DOI:
10.1039/C5RA11561B
(Paper)
RSC Adv., 2015,
5, 67418-67426
Application of magnetic nanoparticles modified with poly(2-amino thiophenol) as a sorbent for solid phase extraction and trace detection of lead, copper and silver ions in food matrices†
Received
16th June 2015
, Accepted 21st July 2015
First published on 22nd July 2015
Abstract
We are introducing magnetic nanoparticles modified with poly(2-amino thiophenol) as a new solid-phase for the extraction of heavy metals ions, including lead(II), copper(II) and silver(I). The synthesized sorbent was characterized by Fourier transform infrared spectrometry, elemental analysis, scanning electron microscopy, energy-dispersive X-ray spectrometry, thermogravimetric analysis and X-ray diffraction analysis. Separation of the synthesized sorbent from the sample solution was simply achieved by applying an external magnetic field. Determination of the extracted ions was performed by flame atomic absorption spectrophotometry (FAAS). Effects of pH value, adsorption and desorption time, type, concentration and volume of the eluent, breakthrough volume, and effect of potentially interfering ions were studied. Under the optimized conditions, the extraction efficiency is >95%, the limits of detection are 2.1, 0.4 and 1.1 ng mL−1 for lead, copper and silver ions, and the adsorption capacities for these ions are 78.2, 68.1 and 52.3 mg g−1, respectively. The data obtained for the adsorption capacity of the sorbent shows the high tendency of the sorbent towards the target ions mentioned. Finally, this sorbent can be used as a simple, rapid, reliable, selective and sensitive method for the determination of trace levels of lead(II), copper(II) and silver(I) in different food samples.
1. Introduction
Because of the harmful negative effects of trace heavy metal ions on human health, environmental trace heavy metal pollution is one of the most serious problems for public health. The necessity of determining trace heavy metal ions in environmental samples has become more important in recent years.1–5 Accurate analysis of trace levels of metal ions is a complicated and difficult process. In the analysis of trace metal ions present in various samples, such as natural water and wastewater, sediment and tissue, direct determination with analytical techniques such as atomic absorption is not possible because of the matrix effect of foreign ions, the low concentration of metal ions in the sample and the low selectivity and sensitivity of the analytical techniques. It is necessary to preconcentrate the trace elements before their analysis to enhance the concentration of the analyte ions to be determined.6 Preconcentration–separation methods, including precipitation,7 solvent extraction,8 ion exchange,9 adsorption,10,11 electrochemical deposition,12 and membrane separation,13 are frequently used for the determination of trace metal levels in environmental matrices. Solid phase extraction is an extraction method that uses a solid phase and a liquid phase to isolate one, or one type, of analyte from a solution. Moreover, this method has the advantages of being more sensitive, simple, environmentally friendly, fast and sample-saving. It has been known as a powerful tool for the separation and pre-concentration of various inorganic and organic analytes.
In recent years, magnetite nanoparticles (MNPs) have been widely used as an efficient class of adsorbents.14–18 The use of nano-sized magnetic particles for selective separation and preconcentration in analytical chemistry may result in the development of a new methodology that is quicker, simpler and more precise than existing methodologies. The greatest advantage of this procedure is that the desired materials can be separated from the sample solution by a simple and compact process, producing fewer secondary wastes. Other advantages are the large active surface area for a given mass of particles, the ability to process solutions that contain suspended solids, the avoidance of channeling effects that are common in packed beds, and the ability to immobilize the target particles at a specific location in a column by the application of an external magnetic field. Earlier studies suggest that magnetic micro and nanoparticles have widespread applications as separation tools in the purification of nucleic acids, proteins and peptides, bacteria, and metals, because of their ability to agglomerate quickly and re-suspend in response to changes in magnetic forces. When magnetic nanoparticles are coated with poly(2-amino thiophenol), they gain a higher affinity for metal ions. This may be due to the coordinating ability of the N and S atoms, as well as π electrons, on the 2-amino thiophenol repeating units. The adsorption of lead(II), copper(II) and silver(I) on new nanocomposites is related to the sharing of an electron pair of N and S in a conducting polymer with target ions.19
In this study, for the first time, magnetic nanoparticles were modified with poly(2-amino thiophenol) and applied as a new solid-phase for the extraction of heavy metal ions, including lead(II), copper(II) and silver(I). A conducting polymer containing Fe3O4 nanoparticles was chosen because magnetic nanoparticles can be rapidly collected from a sample solution by applying magnetically assisted separation, thus shorter extraction times can be achieved. The synthesized sorbent was characterized by Fourier transform infrared spectrometry, elemental analysis, scanning electron microscopy, energy-dispersive X-ray spectrometry, thermogravimetric analysis and X-ray diffraction analysis. The effects of pH, flow rates, type, concentration and volume of eluent on the simultaneous elution of lead, copper and silver ions, as well as the break through volume and the effect of coexisting ions on the separation and determination of these heavy metals were investigated. The developed method was applied for the determination of lead, copper and silver ions in several real samples, and the accuracy of the method was confirmed using a standard reference material.
