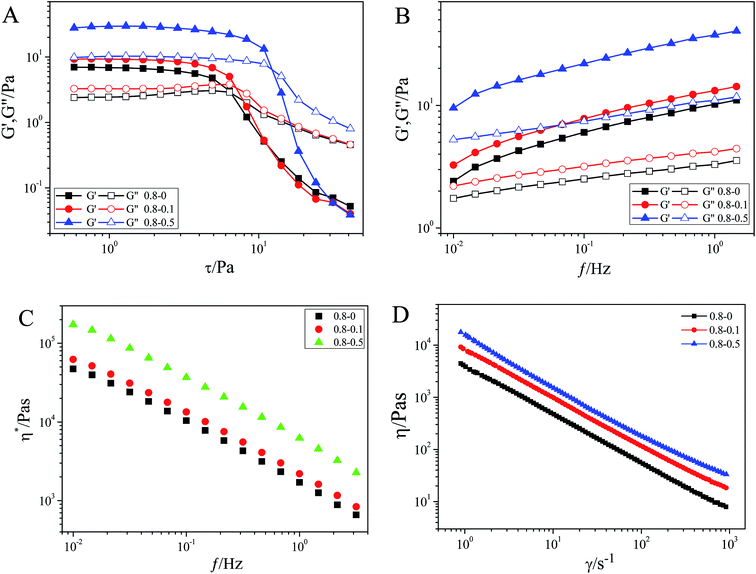 |
| Fig. 6 Rheological results for the welan gum–GO composite hydrogels formed by 8 mg mL−1 welan gum with different concentrations of GO measured at 20.0 ± 0.1 °C. (A) Elastic modulus (G′) and viscous modulus (G′′) as a function of the applied stress at a constant frequency (1.0 Hz); variation of G′ and G′′ (B) and complex viscosity (C) as a function of frequency. (D) Variation of shear viscosity as a function of shear rate. | |
FT-IR and XRD analysis
FT-IR is an important method to characterize the interactions of self-assembling supramolecular hydrogels and it can be used to investigate the interaction between welan gum and GO. As shown in Fig. 7A, the characteristic vibrations of GO are the broad and intense peak of O–H groups centered at 3446 cm−1, strong C
O peak at 1633 cm−1, –OH bending vibration of carboxyl group at 1386 cm−1, the C–OH stretching peak at 1266 cm−1, and the C–O stretching peak at 1044 cm−1.38 In the spectrum of welan gum, the broad band at 3419 cm−1 corresponds to the hydroxyl groups, the peaks at 1727 cm−1 are characteristic of the C
O stretch of the carboxylic group of welan gum, and the peaks near 1628 and 1452 cm−1 were caused by symmetric and asymmetric stretching vibrations of the –COO− groups, respectively.39 For welan gum–GO hybrid hydrogels, it can be seen that both the characteristic peaks of welan gum and GO can be observed but no obvious change of peak position occurs which indicates the formation of mixtures of welan gum and GO.
