DOI:
10.1039/C5RA12577D
(Paper)
RSC Adv., 2015,
5, 72907-72915
Photophysical properties of quinoxaline-fused [7]carbohelicene derivatives†
Received
29th June 2015
, Accepted 13th August 2015
First published on 13th August 2015
Abstract
Helicene and its derivatives have received considerable attention as candidates for organic photoelectronic materials. Recently, novel quinoxaline-fused [7]carbohelicene derivatives have exhibited unique structural and photophysical properties, especially in the crystal state. However, their structure–property relationships have not been fully understood from their micromechanisms, which is also important to further improve their performance. Herein, the electronic transitions, electronic circular dichroism (CD), second-order nonlinear optical (NLO) responses and charge transport properties of five quinoxaline-fused [7]carbohelicene derivatives have been investigated based on density functional theory calculations. The experimental UV-Vis/CD spectra of the studied compounds were reproduced well by our calculations. Thus, we can assign their electron transition properties and absolution configurations (ACs) with high confidence. It is found that the CD bands of quinoxaline-fused [7]carbohelicene derivatives mainly originate from exciton coupling between quinoxaline, phenyl or 4-methoxyphenyl groups and [7]carbohelicene, which is in sharp contrast to [7]carbohelicene. More interestingly, these derivatives possess large first hyperpolarizability values. For example, the βHRS value of compound 6 is 32.96 × 10−30 esu, which is about 190 times larger than that of the organic urea molecule. The bandwidth of the valence band of compound 2 is comparable to that of the conduction band and slightly larger than that of tris(8-hydroxyquinolinato)aluminium. This means that compound 2 is a potential candidate as an ambipolar charge transport material.
1. Introduction
Chirality is not only vital to our life but also plays an important role in material science.1–5 Thus, much effort has been made towards controlling and understanding chirality in chemical synthesis and material design. Helicenes are polycyclic aromatic compounds with screw-shaped skeletons formed by ortho-fused benzene or other aromatic rings.6–9 Steric hindrance interactions between the terminal aromatic rings endow helicenes with helical chirality. As a consequence, helicenes exhibit high optical rotation and circular dichroism values in the visible region.10–17 More interestingly, due to their dissymmetric backbones, helicenes and their derivatives have applications in many fields (e.g. asymmetric catalysis,18–21 liquid crystal molecules,22 enantioselective fluorescent sensors,23 rotors,24 nonlinear optics,25–27 molecular recognition28–31 and organic electronics32–36).
Among the carbohelicene family, [7]carbohelicene is the most interesting member due to its high optical stability (its racemization barrier is 40.5 kcal mol−1),37,38 easy decoration and functionization, and distinguishable photophysical properties.37,39,40 For example, [7]carbohelicene can function as a “molecular tweezer”41–43 of some metallic cations. Fascinating chiroptical properties have been observed by introducing silver(I) ion moieties into [7]carbohelicene.37 Facchetti et al. reported that tetrathia-[7]-helicene derivatives act as p-type semiconductors with high carrier mobilities in organic thin-film transistors.44,45 2,12-dihexyl-2,12-diaza[7]helicene can serve as a deep-blue dopant emitter in an organic light-emitting diode.46 Moreover, [7]carbohelicene can be easily functionalized by varying the substituents. Specifically, the photophysical properties of tetrathia-[7]-helicene can be greatly modulated by decorating the two terminal thiophene rings.47,48 Specifically, novel organometallic Ru(II) and Fe(II) complexes with tetrathia-[7]-helicene have been synthesized. Studies show that tetrathia-[7]-helicene and their Ru(II) and Fe(II) complexes are good candidates for second-order NLO materials.27,49
Quinoxalines (i.e. benzopyrazine) can be easily synthesized from diketones and diamines.50 Quinoxaline has strong electron-withdrawing abilities which originate from the two unsaturated nitrogen atoms in the pyrazine ring. Due to the highly polarized nature of the imine units, quinoxalines are extensively used as light-emitting and electron-transporting materials.51 Moreover, the incorporation of a quinoxaline unit might enable the formation of CH⋯N interactions and influence the crystal packing.52 As a consequence, the quinoxaline units are expected to enhance the luminous efficiency and to control the packing structures in the crystal. Recently, Sakai et al. synthesized a series of quinoxaline-fused [7]carbohelicenes (Fig. 1). It is found that incorporating quinoxaline into [7]carbohelicene greatly enhances the fluorescence quantum yield. Specifically, the fluorescence quantum yield of compound 2 is about four times greater than that of compound 1. Notably, quinoxaline-fused [7]carbohelicene derivatives can possess unique crystal packing. For example, compound 2 possesses racemic crystal packing through intermolecular interactions (π⋯π, CH⋯N and CH⋯π). One-dimensional helical columns were firstly observed which contain the racemic compound 4. This helical columnar structure contains both π–π stacking and CH⋯N interactions, which is in sharp contrast to the packing motif of the corresponding enantiomer. Moreover, the excimer-like delocalized excited state was clearly observed by time-resolved fluorescence measurements.51
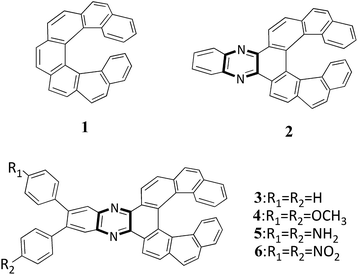 |
| Fig. 1 Chemical structures of the studied compounds 1–6. | |
It is well known that macroscopic properties strongly correlate with microcosmic electron structures, especially for electron transition properties upon excitation. As far as we know, only the frontier molecular orbital levels of quinoxaline-fused [7]carbohelicene derivatives have been studied at the B3LYP/6-31G* level of theory.51 By all appearances, it is necessary to systemically investigate the photophysical properties of these compounds and establish their structure–property relationships at the quantum chemistry level of theory. The main aims of the current investigation were (i) to evaluate the reliability of TDDFT in simulating the UV-Vis/CD spectra of the studied compounds, (ii) to assign their absolute configurations (ACs), (iii) to describe their electronic transition properties and chiroptical origins, and (iv) to investigate their NLO and charge transport properties and determine their potential applications in the materials science field.