2. Experimental
2.1. Materials
Iron(II) chloride tetrahydrate (FeCl2·4H2O), iron(III) chloride hexahydrate (FeCl3·6H2O), tetraethoxysilane (TEOS), (3-aminopropyl)triethoxysilane (APTES), 2-amino thiophenol, ammonium persulfate, HCl, HNO3, toluene, and other solvents and reagents were obtained from Merck company (White-house Station, NJ). All the reagents were of analytical grade. Solutions of Cu(II), Pb(II) and Ag(I) were prepared from nitrate salts. Ore polymetallic gold Zidarovo PMZrZ (206 BG 326) from Bulgaria was used as the reference material for the validation of the proposed technique.
2.2. Synthesis of Fe3O4 nanoparticles
Fe3O4 nanoparticles were prepared using a co-precipitation method as follows:
1.72 g FeCl2·4H2O and 4.72 g FeCl3·6H2O were dissolved in 100 mL deionized water under a nitrogen atmosphere [Fe(II)/Fe(III) molar ratio = 1/2] and heated to 80 °C. Then, 25 mL NH4OH (28%) was added dropwise to the reaction mixture under vigorous stirring. The mixture was stirred for 30 min in an inert atmosphere and the final products were collected by a permanent magnet and washed several times with water and ethanol. The obtained magnetite Fe3O4 nanoparticles were dried at 25 °C in a vacuum oven for 24 h.20
2.3. Synthesis of Fe3O4@SiO2
Fe3O4@SiO2 core–shell structure was prepared by the hydrolysis of TEOS, using the Stöber method.21 Fe3O4 nanoparticles (1 g) were dispersed via ultrasonic irradiation in the solution of 250 mL isopropanol and 10 mL water, and then 40 mL ammonia solution was added. Then, a solution containing 3 mL TEOS and 40 mL isopropanol was added dropwise to the reaction mixture. The reaction was performed at room temperature for 18 h. The product was separated using an external magnet and washed several times with water and ethanol. The product was dried in a vacuum oven for 12 h.
2.4. Synthesis of Fe3O4@SiO2@SiC3H6NH2
Silica-coated magnetic nanoparticles (1.0 g) were dispersed in 70 mL toluene via sonication for 15 min. Then, 0.6 mL APTES was added to the abovementioned solution and the particles were redispersed for 2 min. The reaction was performed under reflux conditions at 80 °C for 24 hours. The product was separated by an external magnet and washed several times with water and ethanol. It was dried in a vacuum oven at 25 °C.22
2.5. Synthesis of Fe3O4@SiO2@SiC3H6NH2/poly(2-amino thiophenol) nanocomposite
Chemical oxidative method was used for the polymerization of 2-amino thiophenol. In this method, 0.5 g synthesized magnetic Fe3O4@SiO2@SiC3H6NH2 nanoparticles were dispersed in 40 mL HCl solution (0.1 M). Then, 1 mL 2-amino thiophenol was added to the abovementioned solution and sonicated for 15 min to increase the homogeneity. The reaction mixture was stirred for 2 h, until the monomers were adsorbed on the nanoparticles. Subsequently, the reaction flask was placed in an ice bath, until it reached 0 °C. Ammonium persulfate (2.13 g) was dissolved in 15 mL 0.1 M HCl to prepare an oxidant solution, and then it was added dropwise to the reaction mixture for 1 h (monomer/oxidant molar ratio was 1/1). The polymerization reaction was performed in an ice bath for 5 h. The product was separated using an external magnet and then washed several times with water and ethanol.19 Fig. 1 shows a step by step scheme for the synthesis of the Fe3O4@SiO2@SiC3H6NH2/poly(2-amino thiophenol) nanocomposite.
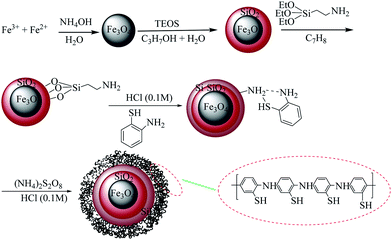 |
| Fig. 1 A scheme for the step by step synthesis of the sorbent. | |
2.6. Instruments
A Shimadzu atomic absorption spectrometer model AA-680 equipped with deuterium background correction was used for the determination of Cu(II), Ag(I) and Pb(II). The hollow cathode lamps (HCL) of the elements were used as radiation sources with wavelengths of 324.8, 328.1 and 283.3 nm, respectively. An air/acetylene flame was utilized in all the measurements. All the instrumental parameters were adjusted according to the manufacturer's recommendations.
