DOI:
10.1039/C5RA12256B
(Paper)
RSC Adv., 2015,
5, 67357-67364
Stabilization and optical switching of liquid crystal blue phase doped with azobenzene-based bent-shaped hydrogen-bonded assemblies
Received
25th June 2015
, Accepted 30th July 2015
First published on 31st July 2015
Abstract
We develop a new kind of bent-shaped hydrogen-bonded assemblies (H-bonded assemblies) to stabilize liquid crystal blue phases (LC-BPs) and optically switch the Bragg reflection band of BPs. Here the H-bonded assemblies are simply assembled with the proton acceptor containing azobenzene mesomorphic group and diacid proton donors. The BPs temperature ranges of the LC samples are significantly widened by H-bonded assembly dopants, and the widest BP temperature range is 16.7 °C. Moreover, the lower temperature of BPs is very close to room temperature. Additionally, a blue-shift of the Bragg reflection bands with the corresponding reflection color from green to blue is observed in the LC sample doped with H-bonded assemblies upon UV light irradiation. The related physics mechanisms for the stabilization and optical switching of BPs may be contributing to the bent-shaped molecular geometry of H-bonded assemblies and photoisomerization of azobenzene moiety in H-bonded assemblies, respectively. This work opens up a way to design self-organized molecules suitable for optically tuning BP display and other photonic devices.
1 Introduction
BPs as a series of special liquid crystal (LC) phase, appearing between the chiral nematic (N*) and isotropic phase, have attracted considerable attention recently due to their unique self-assembled cubic structures and intriguing electro-optical characteristics.1,2 Three typical BPs are observed during cooling and reducing of chirality from the isotropic state: BP III, BP II and BP I. Among them, BP I and BP II are believed to be body-centered cubic and simple cubic structure, respectively. While BP III is macroscopically amorphous with a local cubic lattice in the director field.3–8 BP-LCs have the ability of quick response to external fields, which makes BPs fascinating materials for applications such as fast electro-optical devices and tunable photonic crystals.9,10 Unfortunately, BPs usually appear in an extremely narrow temperature range, which is one of their major obstacles to practical application.
During recent years, many methods have been employed to widen the temperature range of BPs.11–18 For example, polymer stabilized BPLCs were first discovered by H. Kikuchi et al. to broaden the temperature of BPs to over 60 °C.19 H. Yoshida et al. extended the BP temperature range from 0.5 to 5 °C by doping a certain amount of spherical Au nanoparticles.20 H. Yang et al. discovered hydrogen bonds (H-bonds) BP-LC consisting of chiral fluorinated alkoxy benzoic acid and isonicotinic acid derivatives, in which the temperature range of BP varied from the ratio of the H-bond precursor.21
In addition to these, BPs with wide temperature ranges have been fabricated by using achiral bent-core (inducing banana-, T-, and U-shaped) molecules. It has been suggested that biaxiality of this kind of molecules has a positive effect of the phases of BPs.22–30 M. Nakata, et al. demonstrated the appearance of BP I and BP II in N*-LCs when they added achiral bent-core molecules into the N*-LCs.22 H. Yang et al. demonstrated a wide range of 25.9 °C BP with bent-shaped molecule doped system based on thiophene as the bent core.31 Our group have developed a series of H-bonded bent-shaped and T-shaped molecules to stabilize BPs,32 in which the widest temperature range is 15.1 °C. Recently, we designed two bent- and dendritic-like polyoxometalate-based organic–inorganic hybrids with covalently grafted azobenzene mesogenic moieties to stabilize BPs.33 The results demonstrate that bent-like POM hybrid and dendritic-like POM hybrid have positive effects for the stabilization of BP I and BP II, respectively. Meanwhile, the photo-tuning of the Bragg reflection band of BP II was demonstrated.
In this work, we develop a novel type of bent-shaped H-bonded assemblies to stabilize BPs by a facile self-assembly method. Here two bent-shaped H-bonded assemblies are fabricated based on the proton acceptor containing azobenzene mesomorphic group and two different diacid proton donors, and the phase behaviors and thermostability of them are characterized. Then we doped them into nematic-LCs to induce BP, the related physics mechanisms for the stabilization of BPs are discussed in terms of a computer-aided molecule simulation and experimental analysis. Moreover, the optically switch the Bragg reflection band of BPs are also investigated. This widening of BP temperature range and optically tuning in Bragg reflections could be valuable in a wide of applications such as BP display and other photonics devices.
2 Experiments
2.1 Materials
The materials we used are as follows: isophthalic acid (IPA) (Alfa Aesar), 4-bromoisophthalic acid (BIPA) (Alfa Aesar) were used as H-donors, 4-{[4-(hexyloxy)phenyl]diazenyl}phenyl isonicotinate (DAIC) was synthesized as described of Fig. 1(a),32,34 and it was used as an H-acceptor. The chemical structures of bent-shaped H-bonded assemblies (IPA–DAIC, BIPA–DAIC) are shown in Fig. 1(b). The N*-LCs were composed of N-LC, SLC1717 (Slichem Liquid Crystal Material Co., Ltd) and chiral dopant, (S)-octan-2-yl 4-(4-hexyloxybenzoyloxy)benzoate, (S811, Bayishikong Liquid Crystal Material Co. Ltd), the chemical structure of S811 is shown in Fig. 1(c).
