DOI:
10.1039/C5RA11901D
(Paper)
RSC Adv., 2015,
5, 79309-79316
Alizarin red S–zinc(II) fluorescent ensemble for selective detection of hydrogen sulphide and assay with an H2S donor†
Received
20th June 2015
, Accepted 7th September 2015
First published on 8th September 2015
Abstract
A new Alizarin Red S based fluorescent probe ARS–Zn(II) has been reported for the detection of H2S in aqueous buffer solution. In the presence of zinc ions, ARS displayed an increase in fluorescence intensity through the formation of an ARS–Zn(II) ensemble. The addition of H2S to the ensemble caused the disassembly of the metal and ARS, which restored the fluorescence intensity of ARS. This sensing ensemble exhibited H2S-selectivity over other biothiols and biologically relevant analytes. The calculated detection limit of ARS–Zn(II) with H2S was 92 nM. ARS–Zn(II) also detected H2S in serum under physiological conditions. Moreover, as a practical application, it could be used for the real time monitoring of H2S released from the H2S-donor molecule benzoic (methyl carbonic) dithioperoxyanhydride and also applied to live cell imaging. The reported ensemble ARS–Zn(II) has advantages like ready availability, high selectivity with good sensitivity, fast response time, higher excitation (520 nm) and emission (625 nm) wavelengths and real time fluorescence assay with H2S donors.
Introduction
Hydrogen sulphide (H2S) is an unpleasant gas with a characteristic smell of rotten eggs. Endogenously produced H2S has been recognized as one of the three gasotransmitters along with nitric oxide (NO) and carbon monoxide (CO).1 Physiological levels of H2S appear to be involved in various biological functions and it is essential for maintaining human health along with having other roles.2 Unusual levels of H2S in humans has been linked to diseases like Alzheimer’s disease,3 Down’s syndrome4 and hypertension.5 The physiological and therapeutic importance of H2S has led to quick progress in research activity involving H2S.6
A number of fluorescent probes for H2S have been developed in the last decade.7–12 However, the selectivity over competing biothiols such as glutathione (GSH), cysteine (Cys) and homocysteine (Hcy), and the sensitivity, response time, multistep synthesis and biocompatibility are some of the serious limitations with the existing fluorescent probes.13–16 These drawbacks show the need for H2S sensors with specific properties such as selectivity, sensitivity, fast response and ready availability, so that they can be widely used to tackle biological issues and for pharmacological applications. To deal with these demanding issues, we wish to report a displacement approach for the development of practically useful fluorescent probes for H2S.
The dye displacement approach is a competitive binding supramolecular sensor system,17–19 where an analyte displaces a dye from a receptor; as a result a colour or fluorescence change is obtained, and can be related to the amount of analyte present.20 In this regard, Alizarin Red-S (ARS) has been successfully employed as an indicator in competitive binding assays for the detection of various analytes such as biological phosphates,21,22 saccharides,23,24 and anions.25 ARS is also known for the colorimetric detection of metal ions.21,26,27 The analyte mediated release of ARS from ARS–copper complex ensembles has been reported for the selective detection of glutathione,28 tiopronin,29 proteins,30 and L-cysteine.31 Similar to copper, ARS is also known to form complexes with the zinc ion with high affinity.32 However ARS–zinc ion based fluorescent ensembles are not well documented for the detection of analytes using the dye displacement method.
Herein, we wish to report a new probe ARS–Zn(II) (Scheme 1) for the selective detection of H2S under biological conditions. ARS shows a ratiometric absorbance change and fluorescence enhancement upon coordination with zinc to form the ARS–Zn(II) ensemble. In the presence of H2S, the emission intensity of ARS was immediately restored as it separates from the sensing ensemble with the formation of ZnS. We have also used this ensemble to monitor H2S released from H2S donor molecules in aqueous solution.
 |
| Scheme 1 Proposed sensing mechanism for the detection of H2S using the ARS–Zn(II) ensemble. | |
Experimental section
Materials and reagent
Methoxycarbonylsulfenyl chloride, thiobenzoic acid, and sodium sulfide were purchased from Sigma-Aldrich. Other chemicals were purchased from Loba Chemie or Merck limited unless otherwise mentioned. The solvents used were analytically pure and used without further purification.