The morphology of the prepared sorbent was characterized using SEM (Philips XL-30). X-ray diffraction patterns of the products were measured using a Siemens D5000 diffractometer. A 2θ scan in the range from 10 to 80 was recorded with monochromatic CuKα (λ = 1.54060 Å) radiation. The FT-IR spectra of the products in the form of KBr pellets were obtained using a BOMEM MB-series spectrometer. Thermograms of products were obtained using a thermal analyzer instrument (TGA/DTA BAHR: STA 503) at a heating rate of 10 °C min−1 under air. The pH measurements were carried out using a digital pH meter (Metrohm 827) equipped with a glass calomel electrode at 25 ± 1 °C.
2.7. Pretreatment of food samples
Eight types of fruits were chosen for the analysis. These were celery, cucumber, pepper, cabbage, persimmon, kiwi, carrot and pear, which were collected from the local supermarket (Tehran, Iran). 1.0 g of dried and ground samples were placed into a burning cup with 15 mL pure NHO3. The samples were incinerated in a MARS 5 microwave oven at 200 °C. After digestion, the samples were filtered through a Whatman filter paper no. 42. After filtration, the clear solution obtained was diluted to 50.0 mL (pH 7.0) for lead, copper and silver analysis.
2.8. Extraction procedure
A portion of sample solution containing the studied ions was transferred to a 150 mL beaker and then the pH of the solution was adjusted to the desired value. Then, 10 mg of magnetic sorbent was added and the solution was shaken for 5 min to facilitate the adsorption of the target ions onto the modified magnetic nanoparticles. Then, the magnetic adsorbent was rapidly separated using a strong and flat permanent magnet, and the supernatants were decanted directly, washed with 5 mL deionized water and again decanted directly. Finally, 3.0 mL 1 mol L−1 HCl was added as an eluent and shaken for 2 min and the eluted ions were determined by FAAS.
3. Results and discussion
3.1. Characterization of Fe3O4@SiO2@SiC3H6NH2/poly(2-amino thiophenol) nanocomposite
3.1.1. FT-IR analysis. Fig. 2 shows the FT-IR spectra of (a) Fe3O4, (b) Fe3O4@SiO2, (c) Fe3O4@SiO2@SiC3H6NH2 and (d) Fe3O4@SiO2@SiC3H6NH2/poly(2-amino thiophenol) nanocomposite.
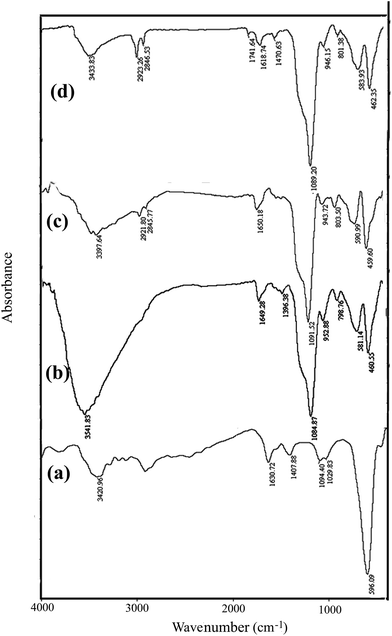 |
| Fig. 2 FT-IR spectra of (a) Fe3O4, (b) Fe3O4@SiO2, (c)Fe3O4@SiO2@SiC3H6NH2 and (d) Fe3O4@SiO2@SiC3H6NH2/poly(2-amino thiophenol) nanocomposite. | |
In Fig. 2a, the absorption peak at 596 cm−1 is related to the Fe–O–Fe vibration of Fe3O4 nanoparticles and the broad peak at 3200–3600 cm−1 is assigned to the O–H stretching vibration of surface hydroxyl groups or water adsorbed onto the surface of the nanoparticles.20,23 In Fig. 2b, the strong peaks at 952 cm−1 and 1084 cm−1 are attributed to the Si–O–Si vibration and the peak at 581 cm−1 corresponds to the Fe–O–Si bond, which demonstrates the presence of the silica layer on Fe3O4 nanoparticles.24 In Fig. 2c, the vibration bands of C–Si and C–N at 1300 cm−1 and 1100 cm−1, respectively, and the bands of aliphatic –CH2– at 2845 cm−1 and 2921 cm−1 demonstrate the presence of APTES on the surfaces of the nanoparticles.25 Fig. 2d shows the absorption peaks of the polymer nanocomposite, including the absorptions at 1618 cm−1 and 1741 cm−1 for the C
C vibration of aromatic rings and S–H and N–H vibrations at 3433 cm−1.19
3.1.2. X-ray diffraction analysis. The XRD patterns of the synthesized nanoparticles are displayed in Fig. 3. In pattern (a), the diffraction peaks at 2θ = 30.09°, 35.44°, 43.07°, 53.43°, 57.15°, and 62.42° are assigned to the magnetic spinel structures of Fe3O4 or γ-Fe2O3.20,23 In Fig. 3b, the broad peak at 2θ = 20–30° demonstrates the presence of a silica layer on the surface of the magnetic nanoparticles.24 All the peaks of Fe3O4@SiO2 are similar to those of Fe3O4@SiO2@SiC3H6NH2 (Fig. 3c). In this figure, part (d) shows the broad peak at 15° < 2θ < 25°, which confirmed the existence of an amorphous polymer layer on the surface of the nanocomposite.