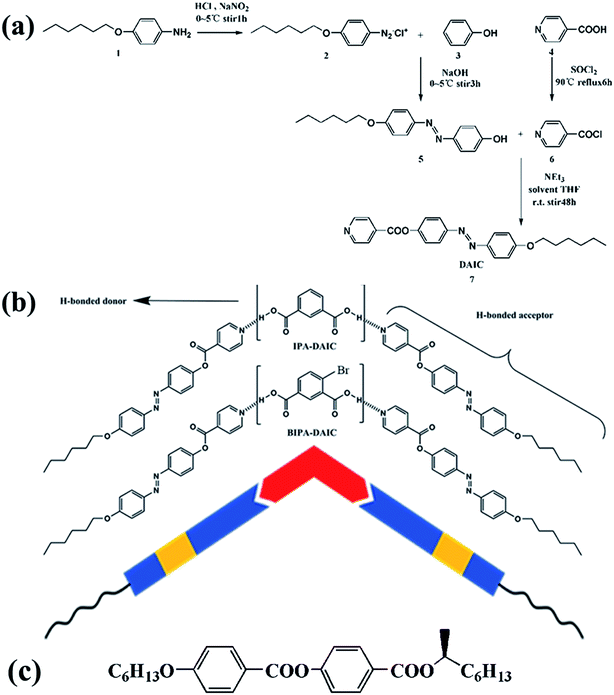 |
| Fig. 1 (a) Synthetic routes to the proton acceptor of bent-shaped H-bonded assemblies; (b) chemical structures of IPA–DAIC, BIPA–DAIC; (c) chemical structure of S811. | |
2.2 Fabrication of H-acceptor
As shown in Fig. 1(a), DAIC was synthesized by esterification reaction similar to our previous study.32 Firstly, intermediate product 4-{[4-(hexyloxy) phenyl]dinitrogen alkenyl}phenol 5 was fabricated by diazotization reaction.34 Secondly, isonicotinoyl chloride 6 was obtained through acylation reaction. Then, compound 5 (0.50 g, 0.0017 mol) and 20 mL THF were mixed into the above solution while stirring continuously, followed by adding triethylamine (2.8 mL, 0.02 mol) to the flask. The solution was kept stirring at room temperature for 48 h, followed by filtered and concentrated in vacuum. The obtained crude product was recrystallized by anhydrous ethanol for three times, an orange powder DAIC was obtained after drying, yield 50%.
1H NMR (400 MHz, CDCl3): δ = 8.973 (s, 2H), 8.324 (s, 2H), 8.028–8.006 (d, J = 8.8 Hz, 2H), 7.960–7.938 (d, J = 8.8 Hz, 2H), 7.423–7.401 (d, J = 8.8 Hz, 2H), 7.053–7.031 (d, J = 8.8 Hz, 2H), 4.098–4.065 (t, J = 6.6 Hz, 2H), 1.8775–1.831 (m, 2H), 1.538–1.500 (m, 2H), 1.394–1.385 (m, 4H), 0.966–0.931 (t, J = 7.0 Hz, 2H).
IR (thin film, frequencies ranging from 400 to 4000 cm−1) νmax: 1739, 1604, 1584, 1497, 1411, 1291, 1251, 1031, 884, 752 and 700 cm−1.
Element contents: N 9.85%, C 70.76%, H 7.112%, O 12.78% respectively, which is in good agreement with the theoretical value.
2.3 Preparation of bent-shaped H-bonded assemblies
Bent-shaped H-bonded assemblies, IPA–DAIC and BIPA–DAIC were prepared by blending the proton acceptor (DAIC) and two proton donors (IPA and BIPA) at molar ratio of 2
:
1 in THF at 60 °C for 20 min respectively, then evaporated under reduced pressure in rotary evaporator immediately, followed by vacuum drying for 12 h.35,36
2.4 Fabrication of BP-LCs doped with bent-shaped H-bonded assemblies
IPA–DAIC and BIPA–DAIC combined with S811 were doped into SLC1717 to induce BPs. Herein, there are two series of LC samples made according to the type and ratio of doped H-bonded assemblies. When 0 wt%, 1.0 wt%, 3.0 wt% and 5.0 wt% of IPA–DAIC H-bonded assemblies were blended into the LC host (67 wt% SLC1717 and 33 wt% S811), they were named samples A0, A1, A2, A3, respectively. And the total mass of each sample remains 0.1 g. To a Laszlo bottle, a mixture of IPA–DAIC, SLC1717 and S811 were added and then dissolved in 5 mL DCM, then the blending proceeded at room temperature for 6 h, no precipitation and/or change of color occurred, followed by vacuum drying for 12 h. While for BIPA–DAIC with the same doped concentration and fabrication procedure, we call them for samples B0, B1, B2 and B3, respectively. Thus the BP-LCs doped with bent-shaped H-bonded assemblies IPA–DAIC and BIPA–DAIC have been prepared.