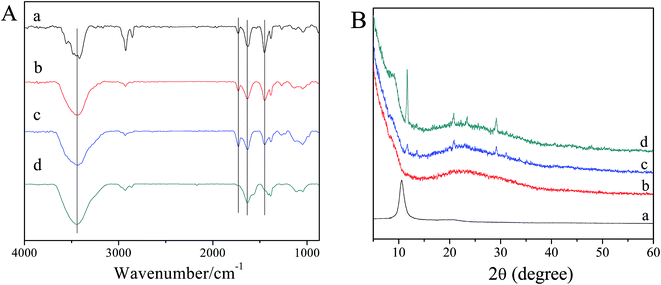 |
| Fig. 7 (A) FT-IR spectra of freeze-dried samples composed of (a) 8 mg mL−1 welan gum, (b) 8 mg mL−1 welan gum/1 mg mL−1 GO; (c) 8 mg mL−1 welan gum/5 mg mL−1 GO; (d) GO. (B) XRD patterns of (a) pure GO, (b) pure welan gum, (c) 8 mg mL−1 welan gum/1 mg mL−1 GO and (d) 8 mg mL−1 welan gum/5 mg mL−1 GO composite hydrogels. | |
XRD patterns of the welan gum–GO composite hydrogels further determine that graphene-based sheets are indeed present as individual graphene sheets in the composites. The XRD pattern of pure GO shows the characteristic peaks of GO around 2θ = 10.6°, which is a clear indication of the structure of GO. After GO is incorporated into the welan gum matrix, the XRD pattern of the welan gum–GO composite hydrogels shows mainly the welan gum diffraction peaks and the diffraction peak of GO nearly disappears, which clearly demonstrates the formation of a fully exfoliated structure of GO sheets in the polymer matrix and the disappearance of the regular and periodic structure of GO.40,41 Moreover, it is noticed that the intensity of the characteristic peaks of welan gum obviously increases with the loading of 1 mg mL−1 and 5 mg mL−1 GO and the characteristic peaks of CS around 2θ = 11.6, 23.5, and 29.2° can be clearly seen in Fig. 7B. The first peak corresponds to the hydrated crystalline structure, whereas the broadened peak at about 23° indicates the existence of an amorphous structure. Several research results also revealed a similar phenomenon in many polymer/carbon nanotube nanocomposites and it is considered to be a result of polymer crystallization that is induced by carbon nanotubes.42,43 It can be deduced that hydrophobic interaction, electrostatic interaction and hydrogen bonding may contribute to a relatively ordered arrangement of the attached CS chains along the rigid template offered by GO.
The FT-IR and XRD results of the welan gum–GO composite hydrogels with the variation of welan gum concentration when the concentration of GO is fixed at 5 mg mL−1 are shown in Fig. S2.†
DSC analysis
Differential scanning calorimetry (DSC) was carried out to get more information about the glass transition temperature (Tg) for our hydrogels and a phase transition temperature was clearly observed for the hydrogel samples. In Fig. 8A, it can be seen that Tg of the native 8 mg mL−1 welan gum hydrogel is 112 °C; with the incorporation of 1 mg mL−1 GO, Tg increased to 115 °C, and when the concentration of GO was further increased to 5 mg mL−1 GO, Tg changed to 123 °C. These results are consistent with the phase behavior study done above. This is because, with the introduction of GO, there are so many interactions between welan gum and GO, such as hydrogen bonding between the –COOH and –OH groups of welan gum and GO, thus, the increase of crosslinking points greatly restricts the motion of the macromolecular chains of welan gum and results in an increase in the glass transition temperature of welan gum–GO hybrid hydrogels.44 Moreover, if the concentration of GO is fixed at 5 mg mL−1 and with an increase of the concentration of welan gum, Tg is also increased (Fig. 8B).
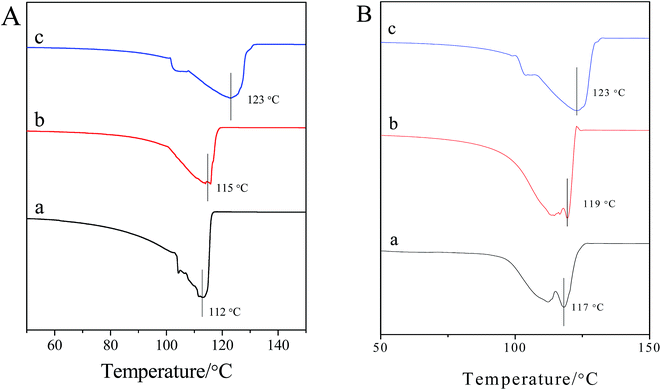 |
| Fig. 8 DSC data of hydrogels of (A): (a) 8 mg mL−1 welan gum, (b) 8 mg mL−1 welan gum/1 mg mL−1 GO, (c) 8 mg mL−1 welan gum/5 mg mL−1 GO; (B): (a) 5 mg mL−1 welan gum/5 mg mL−1 GO, (b) 7 mg mL−1 welan gum/5 mg mL−1 GO, (c) 8 mg mL−1 welan gum/5 mg mL−1 GO. | |
Thermal stability is an imperative parameter for some applications of welan gum–GO hybrid hydrogels and thermogravimetric analysis (TGA) results for pure welan gum and the welan gum/GO nanocomposite are shown in Fig. S3.† It can be seen that pure welan gum decomposes in a one-step process and the welan gum/GO nanocomposite decomposes in a two-step process. The TGA curve of the nanocomposite shifted towards a higher temperature compared to that of pure GO but somewhat lower than that of pure welan gum. Finally, the weight loss of welan gum/GO nanocomposites is between pure welan gum and GO. These results also indicate that the addition of GO at low concentrations somewhat improved the thermal stability of the nanocomposites.