Instrumentation methods
1H NMR was measured on a Bruker Avance 400 MHz. Mass spectra were measured on a Waters Q-Tof mass spectrometer. A Cary Eclipse fluorescence spectrophotometer was used to obtain the fluorescence spectra. UV absorption spectra were recorded on an Evolution 201 UV-Vis spectrophotometer using quartz cells of 1.0 cm pathlength. Live cell images were recorded by using a Nikon Air Laser Scanning Confocal Microscope. Donor studies were performed on a Synergy-HT Multimode Plate reader (BIOTEK). Buffer solutions were prepared by using deionized water and pH studies were done using a HACH sension 2.
General procedures for spectroscopic measurements
Spectral study of ARS with zinc. The absorbance and fluorescence study was performed in a 10 mM MeOH–PBS buffer (3/1 (v/v), pH = 7.4) at RT. For the spectroscopic titrations, 120 μL of the ARS (0.5 mM) stock solution was diluted in 2000 μL by adding buffer. For metal complex formation, the titration was performed with different concentrations of zinc perchlorate (0–30 μM) in buffer. Each spectrum was recorded in a standard quartz cuvette cell of 1 cm in length. The change in absorption was monitored at 530 nm. The emission spectrum was recorded upon excitation at 530 nm with excitation and emission slit widths of 10/10.
Spectral study of ARS–Zn(II) vs. H2S. For the binding study with H2S, 120 μL of ARS (0.5 mM) was mixed with 40 μL of zinc perchlorate (1.5 mM) in buffer and diluted to 2000 μL to form an ARS–Zn(II) complex. The concentrations of [ARS] = 30 μM and [Zn(II)] = 30 μM were maintained for all titrations. UV-Vis and fluorescence titrations were done by adding different concentrations of Na2S (0–44 μM) to this solution. For all the experiments Na2S was used as an H2S gas source.
Fluorescence assay with H2S donor
For the fluorescence assay with H2S donor molecules, 100 μL of ARS–Zn(II) (60 μM) was prepared in a MeOH
:
PBS buffer (3/1, (v/v), 10 mM, pH = 7.4, 0.04% THF used as a co-solvent) and diluted by adding 134 μL of buffer to this. 6 μL of glutathione (0.1 M, 100 equivalents) was added and the fluorescence emission was monitored at 645 nm for 30 minutes at 35 °C. The excitation wavelength was 530 nm and emission wavelength was 645/30 nm. The titration was done in 96 well plates using a Synergy-HT multimode micro plate reader.
Results and discussion
Binding studies of ARS with Zn(II)
Alizarin and ARS are known for their complexation behaviour with different metal ions.32–35 We have studied the complex formation ability of ARS with Zn(II) by using ultraviolet-visible (UV-Vis) and fluorescence spectroscopy in aqueous buffer (MeOH/PBS buffer, 3/1, v/v) at pH 7.4. The UV-Vis spectra resulting from addition of different concentrations of zinc are shown in Fig. 1.
 |
| Fig. 1 Absorption spectra of ARS (30 μM) in MeOH/PBS buffer (3 : 1, v/v, pH = 7.4) in the presence of 0–30 μM equivalents of Zn(II). | |
As shown in Fig. 1, the addition of Zn(II) to ARS induces a ratiometric change with an increase in absorbance at 520 nm, along with a decrease in wavelength at 425 nm and the appearance of an isosbestic point at 465 nm. Based on the UV-Vis spectral change, the binding mode was determined by using a Job’s plot using mixtures of ARS and zinc in buffer solution.
The relative ARS–Zn(II) complex concentration reached a maximum when the molar fraction was 0.33, therefore the Job plot confirmed a 2
:
1 (ARS:Zn(II)) complex formation (SI Fig. 3†), which was similar to the observation of the ARS and Cu2+ coordination stoichiometry as reported earlier.31 At neutral pH one of the phenolic OH groups of ARS is deprotonated and it forms an ARS–Zn(II) complex as shown in Scheme 1.