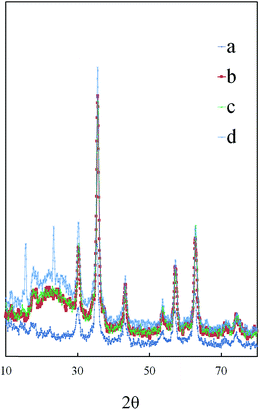 |
| Fig. 3 XRD pattern of (a) Fe3O4, (b) Fe3O4@SiO2, (c)Fe3O4@SiO2@SiC3H6NH2 and (d) Fe3O4@SiO2@SiC3H6NH2/poly(2-amino thiophenol) nanocomposite. | |
3.1.3. Elemental analysis. Elemental analysis of the synthesized Fe3O4@SiO2@SiC3H6NH2 demonstrated 3.27%, 1.22% and 0.57% C, H and N, respectively. However, for the Fe3O4@SiO2@SiC3H6NH2/poly(2-amino thiophenol) nanocomposite, 11.06%, 1.63%, 1.74% and 4.087% of C, H, N, and S, were observed, respectively. The results show that the APTES and the polymer were immobilized on the surface of the magnetic nanoparticles.
3.1.4. SEM and energy-dispersive X-ray spectrometry (EDX). The morphology and size of the nanoparticles were determined using SEM. The elementary content was evaluated by EDX and SEM. The SEM micrographs were obtained, as shown in Fig. 4. The SEM images of (a) Fe3O4, (b) Fe3O4@SiO2, (c) Fe3O4@SiO2@SiC3H6NH2 and (d) Fe3O4@SiO2@SiC3H6NH2/poly(2-amino thiophenol) nanocomposite show that the average size of these nanoparticles are about 15, 30, 35 and 52 nm, respectively. These nanoparticles have a spherical structure with a smooth surface morphology and a narrow size distribution. The EDX spectra of magnetic nanoparticles were obtained for the elemental analysis results, which confirmed the presence of silica, amino silane and polymer in the obtained products in each stage (Fig. 4e–h).
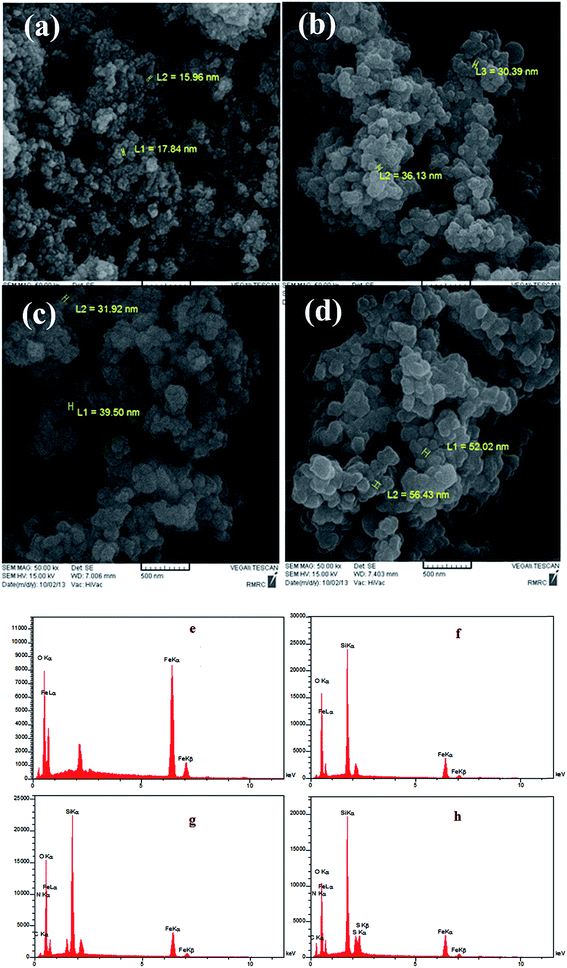 |
| Fig. 4 SEM micrographs of (a) Fe3O4, (b) Fe3O4@SiO2, (c) Fe3O4@SiO2@SiC3H6NH2 and (d) Fe3O4@SiO2@SiC3H6NH2/poly(2-amino thiophenol) nanocomposite; (e), (f), (g) and (h) are the EDX spectra from the corresponding areas of (a), (b), (c) and (d), respectively. | |
3.1.5. Thermogravimetric analysis. The thermal stability of the nanoparticles and the content of organic constituents on the surface of the nanoparticles were assessed using the TGA results (Fig. 1S ESI†). The thermogravimetric curves show a weight loss at temperatures below 200 °C, which corresponds to the removal of adsorbed water and CO2. The thermogravimetric curves of the Fe3O4@SiO2@SiO3C3H6NH2 display a weight loss at temperatures above 200 °C, which is due to the decomposition of aminopropyl groups, and this observation demonstrated the presence of an APTES coating.26 Curve d shows a mass loss at 350–500 °C due to the decomposition of poly(2-amino thiophenol). From comparison of the curves c and d, the percentage of polymers was estimated to be 35% of the weight of the nanocomposite. The TGA results are in good agreement with the elemental analyses and other results.