2. Computational details
Geometrical optimization of the studied compounds was carried out using the B3LYP53 functional as implemented in the Gaussian 09 computational program suite.54 During the process of optimization, there were no symmetry or internal coordination constraints. The B3LYP functional is a combination of Becke's three-parameter hybrid exchange functional53,55 and the Lee–Yang–Parr56 correlation functional. Basis sets of 6-31G(d,p) for C, O, N, and H atoms were applied. The harmonic vibration calculation was used to confirm their minima.
To investigate the linear and chiroptical properties of the studied compounds, their electron excitation energies, oscillator strengths, and rotational strengths were calculated at the TDB3LYP/6-31+G(d) level of theory. Both length and velocity representations were used to obtain the rotational strengths. It is noted that the velocity-gauge representation of the dipole operator is gauge origin independent. Gaussian bandshapes57 with a bandwidth of 0.12 eV were used to compare the calculated UV-Vis/CD spectra with experimental spectra. The effects of different basis sets and DFT functionals on the UV-Vis/CD spectra were also tested. To test the effect of solvent on the CD spectra, the LR-PCM model58,59 was utilized as implemented in Gaussian 09. The solvent tetrahydrofuran (THF) was treated as a continuous dielectric environment with a dielectric constant of 7.4257.
The first hyperpolarizability was calculated as performed in the Gaussian 09 program package. It is noted that hyper-Rayleigh scattering (HRS) was used to determine the second-order nonlinear optical response (NLO) properties. In the case of plane-polarized incident light and observations made perpendicular to the propagation plane without polarization analysis of the scattered beam, the second-order NLO response that can be extracted from HRS data can be described as:60,61
|
 | (1) |
〈
βZZZ2〉 and 〈
βXZZ2〉 correspond to the orientational average of the
β tensor without assuming Kleinman's conditions.
62 Here, we only were concerned with the static first hyperpolarizability. Therefore, the frequency value in
eqn (1) was set to zero.
To investigate the electronic properties in bulk, an electronic band structure calculation was performed by a DFT method as implemented in the Vienna Ab initio Simulation Package (VASP)63,64 with Perdew–Burke–Ernzerhof (PBE) for the exchange correlation functionals and a plane-wave basis set with an energy cut-off of 400 eV. The Monkhorst–Pack scheme was used to sample the Brillouin zone with a grid spacing of 2π × 0.04 Å−1.
3. Results and discussion
3.1. Molecular structures
In this paper, five quinoxaline-fused [7]carbohelicene derivatives were investigated (Fig. 1). To test the influence of quinoxaline on the electronic properties, [7]carbohelicene was also included. Compounds 2–4 have been synthesized and characterized by X-ray crystallography.51 Compounds 5 and 6 were designed to investigate their charge transfer cooperativity and find effective ways to enhance their NLO response. The geometric structures of the studied compounds were fully optimized without any symmetry constraints at the B3LYP/6-31G(d,p) level of theory. The absence of the imaginary frequencies confirms that our optimized structures are minima. It is noted that the optimized geometries of the studied compounds have C1 symmetry. Here, compound 4 is taken as an example to test our adopted method; its main structural parameters were reproduced well by our calculations (Table S1†). Thus, the adopted basis set and functional are suitable to describe molecular structures for the studied compounds.
3.2. The selection of the basis sets and functionals for the studied compounds
The time-dependent density functional theory (TDDFT) method has extensively been employed to investigate the electronic transition properties65–70 and chiroptical properties71–80 of diverse compounds. In general, electronic transition properties are sensitive to basis sets81–84 and DFT functionals,85–93 especially for CD calculations. For our studied compounds, Sakai et al. only calculated their frontier molecular orbital levels at the B3LYP/6-31G* level.51 The electronic transition properties of these compounds have not been systematically studied thus far. As a consequence, five Pople's basis sets: 6-31G(d), 6-31+G(d), 6-31++G(d,p), 6-311++G(d,p), and 6-311++G(2d,2p) were selected to assess the influence of the basis set extension on the absorption wavelengths using the B3LYP functional, which is the most popular functional for organic compounds. For compound 2, an intense absorption band at 276 nm and three moderately intense absorption bands at 332, 366 and 427 nm were observed.51 The computed absorption wavelengths at the different basis set levels are listed in Table S2.† It is noted that the four observed bands are reproduced well by our calculations. Moreover, the difference between the absorption wavelengths of the largest basis set and the smallest basis set is within 8 nm, which means the effect of the basis set size on the calculated absorption wavelengths is negligible. Previous studies have shown that diffuse functions play a vital role in obtaining accurate absorption wavelengths and describing the electronic transition properties.94 Thus, the 6-31+G(d) basis set was used in the following calculations. Subsequently, five DFT functionals: B3PW91,95–97 M06-2X,98,99 BH&HLYP,100,101 PBE0,102,103 and B3LYP,53 were selected to evaluate the influence of these DFT functionals on the absorption wavelengths. The simulated UV-Vis and CD spectra using these functionals along with the experimental spectra are given in Fig. S1.† The results show that the computed absorption wavelengths strongly depend on the functionals used. Specifically, the M06-2X and BH&HLYP functionals could not reproduce the experimental absorption band at about 427 nm. The same trend was also observed in the simulated CD spectra. This observation might result from the larger energy gaps of the M06-2X and BH&HLYP functionals (Table S3†). However, both the band positions and shapes of the B3LYP, B3PW91 and PBE0 functionals are similar, which might be due to their similar HF exchange fractions. It is well known that conventional TDDFT methods usually underestimate charge-transfer excitations due to semi-local exchange-correlation effects. Studies have shown that range-separated functionals (e.g. LC-BLYP and LC-PBE) can give correct localizations, HOMO–LUMO gaps, and ionization/electron affinity energies. For example, the range-separated functionals have successfully described the optoelectronic and excitonic properties of oligoacenes.104,105 Thus, we also selected the LC-BLYP functional to calculate the electronic excitation properties for compound 2. The experimental spectra of compound 2 were not reproduced well by the LC-BLYP functional (Fig. S1†). Overall, the results computed with the B3LYP functional are much closer to the experimental results. Thus, the B3LYP functional combined with the 6-31+G(d) basis set was employed in the following calculations.