The ability of ARS to form complexes with zinc ions was also established by using fluorescence measurements. ARS is a natural dye with weak fluorescence properties in aqueous solution under neutral conditions.36
As depicted in Fig. 2, with increasing concentration of Zn(II) ions, the fluorescence intensity in the orange region at 625 nm increased gradually upon excitation at 530 nm, which was mainly due to a combination of the chelation enhanced fluorescence (CHEF) and internal charge transfer (ICT) mechanisms.
 |
| Fig. 2 Fluorescence spectrum of ARS (30 μM) in MeOH/PBS buffer (3 : 1, v/v, pH = 7.4) upon addition of 0–30 μM equivalents of Zn(II). | |
Moreover, it was observed that Zn(II) ions induced almost a 6 fold increase in the fluorescence intensity of ARS. The fluorescence intensity of ARS reached a maximum at 30 μM of zinc, which makes ARS a more sensitive probe for zinc. But as described earlier, when more than 1 equivalent of Zn(II) was added, the fluorescence intensity dropped.32 The association constant (Ka) was evaluated graphically by plotting 1/ΔF against 1/[Zn(II)] as shown in SI Fig. 4†. The data was linearly fitted according to the Benesi–Hildebrand equation and the apparent binding (Ka) value was obtained from the slope and intercept of the line. The Ka value was found to be 13
826 M−1. Zinc ions induced an increase in the fluorescence intensity and the ratiometric change in the UV-Vis absorbance ensured the determination of H2S with high sensitivity with the ARS–Zn(II) sensing ensemble.
Effect of H2S on the optical signal of the ARS–Zn(II) ensemble
H2S is known to form metal sulphides upon reaction with metal ions. Zinc ions are known to react with sulphides to form stable ZnS species,37 which have a low solubility product constant (Ksp = 1.6 × 10−24). So it was expected that the ARS–Zn(II) sensing ensemble would act as a potential sensor for the detection of H2S through the displacement of zinc thereby recovering the original ARS optical (fluorescence and absorbance) signal. Fig. 3a illustrates the emission spectral changes of the probe with H2S at pH 7.4 in aqueous buffer solution. ARS–Zn(II) emitted strong fluorescence at 625 nm, and upon addition of different concentrations of H2S to this solution, the fluorescence intensity decreased. The decrease in fluorescence at 625 nm was achieved immediately (less than a minute) with a fast response time. Real time detection is important for practical applications; consequently the quick response time of the ARS–Zn(II) sensing ensemble is an advantage as compared to that of the DHAQ–Cu2+ ensemble reported earlier for H2S detection, which has a response time of about 4 minutes.38
 |
| Fig. 3 (A) Fluorescence spectra of the ARS–Zn(II) ensemble in MeOH/PBS buffer (3 : 1, v/v, pH = 7.4) upon addition of 0–42 μM equivalents of H2S. (B) Detection limit plot from the fluorescence titration of ARS–Zn(II) with H2S. | |
In addition, the original fluorescence intensity of ARS was almost 100% recovered, when the concentration of H2S was about 42 μM. It confirmed that H2S disassembles the Zn(II) ion and ARS from the sensing ensemble. These results revealed the applicability of the ARS–Zn(II) system for the detection of H2S in aqueous medium. The fluorescence recovery could be used to plot the calibration curve to afford quantitative measurement of H2S in biological samples and H2S releasing drugs.
Based on the change in emission intensity, the detection limit of H2S calculated for the sensing ensemble was 92 nM, which was better than those of other probes reported for H2S, based on the zinc displacement approach.39–41 The detection limit was calculated by the equation 3σ/slope, where the slope was obtained by plotting the change in fluorescence intensity (ΔF) vs. conc. of H2S and σ = the standard deviation of the blank signal obtained without H2S (Fig. 3b).