Glass cells were made from two pieces of glass bonded together, in which a polypropylene film with a thickness of 12.0 μm was used as a cell spacer. Here we note that all the glass cells have no surface alignment layers and also have no alignment treatment. As for different samples, a homogeneous mixture of H-bonded assemblies and N*-LCs in an appropriate weigh ratio was added to each glass cell in an isotropic state and cooled down to BP temperature at a rate of 1.0 °C min−1.
2.5 Measurements
The bent-shaped H-bonded assemblies were characterized using their FT-IR and variable temperature FT-IR spectra. The FT-IR spectra were recorded on a Nicolet 5700 spectrometer at frequencies ranging from 400–4000 cm−1. The H-NMR spectra were recorded on a nuclear magnetic resonance spectrum (Bruker, AV-400). The optical textures of the LC samples were observed by a polarizing light microscope (POM) (Leica DM2500P) with a hot stage calibrated with an accuracy of ±0.1 °C (LTS 420), and the optical images were obtained by Linksys 2.43 software. The Bragg reflection spectra of BPs were examined with AvaSpec-2048 spectrophotometer in reflection mode, and the temperature of the samples was controlled accurately by the hot stage of LTS 420. Besides, UV-Vis spectra of the samples were taken by a JASCO J810 spectrometer. Both the thermo recycle process of H-bonded assemblies was detected by the differential scanning calorimeter (DSC) (TA, Q2000).
3 Results and discussion
3.1 Thermal stability and LC behaviors of bent-shaped H-bonded assemblies
Firstly, the H-bond formation of the bent-shaped H-bonded assemblies of IPA–DAIC and BIPA–DAIC were mainly confirmed using FT-IR spectroscopy as shown in Fig. 2, the formation of H-bonds was detected through the modification of the –OH stretching band of the H-donors. It is well known that typical absorption peaks will appear at around 2500 cm−1 and 1900 cm−1 when H-bonds are formed between carboxyl acid and pyridine group (–OH⋯N). Fig. 2(a) shows the FT-IR spectra of the IPA–DAIC and its precursor. It can be seen that there exists two new peaks at 2490 cm−1 and 1897 cm−1 after self-assembly, which illustrates that H-bonds between the carboxylic acid and pyridyl groups have successfully replaced those of the IPA dimer and the H-bond assembly has been successfully prepared. As shown in Fig. 2(b), a similar phenomenon could be found for the H-bond assembly BIPA–DAIC.
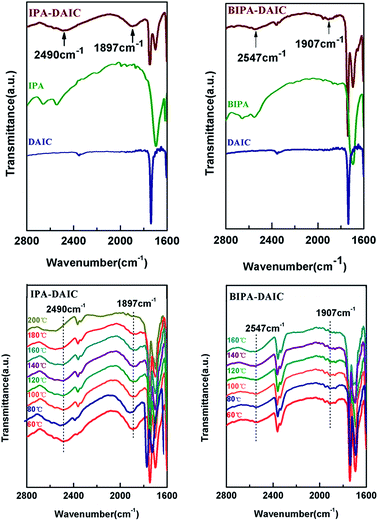 |
| Fig. 2 FT-IR spectra of H-bonded assemblies: (a) IPA–DAIC, (b) BIPA–DAIC; variable temperature FT-IR spectra of H-bonded assemblies: (c) IPA–DAIC, (d) BIPA–DAIC. | |
We further evaluate the thermal stability of the successful assembly of IPA–DAIC and BIPA–DAIC by variable-temperature FI-IR spectra. It is obviously observed that the two characteristic H-bond peaks of IPA–DAIC and BIPA–DAIC near 2500 cm−1 and 1900 cm−1 still existed as the temperature increases until 200 °C and 160 °C in Fig. 2(c) and (d), respectively. All these results indicate that both two bent-shaped H-bonded assemblies have good thermal stabilities.32,35,36 On the other hand, the intensity of the two peaks has a little decrease as the temperature increases in Fig. 2(c) and (d), which means that the H-bonding interaction between the H-donors and the H-acceptor becomes weaker with the increasing temperature.