3.3. UV-Vis and CD spectra
In general, the more experimental bands are involved in the theoretical calculation, the more reliable it is for the assignment of the AC.106 Thus, the 60 lowest energies for the studied compounds were calculated at the TDB3LYP/6-31+G(d) level, which covers the range of experimental measurement (Table S4†). The calculated absorption wavelengths, oscillator strengths, and major contributions of the studied compounds, compared to the experimental values, are summarized in Tables 1 and S5.† The simulated UV-Vis/CD spectra are shown in Fig. 2 along with the experimental spectra. Notably, the simulated UV-Vis/CD spectra are in reasonably good agreement with the experimental spectra, not only for the relative peak intensities but also for the band positions. Moreover, the values of the rotational strengths calculated using the length and velocity gauge representation of the electric dipole operator are close to each other (Table S4, ESI†). Thus, our adopted method can reliably describe the electron transition properties and assign the ACs of the quinoxaline-fused [7]carbohelicene derivatives with high confidence. The CD spectra may be sensitive to the solvent.107 The geometries of the studied compounds were re-optimized to test the influence of THF solvent under the PCM model. Next, their solution UV-Vis/CD spectra were also re-calculated based on PCM structures (Fig. S2†). It was found that the shape of the solution UV-Vis/CD spectra are nearly same as the gas phase spectra except for a slightly hypsochromic shift. This indicates that the influence of the solvent is negligible for the studied compounds.
Table 1 Computed absorption wavelengths (λ in nm) compared to experimental data (in parentheses), oscillator strengths (f), and major contributions for compound 2 at the B3LYP/6-31+G(d) level of theory
Band |
λ |
f |
Major contribution |
Band 1 |
282.49(276) |
0.782 |
HOMO−4 → LUMO+2 (42%) |
HOMO → LUMO+4 (14%) |
Band 2 |
316.45(332) |
0.220 |
HOMO−6 → LUMO (77%) |
HOMO−4 → LUMO+2 (9%) |
Band 3 |
346.13(366) |
0.207 |
HOMO → LUMO+2 (37%) |
HOMO−1 → LUMO+1 (22%) |
Band 4 |
424.85(427) |
0.190 |
HOMO−1 → LUMO (85%) |
HOMO → LUMO+2 (12%) |
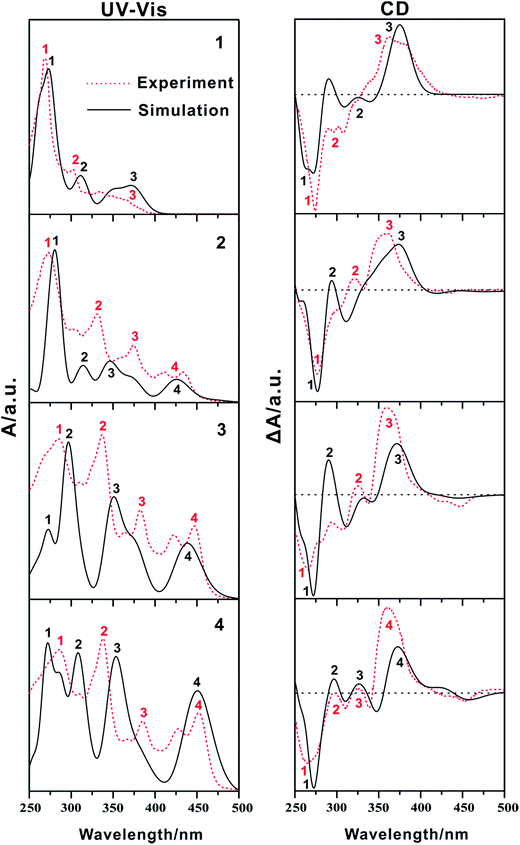 |
| Fig. 2 Calculated UV-Vis (left) and CD (right) spectra in the gas phase of 1, 2, 3 and 4 at the TDB3LYP/6-31+G(d) level of theory along with the experimental spectra (red dashed line). The data to prepare the experimental spectra were taken from ref. 51. | |
The molecular orbitals (MO) involved in the main electronic transitions for the studied compounds 1–4 are shown in Fig. 3 and S3,† respectively. Compound 1 ([7]carbohelicene) exhibits an intense absorption band at 274 nm and two relatively weak absorption bands at 312 and 374 nm. Its main electron transition characteristics can be described as π–π*, as characterized by the fact that these orbitals have π symmetry features. However, compound 2 exhibits four main absorption bands (282, 316, 346, and 425 nm). Notably, the main absorption bands of compound 2 are red-shifted compared with those of compound 1, which is also in agreement with the experimental observations.51 Quinoxaline is an electron–acceptor group.51 In general, the electron–acceptor group mainly modulates the LUMO energy level. As a consequence, the LUMO energy of compound 2 is much lower than that of compound 1 (Table S6†), which leads to the smaller energy gap (Eg) for compound 2. Moreover, the main electron transition characters are charge transfers from quinoxaline to [7]carbohelicene and vice versa (Fig. 3). On the basis of the above analysis, it was found that incorporation of the quinoxaline unit not only modulates the electron absorption wavelength but also alters the electron transition properties. Compounds 3 and 4 also exhibit four absorption bands which are similar to those of compound 2. In other words, the substituent effects of the phenyl or 4-methoxyphenyl groups on the UV-Vis spectra of compound 2 are not great. It is noted that some of the molecular orbitals involved in these transitions are partially located on the phenyl or 4-methoxyphenyl groups.