3.2. Effect of pH on retention of target ions by the synthesized sorbent
To study the effect of pH on the extraction of target ions, the pH of 25 mL of different sample solutions containing 2 mg L−1 of each target ion was adjusted in the range of 2–10. The interactions between electron pairs of N and S of the conducting polymer on the nanocomposite and target ions were effective at natural pH values. The results obtained and shown in Fig. 5 indicate that the target ions could be retained simultaneously on functionalized Fe3O4 NPs at pH 7.0. The adsorption of lead, copper and silver ions by the sorbent increased from pH 2.0 to 7.0 but decreased slightly from pH 7.0 to 10.0. By decreasing the pH value of the solution, the quantitative removal of the target ions was also decreased due to electrostatic repulsion of the protonated active sites on the sorbent with the positively charged lead, copper and silver species. Moreover, the observed decrease in the percentage retention of lead, copper and silver ions on the sorbent at pH values higher than 7.0 is most probably due to the precipitation of target ions in the hydroxide form, which leads to a decrease in the concentration of free lead, copper and silver ions in the sample. Thus, pH of 7.0 was chosen as the optimum pH for further experiments.
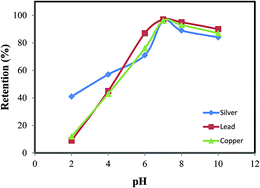 |
| Fig. 5 The effect of solutions' pH on the retention of the target ion by the synthesized sorbent. | |
3.3. Effect of the adsorbent amounts
Compared to conventional sorbents (microsized adsorbents), NPs offer a significantly higher surface-area-to-volume ratio and a short diffusion route, which result in high extraction capacities, rapid extraction dynamics and high extraction efficiencies. Moreover, MNPs can be rapidly collected from the sample solution by applying magnetically assisted separation, thus shorter extraction times can be achieved. Therefore, satisfactory results can be obtained with fewer amounts of these adsorbents. For the optimization of the amount of adsorbent, 5, 10, 15 and 20 mg of the MNP were tested in 150 mL of the sample solution. In the present study, by increasing the amounts of the magnetic nanoparticles modified with poly(2-amino thiophenol), due to an increase in the surface area and accessible sites for the adsorption of the analytes, the extraction efficiency increased. Quantitative extraction of the target ions was achieved using only 10 mg of the magnetic nanoparticles modified with poly(2-amino thiophenol). At higher amounts of the adsorbent, the extraction efficiency was almost constant. Therefore, 10 mg of the sorbent was chosen for further studies.
3.4. Equilibrium sorption time
To investigate the effect of shaking time on extraction efficiency, extraction experiments were carried out at 2, 5, 10, 20, and 30 min time intervals. According to the results, an equilibration time of about 5 min was required for the quantitative extraction of the target ions from solution into the solid phase (ESI, Fig. 2S†). Thus, the mixtures have been shaken for 5 min to reach equilibrium in the subsequent experiments.
3.5. Desorption condition
As the surface of the magnetic nanoparticles is modified with poly(2-amino thiophenol) that is coated with a silica layer, this composite is stable in high concentrations of acids without any leakage of Fe3+ or Fe2+ in solution. Thus, a series of selected eluent solutions, including HNO3, HCl, HNO3
:
HCl and CH3COOH, was used for the elution of target ions from the magnetic nanoparticles modified with poly(2-amino thiophenol). The results show that 1 mol L−1 HCl is a suitable and effective eluent to simultaneously elute the target ions from magnetic nanoparticles modified with poly(2-amino thiophenol). The effect of eluent volume on the recovery of target ions was also studied (Table 1). Moreover, the results show that quantitative recovery can be achieved with 3.0 mL 1 mol L−1 HCl. Therefore, a 3.0 mL volume of eluent for the desorption of target ions was used in the following experiments.