We further employ POM and DSC measurements to study the LC phase behavior of the bent-shaped H-bonded assemblies. As shown in Fig. 3, both IPA–DAIC and BIPA–DAIC exhibit SmA and N phases on the cooling and heating process. As we can see in Fig. 3(a) and (b), the phase transition temperatures of IPA–DAIC are Cr–SmA, SmA–N and N–I are 165 °C, 175 °C and 180 °C on the heating process, and the corresponding enthalpies of transition are 37.41, 14.52, 1.59 J g−1 respectively. A similar phase sequence of BIPA–DAIC has also been observed in Fig. 3(c) and (d), the phase transition temperatures of BIPA–DAIC are Cr–SmA, SmA–N and N–I are 112 °C, 155 °C and 180 °C on the heating process, and the corresponding enthalpies of transition are 38.64, 13.59, 1.39 J g−1 respectively. The summarized thermal dynamic properties of IPA–DAIC and BIPA–DAIC show that both the bent-shaped H-bonded assemblies possess good LC behaviors.37
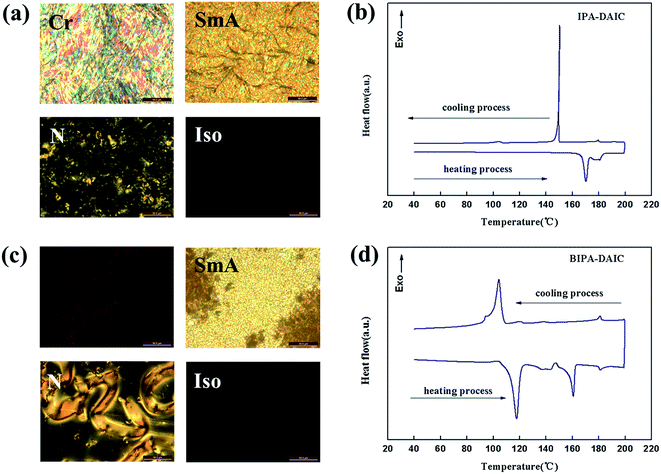 |
| Fig. 3 (a) POM images of IPA–DAIC in different phases; (b) DSC images of IPA–DAIC; (c) POM images of BIPA–DAIC in different phases; (d) DSC images of BIPA–DAIC. | |
3.2 Photo responsive properties of bent-shaped H-bonded assemblies
UV/Vis spectra analysis of H-bonded assemblies in solvent are carried out to investigate the photo responsive of the bent-shaped H-bonded assemblies as shown in Fig. 4. We take the H-bonded assembly of IPA–DAIC as a representative sample shown in Fig. 4(a), there exist two absorption peaks at about 350 nm and 450 nm which are due to a π–π* transition and an n–π* transition, respectively. With the UV exposure time increases, an evident decrease of π–π* transition band and a slight increase of n–π* transition are observed, which shows the trans–cis photoisomerization of IPA–DAIC happens upon UV irradiation. When the exposure time goes to 17 s, the two absorption bands no longer vary, which demonstrates the arrival of photo stationary state. Meanwhile, a similar phenomenon was found in the H-bonded assembly of BIPA–DAIC in Fig. 4(b). To summarize, we confirm that these two bent-shaped H-bonded assemblies exhibit favorable photo responsive properties.
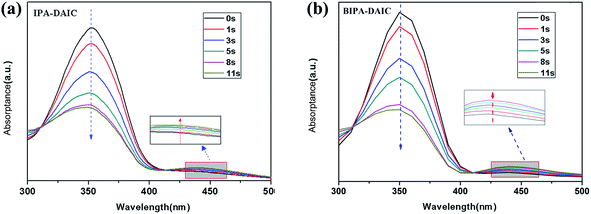 |
| Fig. 4 UV/Vis absorption of bent-shaped H-bonded assemblies in DCM under UV irradiation: (a) IPA–DAIC, (b) BIPA–DAIC. | |
3.3 BP temperature ranges of LC samples doped with bent-shaped H-bonded assemblies
In order to get a more comprehensive understanding of how the bent-shaped H-bonded assemblies affect the temperature range of BPs, we prepared series of LC samples as A0 (B0), A1, A2, A3, B1, B2, B3 as described above. We recorded the LC behaviors of the samples under cooling at 1 °C min−1 at the hot stage by POM, and the BP temperature ranges of the LC samples are summarized in Fig. 5(a). It could be observed that temperature ranges of BP are greatly widened when the LC samples are doped with H-bonded assemblies (both IPA–DAIC and BIPA–DAIC). The LC samples have BP temperature ranges from 6.8 °C to 16.7 °C when the concentrations of H-bonded assemblies range from 1.0 wt% to 5.0 wt%. For example, the LC sample B2 doped with 3.0 wt% BIPA–DAIC exhibits the widest BP I range of about 16.7 °C. What's more, the lower limit of the BPs temperature range is 27.4 °C, which is very close to room temperature, we think it is benefit to the application of BPs in LC displays. Fig. 5(b) shows POM textures of the sample A2 and B2 on the cooling process, we can observe platelet textures of BP I with various colors that are both clear and homogeneous. It should be noted that there's an unusual phase transition behavior in the Bn samples doped with BIPA–DAIC, we think there may be two explanations. First of all, the bent-shaped H-bonded assemblies present well liquid crystalline properties as demonstrated above, which may influence the clearing point of BIPA–DAIC, thus leading to the rise of the transition temperature of N* to BP. Secondly, due to the existence of bromine (Br) in BIPA, the effective compatibility of Bn system doped with BIPA–DAIC may to some extent have influenced as the concentration of BIPA–DAIC increased as a incompatible constituent, which may yield the higher phase transition temperatures. All these two aspects may result in the unusual phase transition behavior of Bn samples doped with BIPA–DAIC.