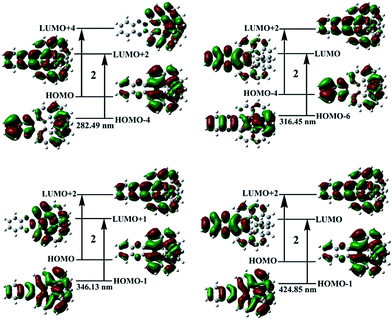 |
| Fig. 3 Molecular orbital isosurfaces involved in the main electron transitions of compound 2 at the TDB3LYP/6-31+G(d) level of theory. | |
Although the CD spectra of quinoxaline-fused [7]carbohelicene derivatives (compounds 2 to 4) are similar to those of [7]carbohelicene (compound 1), there is a slight difference between them. Specifically, compound 1 exhibits a negative Cotton band around 300 nm (labeled as band 2), while this band becomes positive in compounds 2 to 4. This indicates that the CD spectra are sensitive to molecular structures. It should be noted that the calculated relative peak intensities around 300 nm (labeled as band 2) of compounds 1 to 3 are slightly different from the experimental peak intensities. To further understand the chiral origins of the studied compounds, their corresponding molecular orbitals are shown in Fig. S4.† The analysis shows that the chiral origins have a common character for the quinoxaline-fused [7]carbohelicene derivatives (compounds 2 to 4), which can be attributed to the exciton coupling between the quinoxaline, phenyl or 4-methoxyphenyl groups and [7]carbohelicene, which is completely different from those in [7]carbohelicene (Fig. S4†).
3.4. Second-order nonlinear optical response (NLO) properties
Chiral compounds have been viewed as a valuable alternative in the search for new second-order NLO materials due to their intrinsic non-centrosymmetric structures,108 which allows their NLO effects to be observed even in highly symmetric media.108,109 Based on the above electronic transition analysis, quinoxaline-fused [7]carbohelicene derivatives (compounds 2 to 4) exhibit intramolecular charge transfer. Thus, the larger intramolecular charge transfer will come into being under the external electron field and a large NLO response can be observed. Strong electron donor (NH2) or electron acceptor (NO2) groups were also introduced to probe the charge transfer cooperativity and find an effective way to enhance the NLO response. Recently, DFT calculations have emerged as a powerful tool for the investigation and prediction of compounds with large NLO responses.110–115 Thus, the first hyperpolarizability (βHRS) values of the studied compounds were calculated at the CAM-B3LYP/6-31+G(d) level and are given in Table 2. The calculated βHRS values of these studied compounds are larger than those of the typical organic NLO compounds. For example, the calculated βHRS value of compound 6 is about 8 times larger than that of the highly π-delocalized phenyliminomethyl ferrocene complex116,117 and 190 times larger than that of the organic urea molecule.66,118 Thus, the studied compounds could function as excellent second-order NLO materials. The βHRS values for compounds 3 to 5 increase as follows: 3 < 4 < 5, which is agreement with the trend of electron donor ability (H < OCH3 < NH2). However, compound 6 has the largest βHRS value among these compounds. Thus, NO2 as the terminal substitution is an effective way to enhance the NLO response. Electron transition analysis shows that the charge transfer from [7]carbohelicene to the nitrobenzene moieties plays the key role in determining its NLO response. (Fig. S3†). The quinoxaline group in compound 6 acts as a bridge of charge transfer. It is noted that compound 6 has the smallest band gap (Table S6†).
Table 2 The calculated first hyperpolarizability (βHRS) values (10−30 esu) of the studied compounds 3 to 6 at the CAM-B3LYP/6-31+G(d) level of theory
Compound |
βHRS |
3 |
4.43 |
4 |
10.87 |
5 |
20.09 |
6 |
32.96 |
3.5. Charge transport properties
Recently, helicene derivatives have exhibited good and unique charge transport abilities,45,119 which is very important for improving the performance of organic thin-film transistors and organic light emitting diodes. For example, unprecedented carrier inversion has been observed for azaboradibenzo[6]helicene. Its racemate acts as a hole transport material, while the single enantiomer acts as an electron transport material.119 Compounds containing the quinoxaline group exhibit excellent electron transport ability.120 Interestingly, quinoxaline-fused [7]carbohelicene derivatives possess unique crystal packing, as discussed in the introduction. Inspired by those, we investigated their charge transport properties using the band model.121,122 From the viewpoint of the band model, the larger the bandwidth, the larger the carrier mobility.123 It is noted that the spatial distribution of the corresponding wavefunctions is also a factor that influences charge transport properties. Herein, we were mainly concerned with the band model. The calculated band structures of compounds 2 and 4 using the Vienna ab initio simulation package along high-symmetry directions are shown in Fig. 4 and S5.† For compound 2, the bandwidth of the valence band (0.10 eV) is almost equal to that of the conduction band (0.08 eV) and slightly larger than that of tris(8-hydroxyquinolinato)aluminium (AlQ). This finding can also be supported by the frontier molecular orbitals of compound 2. That is, the HOMO is mainly localized on the [7]carbohelicene part, while the LUMO sits on the quinoxaline group (Fig. 3). This is a typical feature for ambipolar charge transport materials.124,125 Thus, compound 2 can act as an ambipolar charge transport material. Compound 4 forms enantiomer and racemate crystals, respectively. It is found that the racemate crystals favour electron transport, while the enantiomer crystals favour ambipolar charge transport. It should be noted that the charge transport ability of compound 4 is much lower than that of compound 1.
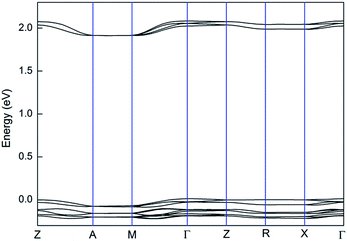 |
| Fig. 4 Calculated band structure of the crystal for compound 2. The high symmetry points are Z = (0, 0, 0.5), A = (0.5, 0.5, 0.5), M = (0.5, 0.5, 0), Γ = (0, 0, 0), Z = (0, 0, 0.5), R = (0, 0.5, 0.5), X = (0, 0.5, 0) and Γ= (0, 0, 0). | |
4. Conclusion
In this paper, the UV-Vis absorption spectra, CD spectra, charge transport properties, and second-order NLO properties of a series of quinoxaline-fused [7]carbohelicene derivatives were systemically investigated using density functional theory. The calculated UV-Vis/CD spectra are in good agreement with the experimental spectra, which can be used to assign the electron transition properties and ACs with high confidence. Exciton coupling between quinoxaline, phenyl or 4-methoxyphenyl groups and [7]carbohelicene are mainly responsible for the chiroptical origin of the quinoxaline-fused [7]carbohelicene derivatives. The charge transport abilities of the studied compounds are sensitive not only to molecular structure but also to crystal packing. Compound 2 can act as an ambipolar charge transport material. In view of their first hyperpolarizability (βHRS) values and intrinsic non-centrosymmetric electronic structures, the studied compounds have the potential to be excellent second-order NLO materials.