Table 1 The effect of type, concentration, volume and time of the elution step on the extraction recovery of lead, silver and copper ions
Eluent |
Concentration (mol L−1) |
Shaking time (min) |
Volume (mL) |
Recovery% ± standard deviation |
Lead |
Copper |
Silver |
HNO3 |
2 |
15 |
10.0 |
84.0 ± 1.2 |
85.0 ± 1.4 |
52.0 ± 1.2 |
HCl |
2 |
15 |
10.0 |
96.0 ± 1.1 |
96.0 ± 1.2 |
96.0 ± 1.4 |
CH3COOH |
2 |
15 |
10.0 |
31.0 ± 1.1 |
71.0 ± 1.2 |
38.0 ± 1.3 |
HNO3 : HCl |
1 : 1 |
15 |
10.0 |
76.0 ± 1.3 |
81.0 ± 1.2 |
94.0 ± 1.2 |
HCl |
1.5 |
15 |
10.0 |
96.0 ± 1.4 |
96.0 ± 1.2 |
96.0 ± 1.5 |
HCl |
1 |
15 |
10.0 |
96.0 ± 1.3 |
96.0 ± 1.4 |
96.0 ± 1.2 |
HCl |
0.5 |
15 |
10.0 |
82.0 ± 1.3 |
84.0 ± 1.3 |
78.0 ± 1.3 |
HCl |
0.25 |
15 |
10.0 |
75.0 ± 1.2 |
71.0 ± 1.5 |
65.0 ± 1.1 |
HCl |
1 |
15 |
5.0 |
96.0 ± 1.2 |
96.0 ± 1.0 |
96.0 ± 1.4 |
HCl |
1 |
15 |
4.0 |
96.0 ± 1.0 |
96.0 ± 1.2 |
96.0 ± 1.2 |
HCl |
1 |
15 |
3.0 |
96.0 ± 1.3 |
96.0 ± 1.1 |
96.0 ± 1.4 |
HCl |
1 |
15 |
2.5 |
89.0 ± 1.3 |
85.0 ± 1.2 |
90.0 ± 1.0 |
HCl |
1 |
10 |
3.0 |
96.0 ± 1.1 |
96.0 ± 1.3 |
96.0 ± 1.3 |
HCl |
1 |
5 |
3.0 |
96.0 ± 1.4 |
96.0 ± 1.2 |
96.0 ± 1.3 |
HCl |
1 |
2 |
3.0 |
96.0 ± 1.2 |
96.0 ± 1.3 |
96.0 ± 1.2 |
Desorption times were evaluated in the range of 2–15 min. The results showed that a time of 2 min is sufficient for the simultaneous desorption of the target ions by 3.0 mL 1 mol L−1 HCl.
3.6. Effect of sample volume
Due to the low concentrations of trace metals in real samples, when using samples with large volumes, the trace metals in these volumes should be concentrated into smaller volumes to achieve a high preconcentration factor. Therefore, the maximum sample volume was optimized by the investigation of the recovery of target ions in various synthetic samples, using volumes in the range of 50–800 mL containing 0.015 mg of targets. In the optimization of the sample volume, 10 mg of the sorbent was used. The recoveries of target metals from different volumes of aqueous solutions are shown in Fig. 6. The recovery was found to be stable up to 500 mL and this was chosen as the largest sample volume to be used in this study.
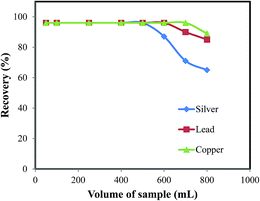 |
| Fig. 6 The effect of sample volume on the recovery of lead, silver and copper ions by the synthesized sorbent. | |
3.7. Effect of potentially interfering ions
Because of the presence of other elements in real samples, the determination and preconcentration of target ions is difficult. Thus, the effects of common coexisting cations and anions on the adsorption of the target ions on the magnetic nanoparticles modified with poly(2-amino thiophenol) were investigated. In these experiments, 150 mL of solution containing 0.003 mg target ions were added to interfering cations and anions and treated according to the recommended procedure. The results in Table 2 show that the vast majority of transition, alkaline, and alkaline earth metals do not interfere at environmentally relevant concentrations. This is due to the low capacity or rates of adsorption for interfering ions under optimum conditions. Thus, these results confirm that the procedure using magnetic nanoparticles modified with poly(2-amino thiophenol) is independent of matrix interference.