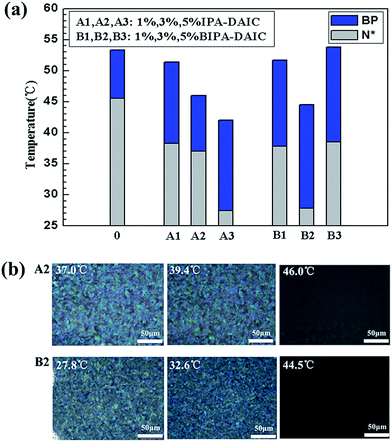 |
| Fig. 5 (a) Temperature ranges of BP-LCs of the samples A0 (B0), A1, A2, A3, B1, B2, B3. (b) POM images of samples A2 and B2 under different temperatures. | |
We further employ the molecular simulation to get the optimized molecular conformation. As shown in Fig. 6, density functional theory (DFT) investigations have been performed on both these two H-bonded assemblies to get more insight of the molecular conformation. Herein, full geometry optimization could be without imposing any constraint using Gaussian 03 program package, and spin-restricted DFT calculations were carried out in the framework of the generalized gradient approximation (CGA) using BeBecke3–Lee–Yang–Parr hybrid functional (B3LYP) exchange–correlation functional and the 6-31G basis set. According to theoretical predictions, it is revealed that bending angles for tans-isomer and cis-isomer of IPA–DAIC is 120.3° and 118.3°, respectively. While bending angles for tans-isomer and cis-isomer of IPA–DAIC is 112.7° and 113.1°, respectively.38–40 These results demonstrate that both IPA–DAIC and BIPA–DAIC are bent-shaped molecular conformations.
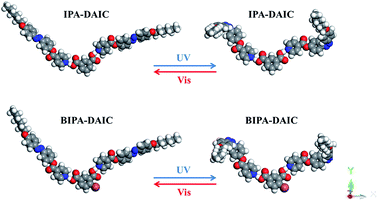 |
| Fig. 6 DFT optimized molecular structure of trans and cis form of IPA–DAIC and BIPA–DAIC using B3LYP hybrid functions at the 6-31G basis set. | |
As is well known, there are two popular ways to stabilize BP or broaden the BP temperature range. One is to increase the chirality of the LC system; the other is to decrease the free energy of the defect core.31 In this work, the stabilization effects may have three explanations. Firstly, the unique achiral bent-shaped molecular conformations of these two H-bonded assembles lead to the increasing of the chirality of the LC system based on the fact that chirality transfer between LC host and bent-core molecules.41 In the N*-LC system, on account of the interactions with the host LC, achiral bent-shaped H-bonded assemblies become structurally tilt and polar order arrangement that induces structurally chirality.22 In this theoretical explanation, the bent shape of H-bonded assemblies is one of the most important factors determining chirality transfer. As a result, this high chirality effect may help to stabilize of BPs as demonstrated in the previous study.42,43 Secondly, the free energy around defects could be decreased by introducing surface elasticity according to defect theory. Here the doped bent-shaped H-bonded assemblies existed in the bulk LC and stabilized the DTC structure of BP I due to their biaxiality from bent molecular shape. Finally, besides facile preparation for H-bonded assemblies, H-bond as a weak bond, in which noncovalent aggregation of H-bonded electrons occurs at the carboxyl and pyridyl positions, could make H-bonded assemblies more compatible in the bulk LCs, this has been suggested to be a favorable effect for the stabilization of BP as mentioned above.32,44 Based on the above analysis, we think that the enhanced chirality and the molecular biaxiality of the bent-shaped H-bonded assemblies could contribute to the stabilization of BP I.