Acknowledgements
The authors gratefully acknowledge the financial support from the National Natural Science Foundation of China (21377021) and the Natural Science Foundation of Jilin Province (20140101045JC and 20150101042JC).
Notes and references
- H. Ascolani, M. W. van der Meijden, L. J. Cristina, J. E. Gayone, R. M. Kellogg, J. D. Fuhr and M. Lingenfelder, Chem. Commun., 2014, 50, 13907–13909 RSC.
- A. Shchyrba, M. T. Nguyen, C. Wackerlin, S. Martens, S. Nowakowska, T. Ivas, J. Roose, T. Nijs, S. Boz, M. Schar, M. Stohr, C. A. Pignedoli, C. Thilgen, F. Diederich, D. Passerone and T. A. Jung, J. Am. Chem. Soc., 2013, 135, 15270–15273 CrossRef CAS PubMed.
- T. Balandina, M. W. van der Meijden, O. Ivasenko, D. Cornil, J. Cornil, R. Lazzaroni, R. M. Kellogg and S. de Feyter, Chem. Commun., 2013, 49, 2207–2209 RSC.
- N. Saleh, B. Moore II, M. Srebro, N. Vanthuyne, L. Toupet, J. A. Williams, C. Roussel, K. K. Deol, G. Muller, J. Autschbach and J. Crassous, Chem.–Eur. J., 2015, 21, 1673–1681 CrossRef CAS PubMed.
- J. J. Xie, Y. Y. Duan and S. A. Che, Adv. Funct. Mater., 2012, 22, 3784–3792 CrossRef CAS PubMed.
- J. F. Lamère, I. Malfant, A. Sournia-Saquet, P. G. Lacroix, J. M. Fabre, L. Kaboub, T. Abbaz, A.-K. Gouasmia, I. Asselberghs and K. Clays, Chem. Mater., 2007, 19, 805–815 CrossRef.
- E. Gomar-Nadal, J. Veciana, C. Rovira and D. B. Amabilino, Adv. Mater., 2005, 17, 2095–2098 CrossRef CAS PubMed.
- P. G. Lacroix, Chem. Mater., 2001, 13, 3495–3506 CrossRef CAS.
- A. Bousseksou, G. B. Molnár and G. Matouzenko, Eur. J. Inorg. Chem., 2004, 2004, 4353–4369 CrossRef PubMed.
- M. J. Fuchter, M. Weimar, X. Yang, D. K. Judge and A. J. P. White, Tetrahedron Lett., 2012, 53, 1108–1111 CrossRef CAS PubMed.
- G. M. Upadhyay, H. R. Talele, S. Sahoo and A. V. Bedekar, Tetrahedron Lett., 2014, 55, 5394–5399 CrossRef CAS PubMed.
- F. Aloui, S. Moussa and B. B. Hassine, Tetrahedron Lett., 2012, 53, 3216–3219 CrossRef CAS PubMed.
- Y. Shen, H. Y. Lu and C. F. Chen, Angew. Chem., Int. Ed., 2014, 53, 4648–4651 CrossRef CAS PubMed.
- A. Ueda, H. Wasa, S. Suzuki, K. Okada, K. Sato, T. Takui and Y. Morita, Angew. Chem., Int. Ed., 2012, 51, 6691–6695 CrossRef CAS PubMed.
- S. M. Mel, M. Srebro, E. Anger, N. Vanthuyne, C. Roussel, C. Lescop, J. Autschbach and J. Crassous, Chirality, 2013, 25, 455–465 CrossRef PubMed.
- M. Gingras, Chem. Soc. Rev., 2013, 42, 968–1006 RSC.
- C. Shen, E. Anger, M. Srebro, N. Vanthuyne, K. K. Deol, T. D. Jefferson Jr, G. Muller, J. A. G. Williams, L. Toupet, C. Roussel, J. Autschbach, R. Reau and J. Crassous, Chem. Sci., 2014, 5, 1915–1927 RSC.
- P. Aillard, A. Voituriez and A. Marinetti, Dalton Trans., 2014, 43, 15263–15278 RSC.
- M. J. Narcis and N. Takenaka, Eur. J. Org. Chem., 2014, 2014, 21–34 CrossRef CAS PubMed.
- N. Takenaka, R. S. Sarangthem and B. Captain, Angew. Chem., Int. Ed., 2008, 47, 9708–9710 CrossRef CAS PubMed.
- K. Soai and I. Sato, Chirality, 2002, 14, 548–554 CrossRef CAS PubMed.
- C. Nuckolls and T. J. Katz, J. Am. Chem. Soc., 1998, 120, 9541–9544 CrossRef CAS.
- M. T. Reetz and S. Sostmann, Tetrahedron, 2001, 57, 2515–2520 CrossRef CAS.
- H. Guédouar, F. Aloui, S. Moussa, J. Marrot and B. B. Hassine, Tetrahedron Lett., 2014, 55, 6167–6170 CrossRef PubMed.
- E. Botek, B. Champagne, M. Turki and J. M. Andre, J. Chem. Phys., 2004, 120, 2042–2048 CrossRef CAS PubMed.
- T. Verbiest, S. V. Elshocht, M. Kauranen, L. Hellemans, J. Snauwaert, C. Nuckolls, T. J. Katz and A. Persoons, Science, 1998, 282, 913–915 CrossRef CAS.