Dye adsorption of welan gum–GO hybrid hydrogels
The obtained hybrid gels present well-defined and interconnected 3D porous networks, which allow the adsorbate molecules to diffuse easily into the adsorbent. Thus, it is expected that the these interconnected 3D porous networks of welan gum–GO hybrid hydrogels could have good dye adsorption capacity. Thus, a variety of water soluble dyes such as MB, MV, MO, AB10B, R6G and CAS were used to study the dye adsorption capacity of the welan gum–GO composite hydrogels. The chemical structures of MB, MV, MO, AB10B, R6G and CAS are shown in the ESI Fig. S4.† Fig. 9 presents the photograph of the adsorption of different dyes at equilibrium for each sample. The adsorption experiments indicate that with the addition of GO, the welan gum–GO composite hydrogels exhibited efficient adsorbability for cationic dyes (MB and MV) and anionic dyes (CAS), a little adsorption effect for AB10B and R6G and nearly no adsorption effect on MO. For the adsorption mechanism, it can be concluded that there are various oxygen-containing functional groups, such as –COOH and –OH on the basal planes and edges of GO. Moreover, the surfaces of the GO sheets are highly negatively charged when dispersed in water which is apparently as a result of the ionization of carboxylic acid and phenolic hydroxyl groups on the GO sheets.45 Thus, the oxygen groups of GO can interact with positively charged dyes such as MB and MV.46,47 Meanwhile, because GO itself also has a huge surface area and graphitized basal plane structure, it allows it to have strong π–π interactions between GO and the aromatic moieties present in the molecules of the dyes which can also increase the adsorption capacity of dyes such as CAS and AB10B.48
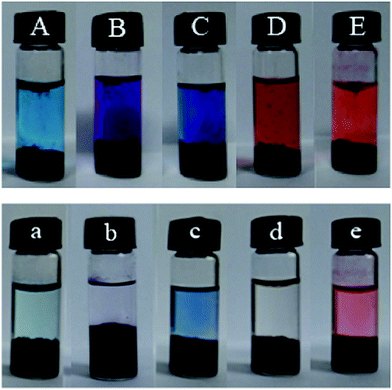 |
| Fig. 9 Photographs of the different dye solutions before (A–E) and after (a–e) adsorption by the welan gum–GO hybrid hydrogels: (A and a) MB, (B and b) MV, (C and c) AB10B, (D and d) CAS, (E and e) R6G. | |
Then, methyl violet (MV), one kind of cationic dye, is chosen as a model adsorbate to investigate the adsorption process. It is clearly seen that the dye molecules of MV were efficiently entrapped in the welan gum–GO hybrid hydrogels and the purple solution became nearly clear after 6 h (Fig. 10A). The concentration variation of MV was monitored using UV-vis spectroscopy (Fig. 10B) and the concentration of MV decreased sharply with time (Fig. 10C).