Furthermore, we have also monitored the UV-Vis absorbance change in the presence of H2S. As shown in Fig. 4, upon addition of H2S to ARS–Zn(II) the absorption band at 525 nm decreased gradually and restored the original absorption state of ARS after adding 44 μM of H2S. It indicates that the dye ARS and Zn(II) have been dissociated from the sensing ensemble in the presence of H2S.
 |
| Fig. 4 Absorption spectra of the ARS–Zn(II) ensemble in MeOH/PBS buffer (3 : 1, v/v, pH = 7.4) upon addition of 0–44 μM of H2S. | |
Hence the results obtained from fluorescence and absorbance spectroscopy confirmed the convenience of using the ARS–Zn(II) sensing ensemble for the detection of H2S in aqueous buffer solution.
Selectivity studies and the effect of interference
The selectivity of the sensing ensemble ARS–Zn(II) for H2S was examined by fluorescence spectroscopy under the same conditions as discussed above. As shown in Fig. 5a, the probe analysed with different biologically important analytes such as reactive sulphur species (glutathione, cysteine, methionine, S2O32−), reactive nitrogen species (NO˙, NO3− and N3−), biological phosphates (ATP, ADP, AMP, PPi, H2PO4− and HPO42−) and other anions (F−, Cl−, Br−, I−, OH−, HCO3− and CH3COO−). Among these biological phosphates (PPi, AMP and H2PO4−), GSH and cysteine displayed little and other analytes exhibited insignificant changes in the fluorescence spectra of ARS–Zn(II) as shown in Fig. 5a. At a higher concentration of cysteine (1 mM) and glutathione (1 mM), notable changes were obtained, but these changes were small compared to those of H2S (30 μM) (SI Fig. 11†).
 |
| Fig. 5 (a) Fluorescence spectra of the ARS–Zn(II) ensemble in the presence of various analytes in MeOH/PBS buffer (3 : 1, v/v, pH = 7.4) at RT upon excitation at 530 nm. (b) Different analyte responses of ARS–Zn(II) in buffer. [ARS] = 30 μM, [Zn(II)] = 30 μM, [anion] = 300 μM, [H2S] = 30 μM. The red colour bar indicates only the probe ARS–Zn(II), the dark blue colour indicates ARS–Zn(II) + anions and the sky blue bars indicate ARS–Zn(II) + anions + H2S. | |
These results confirm the higher selectivity of H2S among other physiological and biologically important analytes. The decrease in the fluorescence intensity with H2S was due to the displacement of zinc and formation of ZnS in aqueous solution. But the non-bonding interaction of glutathione (GSH), cysteine and H2PO4− to the Zn(II) center could be responsible for the weak binding and small change in fluorescence intensity with these analytes.
Furthermore, the selectivity of the probe with H2S was also tested in the presence of interfering species. As shown in Fig. 5b. The competitive experiments revealed minimal interference or no interference with the detection in the coexistence of various species and H2S. Accordingly, probe ARS–Zn(II) could be applicable for the selective determination of H2S even in the presence of other biological species like ATP, ADP, AMP, PPi, GSH and cysteine. This result also confirmed the possible use of the probe even when H2S co-exists with other biological relevant biothiols like glutathione, cysteine, methionine and phosphates.
Effect of pH
The maximum fluorescence of ARS is greatly affected by the pH of the solution.42 The influence of the pH on ARS–Zn(II) was studied using fluorescence measurements. The nature of the fluorescence profile shown in Fig. 6 could be a result of the stability of the ARS–Zn(II) sensing ensemble. At pH values less than 6, the competition between the OH-proton and the metal ion could occur. Therefore, the ARS–Zn(II) complex was less stable, which prevented H2S detection. Therefore, the emission intensity does not change so much with H2S at pH less than 6. Due to the high concentration of hydroxide ions, at a higher pH (pH > 9.0) it is expected that Zn(II) ions may precipitate and consequently, at higher pH the sensing ensembles would not be stable, therefore it may not be suitable for binding studies. These results described that the detection of H2S using probe ARS–Zn(II) was pH dependent and the maximum change in emission intensity with H2S was obtained in the pH range of 6.0–8.0.
 |
| Fig. 6 Effect of the pH on the fluorescence intensity of the ARS–Zn(II) ensemble and the ensemble with H2S. [ARS] = 27 μM, [Zn(II)] = 27 μM, [H2S] = 27 μM in MeOH/PBS buffer (3 : 1, v/v, pH = 7.4). | |
Recycling sensing performance
The reusability of any sensor is very important for practical application, which requires that the sensor device should be reversible. Therefore, the recycling sensing performance of ARS–Zn(II) was studied through disassembly and reassembly of ARS by H2S and zinc.