3.4 Optical switching of BPs and the switching mechanism
In order to evaluate the effectiveness of the bent-shaped H-bonded assemblies on the optical tuning of reflection band of BPs, we fabricated sample A4 with 64.0 wt% of SLC 1717 and 36.0 wt% of S811, 1.0 wt% of IPA–DAIC. It is well known that the reflection band will appear a discontinuous jump and shift of approximately 50–80 nm if there is a phase transition from BP II to BP I.45 As is known in Fig. 7(a), we note clearly that the temperature dependence of the reflection wavelength of sample A4 exhibited a continuous and little change with the temperature decreases in BP temperature range. Therefore, considering the relevant observed POM texture, we confirm that there only exits BP I in sample A4. Seen from Fig. 7(b), under UV light irradiation (365 nm, 5 mW cm−2), the reflection bands are discovered to have a slight blue-shift from 575 nm to 551 nm. After 5 s irradiation, the reflection band of BP I no longer shifted and stayed at 551 nm. Fig. 7(c) and (d) show POM images and the corresponding real LC cell images of the sample A4 at 44.5 °C by UV light irradiation, respectively. As the UV irradiation time increases, the BP platelet changing from green color and blue color was observed. When it went to 5 s, the color no longer changed, which is corresponding to the reflection wavelength under UV irradiation as shown in Fig. 7(b). When exposed to visible light, it recovered to the original state due to a restoration of the reflection occurring when cis isomer converts back to trans form under 450 nm light.
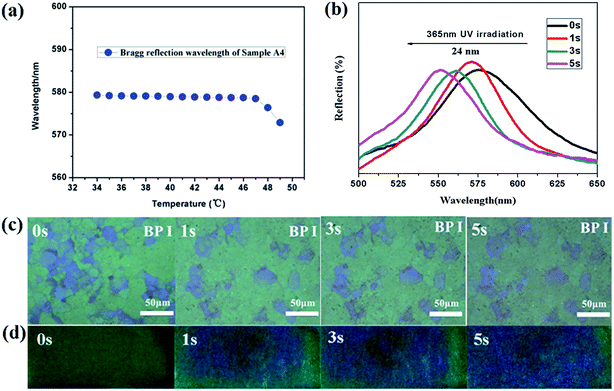 |
| Fig. 7 (a) Reflection wavelength of BP I in sample A4 as a function of temperature with a cooling rate of 1.0 °C min−1; (b) reflection spectra of sample A4 at 44.5 °C under 365 nm light irradiation; (c) POM and (d) the corresponding real images of sample A4 under UV irradiation. | |
In order to estimate the stability and reproducibility of the optical response, the successive “on–off” switching experiment on the reflection band of sample A4 has been investigated. Fig. 8 shows a ten UV-Visible irradiation cycles of reflection wavelength of BP I in sample A4, in each cycle, the reflection wavelength of sample A4 could shift from the original status to its minimum reflection wavelength under UV light irradiation as the red curves demonstrate, and then reversibly return back under visible light as the blue curves show. The above observation demonstrates that sample A4 has a good stability.
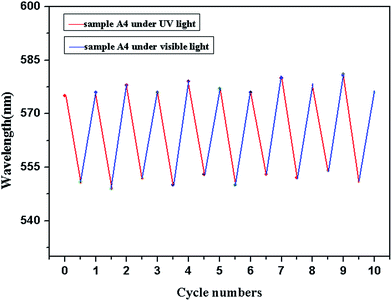 |
| Fig. 8 Ten UV-Visible irradiation cycles of reflection wavelength of BP I in sample A4. | |
Fig. 9 shows the illustrations of BP-LCs doped with bent-shaped H-bonded assemblies and the corresponding phototuning mechanism of double twisted cylinder (DTC) structure before and after light irradiation. As shown in Fig. 6, the theoretical calculations suggest that both IPA–DAIC and BIPA–DAIC exist as bent-shaped structure. Here, the two azobenzene groups which link to the sides of the bent-shaped H-bonded assemblies transform from trans to cis under UV light irradiation, which induces the causes the bending of the rigid part of the H-bonded assemblies as shown in Fig. 9. As a result, this makes LC more compact and results in a decrease of the lattice constant of BP-LCs. Therefore, a blue-shift of the reflection band of BP I happens.46,47 We note that this is different from that of the BP-LC system doped with chiral dopants with azobenzene group.35,48,49 When irradiated by visible light, the reflection band could completely restore since the cis-isomer turns back to the trans configuration. This demonstrates that the phototuning process is reversible by UV/Vis light.
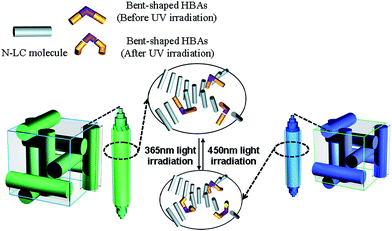 |
| Fig. 9 The possible illustration of bent-shaped H-bonded assemblies doped into the BPs and partial enlargement of the double twist cylinder before and after irradiation. | |
4 Conclusions
In summary, we develop a novel series of bent-shaped H-bonded assemblies of IPA–DAIC and BIPA–DAIC featuring azobenzene groups to stabilize BPs and optically tune the reflection band of BPs. The results demonstrate that the H-bonded assemblies have a positive influence for the stabilization of BPs, in which the widest BP temperature range could reach to around 16.7 °C. On the other hand, the lower limit of the BPs temperature range is very close to the room temperature. Additionally, the stabilization mechanism of the BP should be attributed to bent-shaped the molecular geometry according to the molecular simulations ad experimental analysis. Finally, a reversible photo-switching of BP I of LC sample doped with H-bonded assembly could be achieved due to the configuration changing of the azobenzene from trans to cis by UV/Vis light irradiation, in which a blue-shift of Bragg reflection bands with corresponding reflection color from green to blue could be observed. This work provides a novel idea from molecule design for the stabilization and optically tuning of BP-LCs.