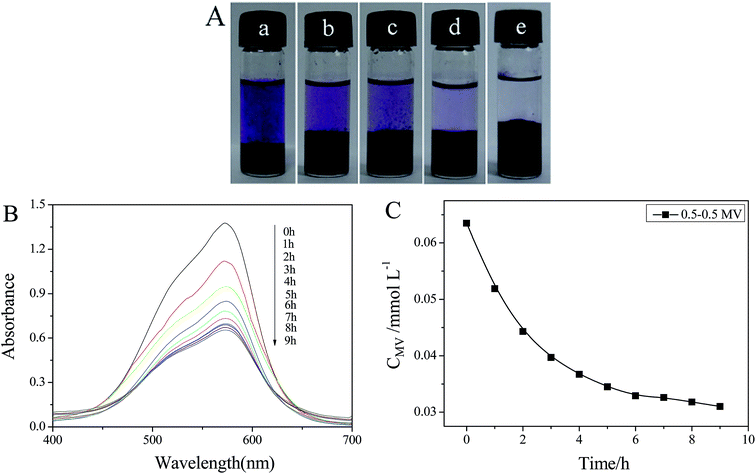 |
| Fig. 10 (A) The adsorption of MV by a welan gum–GO hybrid hydrogel: (a) 0 min, (b) 30 min, (c) 150 min, (d) 240 min. (B) UV-vis spectrum of a dye (MB) solution over time for the samples of the welan gum–GO hybrid hydrogels. (C) UV-vis absorbance of MV solution on top of 5 mg mL−1 welan gum/5 mg mL−1 GO hydrogels as a function of time at 573 nm. | |
Moreover, the effect of the concentration of welan gum or GO on the dye adsorption capacity was also investigated, as shown in Fig. 11 and S5.† After a systematic study, it can be concluded that when the concentration of welan gum was fixed at 5 mg mL−1, the dye adsorption capacity of the welan gum–GO hybrid hydrogels was increased as the concentration of GO increased. However, if the concentration of GO was fixed at 5 mg mL−1, the dye adsorption capacity of the 5 mg mL−1 welan gum/5 mg mL−1 GO hydrogel is higher than that of the 8 mg mL−1 welan gum/5 mg mL−1 GO hydrogel. It indicates that although the strength of the hydrogel is larger, the adsorption efficiency is not better and the best adsorption capacity requires an optimal gel composition.
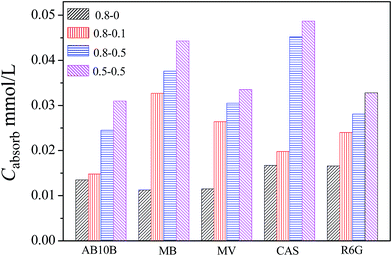 |
| Fig. 11 The adsorption capacity for different dyes by the welan gum–GO hydrogels after 8 h. Each hydrogel (1 g) is applied in 3 mL of 0.05 mmol L−1 dye solution at 25 °C. | |
When an aqueous solution of cationic dye, MV, is mixed together with another different anionic dye, MO, and placed above the welan gum–GO hydrogels, selective removal of the cationic dye, MV, was obviously observed (Fig. 12). These results support the selective removal of the cationic dye by using the constructed gels and this is probably related to the electrostatic interactions between the negatively charged GO sheets, welan gum and the cationic group of MV. In other words, the adsorption experiments indicate that the obtained porous GO–biopolymer gels can efficiently remove cationic dyes from wastewater.
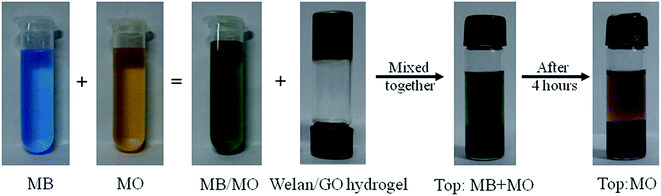 |
| Fig. 12 The selective removal of the cationic dye MV from a mixture of the dyes of MV and MO. | |
4. Conclusions
Biopolymer composite hydrogels were prepared from microbial polysaccharide welan gum as a matrix and GO as a reinforcing additive by a simple self-assembly strategy. Their properties were characterized using a phase behavior study, TEM, AFM, FE-SEM, FT-IR, XRD, DSC and rheological measurements. A uniform distribution and fine dispersion of GO in the welan gum matrix have been demonstrated. The incorporation of GO largely improved the mechanical properties of the welan gum–GO composite hydrogels and they have interconnected 3D porous networks with a highly negative charge and can be used to adsorb dyes efficiently. The results demonstrated that the welan gum–GO composite hydrogels showed good selective adsorbability to cationic dyes. Therefore, the facile and environmental self-assembled microbial polysaccharide welan gum–GO hydrogels could act as promising adsorbents for the removal of dye ions in waste water treatment.