The reversibility study was carried out by monitoring the change in fluorescence of the ARS–Zn(II) ensemble in a buffered solution. The fluorescence intensity was checked after displacing Zn(II) with H2S (42 μM) and again, adding Zn(II) (30 μM) to ARS (30 μM). As shown in Fig. 7 even after 5 cycles, the change in fluorescence or decrease in fluorescence for the ARS–Zn(II) solution with H2S was still relatively good. Thus, switching between the fluorescence OFF–ON states could be repeated more than five times without decomposition of the fluorescence indicator ARS dye. Therefore, these results showing the sensor’s high reversibility and favour real-life applications.
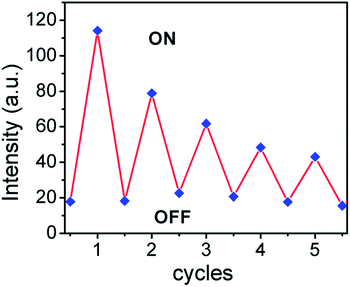 |
| Fig. 7 Fluorescence intensity of the ARS–Zn(II) ensemble upon alternate addition of H2S and Zn(II) [ARS] = 30 μM, [Zn(II)] = 30 μM, [H2S] = 42 μM. | |
Fluorescence study of ARS–Zn(II) with H2S in serum
After confirming the sensitivity and selectivity of the probe in buffer solution, we have decided to explore the practical application of the probe for the quantization of H2S, in human serum (HS) and bovine serum albumin (BSA).
As shown in Fig. 8, the background fluorescence of ARS–Zn(II) with blank HS and BSA did not change much. However, upon addition of H2S spiked (6–30 μM) HS or BSA, the fluorescence intensity of the probe ARS–Zn(II) changed significantly. A linear calibration curve can be found between the change in fluorescence intensity at 620 nm and the concentration of H2S. The change in fluorescence intensity at 620 nm increased in a linear fashion with a higher percentage of H2S in the serum solutions (SI Fig. 12†). This calibration curve could be useful for estimating the concentration of H2S in biological fluids. These results confirmed that probe ARS–Zn(II) can be used to detect H2S rapidly and quantitatively in biological samples.
 |
| Fig. 8 Fluorescence intensity of the ARS–Zn(II) ensemble upon addition of H2S spiked (6 μM, 12 μM, 18 μM, 24 μM, and 30 μM) buffer, human serum and BSA. | |
Real time monitoring of H2S from donor molecules
Recently, the development of novel H2S donors has become a rapidly growing field, because synthetic H2S donors are useful research tools as well as potential therapeutic agents.6,43 These donor molecules release H2S via different mechanisms with different rates. Therefore it is important to understand the rate of H2S release from donor molecules. Various methods have been used to determine the concentration of H2S released by these donor molecules. Fluorescence assaying is one such promising method; in this regard researchers are always looking for better probes that use fluorescence techniques to measure and monitor the release of H2S from donors. The present probe ARS–Zn(II) has high selectivity, sensitivity and quick response time for the detection of H2S in biological conditions. Thus, we have decided to make use of this new probe to monitor the H2S release from a donor molecule (Scheme 2).
 |
| Scheme 2 Sensing mechanism for the detection of H2S released from an H2S donor molecule. | |
In this regard, we have prepared the H2S donor benzoic (methyl carbonic) dithioperoxyanhydride as described in the literature.44
Our aim was to examine the feasibility of using ARS–Zn(II) to monitor in situ the production of H2S from donor molecules. In a typical experimental setup, the sensing ensemble ARS–Zn(II) (1 mM, 100 μL of the stock solution) in phosphate buffer (134 μL) was mixed with GSH (0.1 M, 6 μL of the stock solution). Then, the H2S donor (1 mM, 60 μL of the stock solution in THF) was added just before starting the fluorescence measurement. The time dependent fluorescence measurement was performed using a Synergy HT micro plate reader at 530/645 nm as the excitation/emission wavelength for a 30 minute period of time.