Table 2 The effect of potentially interfering ions on the extraction recovery of lead, silver and copper by the synthesized sorbenta
Interfering ion |
Recovery% ± standard deviation |
Pb(II) |
Cu(II) |
Ag(I) |
The ratio of the concentration of interfering ions/target ions was 1000. |
K+ |
97.0 ± 1.2 |
99.0 ± 1.0 |
98.0 ± 1.5 |
Na+ |
98.0 ± 1.3 |
97.0 ± 1.2 |
99.0 ± 1.0 |
Cs+ |
98.0 ± 1.1 |
98.0 ± 1.2 |
97.0 ± 1.2 |
Ca2+ |
97.0 ± 1.4 |
96.0 ± 1.1 |
96.0 ± 1.5 |
Ba2+ |
97.0 ± 1.3 |
97.0 ± 1.2 |
97.0 ± 1.1 |
Mg2+ |
97.0 ± 1.2 |
97.0 ± 1.3 |
96.0 ± 1.7 |
Co2+ |
95.0 ± 1.2 |
95.0 ± 1.1 |
95.0 ± 1.7 |
Fe2+ |
96.0 ± 0.8 |
95.0 ± 1.4 |
96.0 ± 1.0 |
Mn2+ |
95.0 ± 0.6 |
96.0 ± 0.6 |
95.0 ± 1.1 |
Ni2+ |
96.0 ± 0.5 |
95.0 ± 1.5 |
95.0 ± 1.2 |
Fe3+ |
95.0 ± 0.8 |
96.0 ± 1.0 |
95.0 ± 1.2 |
Cd2+ |
95.0 ± 0.6 |
96.0 ± 0.5 |
95.0 ± 1.1 |
NO3− |
95.0 ± 1.1 |
96.0 ± 1.1 |
95.0 ± 1.2 |
SO42− |
95.0 ± 1.2 |
95.0 ± 0.3 |
96.0 ± 1.3 |
3.8. Maximum adsorption capacity
To determine the amount of sorbent that was required to quantitatively remove a specific amount of a metal ion from the solution, the capacity of the sorbent was calculated. To evaluate this factor, 25 mL of a solution containing 1 mg target ions underwent the extraction procedure and the maximum capacity was calculated (with 10 mg of the synthesized sorbent). The obtained capacities of magnetic nanoparticles modified with poly(2-amino thiophenol) were found to be 78.2, 68.1 and 52.3 mg g−1 for lead, copper and silver ions, respectively. To evaluate the maximum adsorption capacity of the sorbent, the difference between the concentration of the solution before and after solid phase extraction was calculated.
3.9. Analytical performance
Under the optimized conditions, calibration curves were plotted for the determination of lead, copper and silver ions according to the optimum extraction procedure. Linearity was maintained at 1–200 μg L−1 for copper, 3–250 μg L−1 for lead and 2–250 μg L−1 for silver in the initial solution (150 mL of sample). The correlation of determination (R2) was 0.996 for copper, 0.997 for lead and 0.994 for silver ions. The limit of quantification (LOQ) for the trace detection of copper, lead and silver ions by the proposed solid phase extraction method was 1, 3 and 2 μg L−1, respectively. The limit of detection, which is defined as CLOD = 3Sb/m, where Sb is the standard deviation of eight replicate blank signals and m is the slope of the calibration curve after preconcentration, for a sample volume of 150 mL, was found to be 0.4 ng mL−1 for copper, 2.2 ng mL−1 for lead and 1.1 ng mL−1 for silver ions. The relative standard deviations for eight separate experiments for the determination of 7.5 μg of lead, copper and silver ions in 150 mL of water was 2.4%, 2.2% and 2.7%, respectively. The regression equation, dynamic linear range (DLR), correlation of determination (R2), preconcentration factor (PF), limit of detection (LOD) and relative standard deviation (RSD) for lead, copper and silver were calculated under optimized conditions and are summarized in Table 3. To demonstrate the applicability of the proposed method for the removal and pre-concentration of target ions, recovery was calculated for a known concentration (50 ng mL−1) of these target ions. The obtained data for recovery is presented in Table 3.
Table 3 Regression equation, dynamic linear range (DLR), correlation of determination (R2), preconcentration factor (PF), limit of detection (LOD) and relative standard deviation (RSD) for lead, copper and silver were calculated under optimized conditions
Analyte |
Regression equation |
R2 |
LOD (ng mL−1) |
DLR (ng mL−1) |
PF |
Recoverya (%) |
RSDa (%) |
Maximum sorption capacity (mg g−1) |
Recovery and RSD were calculated for a known concentration (50 ng mL−1) of the target ions. |
Copper ion |
Y = 1.412X + 0.002 |
0.996 |
0.4 |
1–200 |
50 |
96.7 |
2.2 |
68.1 |
Lead ion |
Y = 1.202X + 0.001 |
0.997 |
2.1 |
3–250 |
50 |
97.2 |
2.4 |
78.2 |
Silver ions |
Y = 1.241X + 0.001 |
0.994 |
1.1 |
2–250 |
50 |
98.4 |
2.7 |
52.3 |
Because 150 mL of the solution was preconcentrated to 3.0 mL, an approximate preconcentration factor of 50 was obtained.