Acknowledgements
We gratefully acknowledge financial support from the National Natural Science Foundation of China (21203109), Ji’nan Youth Science and Technology Star Program (2013040) and China–Australia Centre for Health Sciences Research (CACHSR, No. 2015GJ09).
References
- N. M. Sangeetha and U. Mailra, Chem. Soc. Rev., 2005, 34, 821–836 RSC.
- M. Patenaude, N. M. B. Smeets and T. Hoare, Macromol. Rapid Commun., 2014, 35, 598–617 CrossRef CAS PubMed.
- S. V. Van, P. Dubruel and E. Schacht, Biomacromolecules, 2011, 12, 1387–1408 CrossRef PubMed.
- B. Narayan, G. Jonathan and M. Zhang, Adv. Drug Delivery Rev., 2010, 62, 83–99 CrossRef PubMed.
- K. Y. Lee and D. J. Mooney, Prog. Polym. Sci., 2012, 37, 106–126 CrossRef CAS PubMed.
- W. A. Laftah, S. Hashim and A. N. Ibrahim, Polym.-Plast. Technol. Eng., 2011, 50, 1475–1486 CrossRef CAS PubMed.
- V. K. Thakur and M. K. Thakur, J. Cleaner Prod., 2014, 82, 1–15 CrossRef CAS PubMed.
- V. K. Thakur and M. K. Thakur, Int. J. Biol. Macromol., 2015, 72, 834–847 CrossRef CAS PubMed.
- V. K. Thakur and M. K. Thakur, ACS Sustainable Chem. Eng., 2014, 2, 2637–2652 CrossRef CAS.
- V. K. Thakur and M. R. Kessler, Polymer, 2015, 69, 369–383 CrossRef CAS PubMed.
- V. K. Thakur and M. K. Thakur, Carbohydr. Polym., 2014, 109, 102–117 CrossRef CAS PubMed.
- V. K. Thakur and M. K. Thakur, Int. J. Polym. Anal. Charact., 2014, 19, 256–271 CrossRef CAS PubMed.
- V. K. Thakur and M. K. Thakur, ACS Sustainable Chem. Eng., 2014, 2, 1072–1092 CrossRef CAS.
- V. K. Thakur and M. K. Thakur, Carbohydr. Polym., 2013, 97, 18–25 CrossRef CAS PubMed.
- V. K. Thakur and M. K. Thakur, Carbohydr. Polym., 2013, 98, 820–828 CrossRef CAS PubMed.
- L. Xu, G. Xu, H. Gong, M. Dong, Y. Li and Y. Zhou, Colloids Surf., A, 2014, 456, 176–183 CrossRef CAS PubMed.
- M. Tako, T. Teruya, Y. Tamaki and T. Konishi, Colloid Polym. Sci., 2009, 287, 1445–1454 CAS.
- M. Rinaudo, Biomacromolecules, 2004, 5, 1155–1165 CrossRef CAS PubMed.
- R. Colby, Rheol. Acta, 2010, 49, 425–442 CrossRef CAS.
- M. Sonebi and S. Malinov, ACI Mater. J., 2011, 108, 316–326 CAS.
- H. Itagaki, T. Kurokawa, H. Furukawa, T. Nakajima, Y. Katsumoto and J. P. Gong, Macromolecules, 2010, 43, 9495–9500 CrossRef CAS.
- T. Nakajima, H. Furukawa, Y. Tanaka, T. Kurokawa, Y. Osada and J. P. Gong, Macromolecules, 2009, 42, 2184–2189 CrossRef CAS.
- R. Dash, M. Foston and A. J. Ragauskas, Carbohydr. Polym., 2013, 91, 638–645 CrossRef CAS PubMed.
- W. C. Lin, W. Fan, A. Marcellan, D. Hourdet and C. Creton, Macromolecules, 2010, 43, 2554–2563 CrossRef CAS.
- J. Kim, L. J. Cote, F. Kim, W. Yuan, K. R. Shull and J. Huang, J. Am. Chem. Soc., 2010, 132, 8180–8186 CrossRef CAS PubMed.