Acknowledgements
This research was supported by National Natural Science foundation (Grant no. 51373013, 50903004) and Beijing Young Talents Plan (YETP0489). We also thank CHEMCLOUDCOMPUTING of Beijing University of Chemical Technology for the molecular simulation of this investigation.
References
- G. W. Gray, J. Chem. Soc., 1956, 3733–3739 RSC.
- G. Nordendorf, A. Hoischen, J. Schmidtke and H.-S. Kitzerow, Polym. Adv. Technol., 2014, 25(11), 1195–1207 CrossRef CAS PubMed.
- N. L. Kramarenko, G. P. Semenkova, V. I. Kulishov, A. S. Tolochko, L. A. Kutulya, V. V. Vaschenko and T. V. Handrimajlova, Liq. Cryst., 1994, 17(3), 351–360 CrossRef CAS PubMed.
- H. Grebel, R. M. Hornreich and S. Shtrikman, Phys. Rev. A, 1983, 28(2), 1114–1138 CrossRef CAS.
- D. K. Yang and P. P. Crooker, Phys. Rev. A, 1987, 35(10), 4419–4423 CrossRef CAS.
- O. Henrich, K. Stratford, M. E. Cates and D. Marenduzzo, Phys. Rev. Lett., 2011, 106(10), 107801 CrossRef CAS.
- R. Memmer, Liq. Cryst., 2000, 27(4), 533–546 CrossRef CAS PubMed.
- K. Higashiguchi, K. Yasui and H. Kikuchi, J. Am. Chem. Soc., 2008, 130(20), 6326–6327 CrossRef CAS PubMed.
- W. Cao, A. Munoz, P. Palffy-Muhpray and B. Taheri, Nat. Mater., 2002, 1(2), 111–113 CrossRef CAS PubMed.
- S. Yokoyama, S. Mashiko, H. Kikuchi, K. Uchida and T. Nagamura, Adv. Mater., 2006, 18(1), 48–51 CrossRef CAS PubMed.
- G. P. Alexander and J. M. Yeomans, Phys. Rev. E: Stat., Nonlinear, Soft Matter Phys., 2006, 74, 061706 CrossRef CAS.
- F. Castles, S. M. Morris, E. M. Terentjev and H. J. Coles, Phys. Rev. Lett., 2010, 104(15), 157801 CrossRef CAS.
- A. Yoshizawa, RSC Adv., 2013, 3(48), 25475–25497 RSC.
- J. Guo, Y. Shi, X. Han, O. Jin, J. Wei and H. Yang, J. Mater. Chem. C, 2013, 1(5), 947–957 RSC.
- A. Yoshizawa, Y. Kogawa, K. Kobayashi and J. Yamamoto, J. Mater. Chem., 2009, 19(32), 5759–5764 RSC.
- K. Kakisaka, H. Higuchi, Y. Okumura and H. Kikuchi, J. Mater. Chem. C, 2014, 2(32), 6467–6470 RSC.
- F. Castles, F. V. Day, S. M. Morris, D.-H. Ko, D. J. Gardiner, M. M. Qasim, S. Nosheen, P. J. W. Hands, S. S. Choi, R. H. Friend and H. J. Coles, Nat. Mater., 2012, 11, 599–603 CrossRef CAS PubMed.
- E. Karatairi, B. Rozic, Z. Kutnjak, V. Tzitzios, G. Nounesis, G. Cordoyiannis, J. Thoen, C. Glorieux and S. Kralj, Phys. Rev. E: Stat., Nonlinear, Soft Matter Phys., 2010, 81, 041703 CrossRef.
- H. Kikuchi, M. Yokota, Y. Hisakado, H. Yang and T. Kajiyama, Nat. Mater., 2002, 1(1), 64–68 CrossRef CAS PubMed.
- H. Yoshida, Y. Tanaka, K. Kawamoto, H. Kubo, T. Tsuda, A. Fujii, S. Kuwabata, H. Kikuchi and M. Ozaki, Appl. Phys. Express, 2009, 2(12), 121501 CrossRef.
- W. He, G. Pan, Z. Yang, D. Zhao, G. Niu, W. Huang, X. Yuan, J. Guo, H. Cao and H. Yang, Adv. Mater., 2009, 21(20), 2050–2053 CrossRef CAS PubMed.
- M. Nakata, Y. Takanishi, J. Watanabe and H. Takezoe, Phys. Rev. E: Stat., Nonlinear, Soft Matter Phys., 2003, 68(4), 041710 CrossRef.