- C. A. Daul, I. Ciofini and V. Weber, Int. J. Quantum Chem., 2003, 91, 297–302 CrossRef CAS.
- E. Murguly, R. McDonald and N. R. Branda, Org. Lett., 2000, 2, 3169–3172 CrossRef CAS PubMed.
- Z. An and M. Yamaguchi, Chem. Commun., 2012, 48, 7383–7385 RSC.
- S. Honzawa, H. Okubo, S. Anzai, M. Yamaguchi, K. Tsumoto and I. Kumagai, Bioorg. Med. Chem., 2002, 10, 3213–3218 CrossRef CAS.
- H. Nakagawa, M. Yoshida, Y. Kobori and K.-I. Yamada, Chirality, 2003, 15, 703–708 CrossRef CAS PubMed.
- J. Storch, J. Zadny, T. Strasak, M. Kubala, J. Sykora, M. Dusek, V. Cirkva, P. Matejka, M. Krbal and J. Vacek, Chem.–Eur. J., 2015, 21, 2343–2347 CrossRef CAS PubMed.
- N. Islam and A. H. Pandith, J. Mol. Model., 2014, 20, 2535 CrossRef PubMed.
- R. Hassey, E. J. Swain, N. I. Hammer, D. Venkataraman and M. D. Barnes, Science, 2006, 314, 1437–1439 CrossRef CAS PubMed.
- R. Hassey, K. D. McCarthy, E. Swain, D. Basak, D. Venkataraman and M. D. Barnes, Chirality, 2008, 20, 1039–1046 CrossRef CAS PubMed.
- Y. Zhou, T. Lei, L. Wang, J. Pei, Y. Cao and J. Wang, Adv. Mater., 2010, 22, 1484–1487 CrossRef CAS PubMed.
- M. J. Fuchter, J. Schaefer, D. K. Judge, B. Wardzinski, M. Weimar and I. Krossing, Dalton Trans., 2012, 41, 8238–8241 RSC.
- J. Žádný, P. Velíšek, M. Jakubec, J. Sýkora, V. Církva and J. Storch, Tetrahedron, 2013, 69, 6213–6218 CrossRef PubMed.
- A. Urbano, Angew. Chem., Int. Ed., 2003, 42, 3986–3989 CrossRef CAS PubMed.
- M. C. Carreno, M. G. Lopez and A. Urbano, Chem. Commun., 2005, 611–613 RSC.
- F.-G. Klärner and B. Kahlert, Acc. Chem. Res., 2003, 36, 919–932 CrossRef PubMed.
- T. J. Lahary, D. Jacquemin, B. Legouin, J.-Y. L. Questel, J.-F. Cupif, L. Toupet, P. Uriac and J. Graton, J. Phys. Chem. C, 2015, 119, 3771–3779 Search PubMed.
- M. Lindqvist, K. Borre, K. Axenov, B. Kotai, M. Nieger, M. Leskela, I. Papai and T. Repo, J. Am. Chem. Soc., 2015, 137, 4038–4041 CrossRef CAS PubMed.
- Y. L. Si and G. C. Yang, J. Mater. Chem. C, 2013, 1, 2354–2361 RSC.
- C. Kim, T. J. Marks, A. Facchetti, M. Schiavo, A. Bossi, S. Maiorana, E. Licandro, F. Todescato, S. Toffanin, M. Muccini, C. Graiff and A. Tiripicchio, Org. Electron., 2009, 10, 1511–1520 CrossRef CAS PubMed.
- L. Q. Shi, Z. Liu, G. F. Dong, L. Duan, Y. Qiu, J. Jia, W. Guo, D. Zhao, D. L. Cui and X. T. Tao, Chem.–Eur. J., 2012, 18, 8092–8099 CrossRef CAS PubMed.
- M. Monteforte, S. Cauteruccio, S. Maiorana, T. Benincori, A. Forni, L. Raimondi, C. Graiff, A. Tiripicchio, G. R. Stephenson and E. Licandro, Eur. J. Org. Chem., 2011, 2011, 5649–5658 CrossRef CAS PubMed.
- S. Maiorana, A. Papagni, E. Licandro, R. Annunziata, P. Paravidino, D. Perdicchia, C. Giannini, M. Bencini, K. Clays and A. Persoons, Tetrahedron, 2003, 59, 6481–6488 CrossRef CAS.
- M. H. Garcia, P. Florindo, M. D. F. M. Piedade, S. Maiorana and E. Licandro, Polyhedron, 2009, 28, 621–629 CrossRef CAS PubMed.
- H.-J. Son, W.-S. Han, D.-H. Yoo, K.-T. Min, S.-N. Kwon, J. Ko and S. O. Kang, J. Org. Chem., 2009, 74, 3175–3178 CrossRef CAS PubMed.
- H. Sakai, S. Shinto, Y. Araki, T. Wada, T. Sakanoue, T. Takenobu and T. Hasobe, Chem.–Eur. J., 2014, 20, 10099–10109 CrossRef CAS PubMed.
- K.-T. Lin, H.-M. Kuo, H.-S. Sheu and C. K. Lai, Tetrahedron, 2014, 70, 6457–6466 CrossRef CAS PubMed.
- A. D. Becke, J. Chem. Phys., 1993, 98, 5648 CrossRef CAS PubMed.
- M. Caricato, G. W. Trucks and M. J. Frisch, J. Chem. Theory Comput., 2010, 6, 1966–1970 CrossRef CAS.
- C.-G. Liu, X.-H. Guan and Z.-M. Su, J. Phys. Chem. C, 2011, 115, 6024–6032 CAS.
- S. Grimme and S. D. Peyerimhoff, Chem. Phys., 1996, 204, 411–417 CrossRef CAS.
- P. A. Brooksby and W. R. Fawcett, Spectrochim. Acta, Part A, 2001, 57, 1207–1221 CrossRef CAS.
- C. Bernini, L. Zani, M. Calamante, G. Reginato, A. Mordini, M. Taddei, R. Basosi and A. Sinicropi, J. Chem. Theory Comput., 2014, 10, 3925–3933 CrossRef CAS.