As shown in Fig. 9, ARS–Zn(II) could determine the H2S release associated with the decomposition of the donor in the presence of GSH by using a multimode micro plate reader. The amount of H2S released was expressed as a relative fluorescence unit (F/F0). The fluorescence assay showed that the spontaneous release of H2S by donor molecules was easily monitored by the new ARS–Zn(II) ensemble probe based on fluorescence changes.
 |
| Fig. 9 H2S release monitored by the ARS–Zn(II) (2 μM) ensemble with H2S donor (20 μM) and GSH (200 μM) at 35 °C. | |
We have also assessed H2S release assays in a temperature-dependent experiment by varying the assay temperature from 30 to 50 °C (SI Fig. 13†). We found that the rate of the H2S release from the donor was slightly faster at higher temperatures; it confirmed the stability of the probe at higher temperatures. Hence, the results demonstrated that the H2S release from donor molecules can be monitored by probe ARS–Zn(II) in real time.
The spectroscopic properties of ARS–Zn(II) and its selectivity for H2S appeared to be suitable for live cell image studies. Thus we have used cultured C6 cell lines to investigate the potential application of ARS–Zn(II) for the detection of H2S in biological cells. As shown in Fig. 10, C6 cells images were recorded after incubation with compound ARS for 30 min, and we did not observe any fluorescence signal. But strong fluorescence in the cells was induced after the addition of zinc and the fluorescence disappeared upon treatment with H2S. This result proves that probe ARS–Zn(II) can be used for the detection of H2S in cultured cells.
 |
| Fig. 10 Fluorescence images of C6 cell lines. (a) DIC image of C6 cells treated with ARS (30 μM) only. (b) Fluorescence image of cells in the red channel treated with ARS (30 μM) for 20 min at 37 °C. (c) DIC image of cells treated with ARS (30 μM) and then treated with 30 μM of Zn(II) ions. (d) Fluorescence image of cells in the red channel upon treatment with 30 μM of ARS and then 30 μM Zn(II) ions for 20 min at 37 °C. (e) DIC image of cells treated with 30 μM of ARS and then treated with 30 μM of Zn(II) ions followed by the addition of Na2S. (f) Fluorescence images of cells in the red channel upon treatment with 30 μM of ARS and then treated with 30 μM of Zn(II) ions followed by the addition of 44 μM of Na2S for 20 min at 37 °C. Images were taken at λex = 543 nm with a 40× objective. | |
Table 1 List of zinc(II) based probes for the detection of H2S available in the literaturea
Sensor probe |
LOD |
Medium |
Detection time |
Reference |
LOD = limit of detection, ND = not determined. |
8-Aminoquinoline based Zn-complex |
1.2 μM |
CH3CN–Tris–HCl |
2 min |
40 |
7-Mercapto-4-methylcoumarin based Zn-complex |
1 μM |
Acetone–HEPES |
ND |
39 |
4-Methyl-2,6-diformyl phenol based Zn-complex |
μM level |
Ethanol–Tris–HCl |
ND |
41 |
ARS–Zn(II) |
92 nM |
Methanol–PBS |
<1 min |
Present work |
Conclusions
In summary, we have reported an Alizarin Red S based fluorescent sensing ensemble ARS–Zn(II) for the selective detection of H2S in aqueous solution. The probe displayed good selectivity and remarkable sensitivity. Compared to other reported probes based on zinc complexes for the detection of H2S (Table 1), we see that the present probe has a number of advantages as listed below: (a) Probe ARS–Zn(II) is commercially available and very cheap, therefore this strategy eliminated the need for multi-step synthesis, purification and characterization by sophisticated analytical instrumentations, (b) longer excitation (λexc = 530 nm) and emission (λem = 625 nm) wavelengths are more suitable for biological purposes, (c) fast response (<30 seconds) time with H2S, (d) high detection limit (92 nM), (e) the reversible ON–OFF–ON fluorescence change mode with H2S and zinc makes ARS–Zn(II) a recyclable probe for H2S, (f) suitable for real time detection of H2S released from H2S donor molecules. In addition, the fluorescence response with H2S is also applied in live cell imaging. We anticipate that sensing ensemble ARS–Zn(II) based fluorescence assays will be used in future as a powerful tool to monitor the release of H2S from biological and pharmacological samples.