- L. J. Cote, F. Kim and J. Huang, J. Am. Chem. Soc., 2009, 131, 1043–1049 CrossRef CAS PubMed.
- X. Li, G. Zhang, X. Bai, X. Sun, X. Wang, E. Wang and H. Dai, Nat. Nanotechnol., 2008, 3, 538–542 CrossRef CAS PubMed.
- S. Sun and P. Wu, J. Mater. Chem., 2011, 21, 4095–4097 RSC.
- L. Zhang, Z. Wang, C. Xu, Y. Li, J. Gao, W. Wang and Y. Liu, J. Mater. Chem., 2011, 21, 10399–10406 RSC.
- Y. Xu, W. Hong, H. Bai, C. Li and G. Shi, Carbon, 2009, 47, 3538–3543 CrossRef CAS PubMed.
- F. Liu, S. Chung, G. Oh and T. S. Seo, ACS Appl. Mater. Interfaces, 2011, 4, 922–927 Search PubMed.
- C. Cheng, J. Deng, B. Lei, A. He, X. Zhang, L. Ma, S. Li and C. Zhao, J. Hazard. Mater., 2013, 263, 467–478 CrossRef CAS PubMed.
- Z. Dong, F. Zhang, D. Wang, X. Liu and J. Jin, J. Solid State Chem., 2015, 224, 88–93 CrossRef CAS PubMed.
- D. Li, M. B. Muller, S. Gilje, R. B. Kaner and G. G. Wallace, Nat. Nanotechnol., 2008, 3, 101–105 CrossRef CAS PubMed.
- J. Shen, G. Xu, X. Xin, L. Wang, Z. Song, H. Zhang, L. Tong and Z. Yang, RSC Adv., 2015, 5, 40173–40182 RSC.
- R. Xue, X. Xin, L. Wang, J. Shen, F. Ji, W. Li, C. Jia and G. Xu, Phys. Chem. Chem. Phys., 2015, 17, 5431–5440 RSC.
- H. Bai, C. Li, X. L. Wang and G. Q. Shi, J. Phys. Chem. C, 2011, 115, 5545–5551 CAS.
- J. Zhang, H. Yang, G. Shen, P. Cheng, J. Zhang and S. Guo, Chem. Commun., 2010, 46, 1112–1114 RSC.
- T. Çaykara, S. Demirci, M. S. Erŏglu and O. Guven, Polymer, 2005, 46, 10750–10757 CrossRef PubMed.
- X. S. Du, M. Xiao, Y. Z. Meng and A. S. Hay, Carbon, 2005, 43, 195–197 CrossRef CAS PubMed.
- T. Jiang, Z. Sui, Q. Yang, X. Zhang and B. Han, Soft Matter, 2015, 11, 3215–3221 RSC.
- O. Probst, E. M. Moore, D. E. Resasco and B. P. Grady, Polymer, 2004, 45, 4437–4443 CrossRef CAS PubMed.
- B. P. Grady, F. Pompeo, R. L. Shambaugh and D. E. Resasco, J. Phys. Chem. B, 2002, 106, 5852–5858 CrossRef CAS.
- J. Fan, Z. Shi, M. Lian, H. Li and J. Yin, J. Mater. Chem. A, 2013, 1, 7433 CAS.
- X. Yang, Y. Tu, L. Li, S. Shang and X. Tao, ACS Appl. Mater. Interfaces, 2010, 2, 1707–1713 CAS.
- N. Zhang, H. Qiu, Y. Si, W. Wang and J. Gao, Carbon, 2011, 49, 827–837 CrossRef CAS PubMed.
- G. L. Li, G. Liu, M. Li, D. Wan, K. G. Neoh and E. T. Kang, J. Phys. Chem. C, 2010, 114, 12742–12748 CAS.
- G. K. Ramesha, A. Vijaya
Kumara, H. B. Muralidhara and S. Sampath, J. Colloid Interface Sci., 2011, 361, 270–277 CrossRef CAS PubMed.
Footnote |
† Electronic supplementary information (ESI) available. See DOI: 10.1039/c5ra12806d |
|
This journal is © The Royal Society of Chemistry 2015 |