- C. J. Cramer and D. G. Truhlar, Chem. Rev., 1999, 99, 2161–2200 CrossRef CAS PubMed.
- F. Mançois, L. Sanguinet, J.-L. Pozzo, M. Guillaume, B. Champagne, V. Rodriguez, F. Adamietz, L. Ducasse and F. Castet, J. Phys. Chem. B, 2007, 111, 9795–9802 CrossRef PubMed.
- A. Plaquet, M. Guillaume, B. Champagne, F. Castet, L. Ducasse, J.-L. Pozzo and V. Rodriguez, Phys. Chem. Chem. Phys., 2008, 10, 6223–6232 RSC.
- R. Bersohn, J. Chem. Phys., 1966, 45, 3184 CrossRef CAS PubMed.
- G. Kresse and J. Furthmüller, Phys. Rev. B: Condens. Matter Mater. Phys., 1996, 54, 11169–11186 CrossRef CAS.
- G. Kresse and J. Hafner, Phys. Rev. B: Condens. Matter Mater. Phys., 1993, 48, 13115–13118 CrossRef CAS.
- Y. L. Si, G. C. Yang and Z. M. Su, J. Mater. Chem. C, 2013, 1, 1399 RSC.
- Y. L. Si and G. C. Yang, J. Phys. Chem. A, 2014, 118, 1094–1102 CrossRef CAS PubMed.
- Y. M. Sang, L. K. Yan, N. N. Ma, J. P. Wang and Z. M. Su, J. Phys. Chem. A, 2013, 117, 2492–2498 CrossRef CAS PubMed.
- Y. L. Si and G. C. Yang, RSC Adv., 2013, 3, 2241–2247 RSC.
- Y. M. Sang, L. K. Yan, J. P. Wang and Z. M. Su, J. Phys. Chem. A, 2012, 116, 4152–4158 CrossRef CAS PubMed.
- H. Lee, S. Cheon and M. Cho, J. Chem. Phys., 2010, 132, 225102 CrossRef PubMed.
- J. P. Wang, L. K. Yan, G. C. Yang, W. Guan and Z. M. Su, J. Mol. Graphics Modell., 2012, 35, 49–56 CrossRef CAS PubMed.
- I. Valencia, Y. Á. Torres, N. B. Behrens and I. L. Garzón, J. Mol. Struct., 2015, 1085, 52–62 CrossRef CAS PubMed.
- L. Li, B.-B. Yang and Y.-K. Si, Chin. Chem. Lett., 2014, 25, 1586–1590 CrossRef CAS PubMed.
- J. M. Slocik, A. O. Govorov and R. R. Naik, Nano Lett., 2011, 11, 701–705 CrossRef CAS PubMed.
- S. Grimme, J. Harren, A. Sobanski and F. Vögtle, Eur. J. Org. Chem., 1998, 1998, 1491–1509 CrossRef.
- A. Rauk, D. Yang, D. Tsankov, H. Wieser, Y. Koltypin, A. Gedanken and G. V. Shustov, J. Am. Chem. Soc., 1995, 117, 4160–4166 CrossRef CAS.
- G. V. Shustov, A. V. Kachanov, G. K. Kadorkina, R. G. Kostyanovskii and A. Rauk, J. Am. Chem. Soc., 1992, 114, 8257–8262 CrossRef CAS.
- G. V. Shustov, S. V. Varlamov, I. I. Chervin, A. E. Aliev, R. G. Kostyanovskii, D. Kim and A. Rauk, J. Am. Chem. Soc., 1989, 111, 4210–4215 CrossRef CAS.
- A. E. Hansen and T. D. Bouman, J. Am. Chem. Soc., 1985, 107, 4828–4839 CrossRef CAS.
- J. M. Sauvage, M. Morcellet and C. Loucheux, Macromolecules, 1984, 17, 452–456 CrossRef.
- C. R. Legler, N. R. Brown, R. A. Dunbar, M. D. Harness, K. Nguyen, O. Oyewole and W. B. Collier, Spectrochim. Acta, Part A, 2015, 145, 15–24 CrossRef CAS PubMed.
- E. Miliordos and S. S. Xantheas, J. Chem. Phys., 2015, 142, 094311 CrossRef PubMed.
- B. G. Janesko and D. Yaron, J. Chem. Theory Comput., 2005, 1, 267–278 CrossRef CAS.
- G. I. Csonka, A. Ruzsinszky and J. P. Perdew, J. Phys. Chem. B, 2005, 109, 21471–21475 CrossRef CAS PubMed.
- E. A. Bushnell and R. J. Boyd, J. Phys. Chem. A, 2015, 119, 911–918 CrossRef CAS PubMed.
- E. Geidel and F. Billes, J. Mol. Struct.: THEOCHEM, 2000, 507, 75–87 CrossRef CAS.
- N. Ramos-Berdullas, I. Perez-Juste, C. van Alsenoy and M. Mandado, Phys. Chem. Chem. Phys., 2015, 17, 575–587 RSC.
- A. Neugebauer and G. Häfelinger, J. Mol. Struct.: THEOCHEM, 2002, 578, 229–247 CrossRef CAS.
- A. Slimani, X. Yu, A. Muraoka, K. Boukheddaden and K. Yamashita, J. Phys. Chem. A, 2014, 118, 9005–9012 CrossRef CAS PubMed.
- M. D. Wodrich, C. Corminboeuf and P. V. R. Schleyer, Org. Lett., 2006, 8, 3631–3634 CrossRef CAS PubMed.
- C. Lepetit, H. Chermette, M. Gicquel, J.-L. Heully and R. Chauvin, J. Phys. Chem. A, 2007, 111, 136–149 CrossRef CAS PubMed.
- A. Forni, S. Pieraccini, S. Rendine and M. Sironi, J. Comput. Chem., 2014, 35, 386–394 CrossRef CAS PubMed.
- F. Moscardó and A. J. Pérez-Jiménez, Int. J. Quantum Chem., 1998, 67, 143–156 CrossRef.