Acknowledgements
We acknowledge the Director and Head of the Chemistry Department, NIT Kurukshetra, Haryana for the research facilities. The authors also thank Prof. Manoj Kumar for his support in live cell images study. DAJ acknowledges the financial support of the Department of Science and Technology (DST), India, for the SERB-DST project grant SB/FT/CS-195/2013. AG is thankful to the financial support of the DST, India, for the SERB-DST young scientist research project grants (SB/FT/CS-193/2013).
Notes and references
- H. Kimura, Mol. Neurobiol., 2002, 26, 13–19 CrossRef CAS PubMed.
- R. Wang, Physiol. Rev., 2012, 92, 791–896 CrossRef CAS PubMed.
- L. E. Rojo, J. A. Fernández, A. A. Maccioni, J. M. Jimenez and R. B. Maccioni, Arch. Med. Res., 2008, 39, 1–16 CrossRef CAS PubMed.
- P. Kamoun, Med. Hypotheses, 2001, 57, 389–392 CrossRef CAS PubMed.
- G. Yang, L. Wu, S. Bryan, N. Khaper, S. Mani and R. Wang, Cardiovasc. Res., 2010, 86, 487–495 CrossRef CAS PubMed.
- J. L. Wallace and R. Wang, Nat. Rev. Drug Discovery, 2015, 14, 329–345 CrossRef CAS PubMed.
- V. S. Lin and C. J. Chang, Curr. Opin. Chem. Biol., 2012, 16, 595–601 CrossRef CAS PubMed.
- M. Strianese, M. Staiano, G. Ruggiero, T. Labella, C. Pellecchia and S. D’Auria, Methods Mol. Biol., 2012, 875, 193–216 CAS.
- C.-X. Duan and Y.-G. Liu, Curr. Med. Chem., 2013, 20, 2929–2937 CrossRef CAS.
- A. R. Lippert, J. Inorg. Biochem., 2014, 133, 136–142 CrossRef CAS PubMed.
- J. Li, C. Yin and F. Huo, RSC Adv., 2015, 5, 2191–2206 RSC.
- N. Kumar, V. Bhalla and M. Kumar, Coord. Chem. Rev., 2013, 257, 2335–2347 CrossRef CAS PubMed.
- H. S. Jung, X. Chen, J. S. Kim and J. Yoon, Chem. Soc. Rev., 2013, 42, 6019–6031 RSC.
- K. Wang, H. Peng and B. Wang, J. Cell. Biochem., 2014, 115, 1007–1022 CrossRef CAS PubMed.
- Z. Guo, G. Chen, G. Zeng, Z. Li, A. Chen, J. Wang and L. Jiang, Analyst, 2015, 140, 1772–1786 RSC.
- H. Peng, W. Chen, S. Burroughs and B. Wang, Curr. Org. Chem., 2013, 17, 641–653 CrossRef CAS.
- D. A. Jose, M. Elstner and A. Schiller, Chem.–Eur. J., 2013, 19, 14451–14457 CrossRef CAS PubMed.
- X. Lou, D. Ou, Q. Li and Z. Li, Chem. Commun., 2012, 48, 8462–8477 RSC.
- G. Ghale and W. M. Nau, Acc. Chem. Res., 2014, 47, 2150–2159 CrossRef CAS PubMed.
- B. T. Nguyen and E. V. Anslyn, Coord. Chem. Rev., 2006, 250, 3118–3127 CrossRef CAS PubMed.
- R. Villamil-Ramos and A. K. Yatsimirsky, Chem. Commun., 2011, 47, 2694–2696 RSC.