- Z. Zheng, D. Shen and P. Huang, New J. Phys., 2010, 12, 113018 CrossRef.
- K.-W. Park, M.-J. Gim, S. Kim, S.-T. Hur and S.-W. Choi, ACS Appl. Mater. Interfaces, 2013, 5(16), 8025–8029 CAS.
- S. Taushanoff, K. V. le, J. Williams, R. J. Twieg, B. K. Sadashiva, H. Takezoe and A. Jakli, J. Mater. Chem., 2010, 20, 5893–5898 RSC.
- A. Yoshizawa, M. Sato and J. Rokunohe, J. Mater. Chem., 2005, 15, 3285–3290 RSC.
- S.-T. Hur, M.-G. Gim, H.-J. Yoo, S.-W. Choi and H. Takezoe, Soft Matter, 2011, 7, 8800–8803 RSC.
- A. Yoshizawa, M. Kamiyama and T. Hirose, Appl. Phys. Express, 2011, 4, 101701 CrossRef.
- M. Tanaka and A. Yoshizawa, J. Mater. Chem. C, 2013, 1, 315–320 RSC.
- H. J. Coles and M. N. Pivnenko, Nature, 2005, 436, 997–1000 CrossRef CAS PubMed.
- L. Wang, W. He, X. Xiao, Q. Yang, B. Li, P. Yang and H. Yang, J. Mater. Chem., 2012, 22(6), 2383–2386 RSC.
- Y. Shi, X. Wang, J. Wei, H. Yang and J. Guo, Soft Matter, 2013, 9(42), 10186–10195 RSC.
- J. Wang, C. Lin, J. Zhang, J. Wei, Y. Song and J. Guo, J. Mater. Chem. C, 2015, 3(16), 4179–4187 RSC.
- M. Alaasar, M. Prehm, K. May, A. Eremin and C. Tschierske, Adv. Funct. Mater., 2014, 24(12), 1703–1717 CrossRef CAS PubMed.
- O. Jin, D. Fu, J. Wei, H. Yang and J. Guo, RSC Adv., 2014, 4(54), 28597–28600 RSC.
- O. Jin, D. Fu, Y. Ge, J. Wei and J. Guo, New J. Chem., 2015, 39, 254–261 RSC.
- T. Kato and J. M. J. Frechet, J. Am. Chem. Soc., 1989, 111(22), 8533–8534 CrossRef CAS.
- I.-H. Chiang, C.-J. Long, H.-C. Lin, W.-T. Chuang, J.-J. Lee and H.-C. Lin, ACS Appl. Mater. Interfaces, 2014, 6(1), 228–235 CAS.
- C.-L. Wei, T.-C. Chen, P. Raghunath, M.-C. Lin and H.-C. Lin, RSC Adv., 2015, 5(6), 4615–4622 RSC.
- K. Kishikawa, T. Sugiyama, T. Watanabe, S. Aoyagi, M. Kohri, T. Taniguchi, M. Takahashi and S. Kohmoto, J. Phys. Chem. B, 2014, 118(34), 10319–10332 CrossRef CAS PubMed.
- Y. Kogawa and A. Yoshizawa, Liq. Cryst., 2011, 38(3), 303–307 CrossRef CAS PubMed.
- J. Thisayukta, H. Niwano, H. Takezoe and J. Watanabe, J. Am. Chem. Soc., 2002, 124(13), 3354–3358 CrossRef CAS PubMed.
- E. Gorecka, D. Pociecha, M. Cepic, B. Zeks and R. Dabrowski, Phys. Rev. E: Stat., Nonlinear, Soft Matter Phys., 2002, 65(6), 061703 CrossRef CAS.
- L.-Y. Wang, I.-H. Chiang, P.-J. Yang, W.-S. Li, I.-T. Chao and H.-C. Lin, J. Phys. Chem. B, 2009, 113, 14648–14660 CrossRef CAS PubMed.
- H. Choi, H. Higuchi and H. Kikuchi, Appl. Phys. Lett., 2011, 98(13), 131905 CrossRef PubMed.
- X. Chen, L. Wang, C. Li, J. Xiao, H. Ding, X. Liu, X. Zhang, W. He and H. Yang, Chem. Commun., 2013, 49, 10097–10099 RSC.
- Y. Wu, Y. Zhou, L. Yin, G. Zou and Q. Zhang, Liq. Cryst., 2013, 40(6), 726–733 CrossRef CAS PubMed.
- J. B. Guo, J. Wang, J. Y. Zhang, Y. Shi, X. W. Wang and J. Wei, J. Mater. Chem. C, 2014, 2, 9159–9166 RSC.
- T. H. Lin, Y. Li, C. T. Wang, H. C. Jau, C. W. Chen, C. C. Li, H. K. Bisoyi, T. J. Bunning and Q. Li, Adv. Mater., 2013, 25, 5050–5054 CrossRef CAS PubMed.
|
This journal is © The Royal Society of Chemistry 2015 |