- D. Jacquemin and C. Adamo, Int. J. Quantum Chem., 2012, 112, 2135–2141 CrossRef CAS.
- R. Gayathri and M. Arivazhagan, Spectrochim. Acta, Part A, 2014, 123, 309–326 CrossRef CAS PubMed.
- D. Mahadevan, S. Periandy and S. Ramalingam, Spectrochim. Acta, Part A, 2011, 84, 86–98 CrossRef CAS PubMed.
- N. X. Wang and A. K. Wilson, J. Phys. Chem. A, 2003, 107, 6720–6724 CrossRef CAS.
- J.-L. Chen, J.-T. Hong, K.-J. Wu and W.-P. Hu, Chem. Phys. Lett., 2009, 468, 307–312 CrossRef CAS PubMed.
- E. G. Hohenstein, S. T. Chill and C. D. Sherrill, J. Chem. Theory Comput., 2008, 4, 1996–2000 CrossRef CAS.
- B. S. Jursic, J. Mol. Struct.: THEOCHEM, 1998, 432, 211–217 CrossRef CAS.
- W.-T. Chan, I. P. Hamilton and H. O. Pritchard, J. Chem. Soc., Faraday Trans., 1998, 94, 2303–2306 RSC.
- R. Orlando, V. Lacivita, R. Bast and K. Ruud, J. Chem. Phys., 2010, 132, 244106 CrossRef PubMed.
- S. Tomić and N. M. Harrison, AIP Conf. Proc., 2010, 1199, 65–66 CrossRef PubMed.
- N. Kuritz, T. Stein, R. Baer and L. Kronik, J. Chem. Theory Comput., 2011, 7, 2408–2415 CrossRef CAS.
- B. M. Wong and T. H. Hsieh, J. Chem. Theory Comput., 2010, 6, 3704–3712 CrossRef CAS PubMed.
- F. Furche and R. Ahlrichs, J. Chem. Phys., 2001, 114, 10362 CrossRef CAS PubMed.
- G. C. Yang, Y. L. Si and Z. M. Su, J. Phys. Chem. A, 2011, 115, 13356–13363 CrossRef CAS PubMed.
- E. Botek, J.-M. André, B. Champagne, T. Verbiest and A. Persoons, J. Chem. Phys., 2005, 122, 234713 CrossRef.
- M. Kauranen, T. Verbiest and A. Persoons, J. Nonlinear Opt. Phys. Mater., 1999, 08, 171–189 CrossRef CAS.
- Y. Zhang and B. Champagne, J. Phys. Chem. C, 2012, 116, 21973–21981 CAS.
- A. Mahmood, M. I. Abdullah and M. F. Nazar, Bull. Korean Chem. Soc., 2014, 35, 1391–1396 CrossRef CAS.
- Y. Z. Li, Y. H. Zhang, D. W. Qi, C. F. Sun and L. P. Yang, J. Mater. Sci.: Mater. Electron., 2014, 25, 5255–5263 CrossRef CAS.
- F. Castet, A. Pic and B. Champagne, Dyes Pigm., 2014, 110, 256–260 CrossRef CAS PubMed.
- L. J. Zhang, D. D. Qi, L. Y. Zhao, C. Chen, Y. Z. Bian and W. J. Li, J. Phys. Chem. A, 2012, 116, 10249–10256 CrossRef CAS PubMed.
- L.-H. Zhang, Y. Wang, F. Ma and C.-G. Liu, J. Organomet. Chem., 2012, 716, 245–251 CrossRef CAS PubMed.
- G. C. Yang, Y. L. Si and Z. M. Su, Org. Biomol. Chem., 2012, 10, 8418–8425 CAS.
- J. S. Chappell, A. N. Bloch, W. A. Bryden, M. Maxfield, T. O. Poehler and D. O. Cowan, J. Am. Chem. Soc., 1981, 103, 2442–2443 CrossRef CAS.
- D. R. Kanis, M. A. Ratner and T. J. Marks, Chem. Rev., 1994, 94, 195–242 CrossRef CAS.
- T. Hatakeyama, S. Hashimoto, T. Oba and M. Nakamura, J. Am. Chem. Soc., 2012, 134, 19600–19603 CrossRef CAS PubMed.
- T. H. Huang, W. T. Whang, J. Y. Shen, Y. S. Wen, J. T. Lin, T. H. Ke, L. Y. Chen and C. C. Wu, Adv. Funct. Mater., 2006, 16, 1449–1456 CrossRef CAS PubMed.
- Y. T. Yang, H. Geng, S. W. Yin, Z. G. Shuai and J. B. Peng, J. Phys. Chem. B, 2006, 110, 3180–3184 CrossRef CAS PubMed.
- J. Widany, G. Daminelli, A. Di Carlo, P. Lugli, G. Jungnickel, M. Elstner and T. Frauenheim, Phys. Rev. B: Condens. Matter Mater. Phys., 2001, 63, 233204 CrossRef.
- M. C. R. Delgado, E.-G. Kim, D. A. D. S. Filho and J.-L. Bredas, J. Am. Chem. Soc., 2010, 132, 3375–3387 CrossRef PubMed.
- C. L. Li, S. P. Wang, W. P. Chen, J. B. Wei, G. C. Yang, K. Q. Ye, Y. Liu and Y. Wang, Chem. Commun., 2015, 51, 10632–10635 RSC.
- G. C. Yang, Y. Liao, Z. M. Su, H. Y. Zhang and Y. Wang, J. Phys. Chem. A, 2006, 110, 8758–8762 CrossRef CAS PubMed.
Footnote |
† Electronic supplementary information (ESI) available: The simulated UV-Vis spectra and CD spectra for the different functionals; a comparison between the calculated and experimentally measured bond lengths; the calculated excitation energies, oscillator and rotational strengths of the 60 lowest energy electronic excitations; molecular orbitals involved into the main electronic transition. See DOI: 10.1039/c5ra12577d |
|
This journal is © The Royal Society of Chemistry 2015 |