- A. Nonaka, S. Horie, T. D. James and Y. Kubo, Org. Biomol. Chem., 2008, 6, 3621–3625 CAS.
- K. K. Ghosh, E. Yap, H. Kim, J.-S. Lee and Y.-T. Chang, Chem. Commun., 2011, 47, 4001–4003 RSC.
- W. M. J. Ma, M. P. Pereira Morais, F. D’Hooge, J. M. H. van den Elsen, J. P. L. Cox, T. D. James and J. S. Fossey, Chem. Commun., 2009, 532–534 RSC.
- Y. Kubo, T. Ishida, A. Kobayashi and T. D. James, J. Mater. Chem., 2005, 15, 2889–2895 RSC.
- A. Safavi and M. Bagheri, Anal. Chim. Acta, 2005, 530, 55–60 CrossRef CAS PubMed.
- A. Abbaspour and L. Baramakeh, Sens. Actuators, B, 2006, 114, 950–956 CrossRef CAS PubMed.
- Z. Chen, Z. Wang, J. Chen and X. Chen, Biosens. Bioelectron., 2012, 38, 202–208 CrossRef CAS PubMed.
- Z. Chen, Z. Wang, J. Chen and W. Gao, Talanta, 2012, 99, 774–779 CrossRef CAS PubMed.
- X. Yu and C. Mou, Anal. Lett., 2011, 44, 137–145 CrossRef CAS PubMed.
- F.-Y. Wu, W.-S. Liao, Y.-M. Wu and X.-F. Wan, Spectrosc. Lett., 2008, 41, 393–398 CrossRef CAS PubMed.
- L. Zhang, S. Dong and L. Zhu, Chem. Commun., 2007, 1891–1893 RSC.
- H. Kunkely and A. Vogler, Inorg. Chem. Commun., 2007, 10, 355–357 CrossRef CAS PubMed.
- M. Doskocz, K. Kubas, A. Frąckowiak and R. Gancarz, Polyhedron, 2009, 28, 2201–2205 CrossRef CAS PubMed.
- S. Sinha, P. Gaur, T. Mukherjee, S. Mukhopadhyay and S. Ghosh, J. Photochem. Photobiol., B, 2015, 148, 181–187 CrossRef CAS PubMed.
- N. S. Allen and J. F. McKellar, J. Photochem., 1976, 5, 317–320 CrossRef CAS.
- P. Wu, J. Zhang, S. Wang, A. Zhu and X. Hou, Chem.–Eur. J., 2014, 20, 952–956 CrossRef CAS PubMed.
- Y. Wang, H. Sun, L. Hou, Z. Shang, Z. Dong and W. Jin, Anal. Methods, 2013, 5, 5493–5500 RSC.
- E. Galardon, A. Tomas, P. Roussel and I. Artaud, Dalton Trans., 2009, 9126–9130 RSC.
- Z. Dong, X. le, P. Zhou, C. Dong and J. Ma, New J. Chem., 2014, 38, 1802–1808 RSC.
- Z. Dong, X. le, P. Zhou, C. Dong and J. Ma, RSC Adv., 2014, 4, 18270–18277 RSC.
- G. Springsteen and B. Wang, Chem. Commun., 2001, 1608–1609 RSC.
- Z. J. Song, M. Y. Ng, Z.-W. Lee, W. Dai, T. Hagen, P. K. Moore, D. Huang, L.-W. Deng and C.-H. Tan, MedChemComm, 2014, 5, 557–570 RSC.
- T. Roger, F. Raynaud, F. Bouillaud, C. Ransy, S. Simonet, C. Crespo, M.-P. Bourguignon, N. Villeneuve, J.-P. Vilaine, I. Artaud and E. Galardon, ChemBioChem, 2013, 14, 2268–2271 CrossRef CAS PubMed.
Footnote |
† Electronic supplementary information (ESI) available: Synthesis of the H2S donor, experimental methods and some UV-Vis and fluorescence spectra are available. See DOI: 10.1039/c5ra11901d |
|
This journal is © The Royal Society of Chemistry 2015 |