3.10. Real sample analysis
Finally, this method was performed on real samples to check its accuracy. The amounts of lead, copper and silver ions in real samples are too low to be reliably determined. To test the reliability of the method, it was applied to the extraction and determination of the target ions in the food samples. The results demonstrate that quantitative recoveries of the target ions for magnetic nanoparticles modified with poly(2-amino thiophenol) are achievable. The results are listed in Table 4.
Table 4 Analysis of silver, copper and lead ions in different food samples
Food sample |
Element |
Real sample (μg kg−1) |
Added (μg kg−1) |
Found (μg kg−1) |
Recovery (%) |
Celery |
Pb |
7.1 ± 0.1 |
10.0 |
17.2 ± 0.1 |
100.5 |
Ag |
2.2 ± 0.1 |
10.0 |
12.1 ± 0.1 |
99.2 |
Cu |
11.2 ± 0.1 |
10.0 |
21.0 ± 0.1 |
99.0 |
Cucumber |
Pb |
6.5 ± 0.1 |
10.0 |
16.3 ± 0.2 |
98.8 |
Ag |
N.D. |
10.0 |
9.8 ± 0.1 |
98.0 |
Cu |
19.5 ± 0.2 |
10.0 |
29.3 ± 0.1 |
99.3 |
Pepper |
Pb |
5.7 ± 0.1 |
10.0 |
15.5 ± 0.1 |
98.7 |
Ag |
N.D. |
10.0 |
9.9 ± 0.1 |
99.0 |
Cu |
14.2 ± 0.2 |
10.0 |
24.0 ± 0.1 |
99.2 |
Cabbage |
Pb |
5.9 ± 0.1 |
10.0 |
15.6 ± 0.2 |
98.1 |
Ag |
2.4 ± 0.2 |
10.0 |
12.5 ± 0.1 |
100.8 |
Cu |
11.8 ± 0.2 |
10.0 |
21.6 ± 0.1 |
99.1 |
Persimmon |
Pb |
4.3 ± 0.1 |
10.0 |
14.4 ± 0.1 |
100.6 |
Ag |
2.3 ± 0.1 |
10.0 |
12.1 ± 0.2 |
98.4 |
Cu |
10.2 ± 0.2 |
10.0 |
20.1 ± 0.1 |
99.5 |
Kiwi |
Pb |
3.9 ± 0.1 |
10.0 |
13.6 ± 0.1 |
97.8 |
Ag |
N.D. |
10.0 |
9.8 ± 0.1 |
98.0 |
Cu |
10.5 ± 0.2 |
10.0 |
20.2 ± 0.1 |
98.5 |
Carrot |
Pb |
4.4 ± 0.1 |
10.0 |
14.2 ± 0.2 |
98.6 |
Ag |
2.1 ± 0.1 |
10.0 |
12.0 ± 0.1 |
99.2 |
Cu |
14.4 ± 0.2 |
10.0 |
24.1 ± 0.2 |
98.8 |
Pear |
Pb |
6.4 ± 0.1 |
10.0 |
16.1 ± 0.1 |
98.2 |
Ag |
N.D. |
10.0 |
9.8 ± 0.1 |
98.0 |
Cu |
13.5 ± 0.2 |
10.0 |
23.3 ± 0.1 |
99.1 |
The concentration of copper, lead and silver ions obtained by magnetic nanoparticles modified with poly(2-amino thiophenol) was compared to the standard reference material. For this, the concentration of the copper, lead and silver ions was determined under optimum conditions for the standard reference material (ore polymetallic gold Zidarovo PMZrZ (206 BG 326)). As can be seen in Table 1S (ESI),† good correlation was achieved between the estimated content and the found content by the present method for the reference materials. Therefore, magnetic nanoparticles modified with poly(2-amino thiophenol) can be used as a reliable magnetic solid phase for the extraction and determination of copper, lead and silver ions in real samples.
3.11. Comparison of the proposed solid phase extraction with those in the literature
A conducting polymer containing Fe3O4 nanoparticles was chosen because magnetic nanoparticles can be rapidly collected from a sample solution by applying magnetically assisted separation, thus shorter extraction times can be achieved. When magnetic nanoparticles are coated with poly(2-amino thiophenol), they gain a higher affinity for metal ions. This may be due to the coordinating ability of the N and S atoms, as well as π electrons, on the (2-amino thiophenol) repeating units.
The proposed method was compared with other reported methods in the literature. According to Table 5, the appropriate LOD was achieved by the proposed method. This method is clearly different from other reported methods in breakthrough volume, dynamic linear range and maximum adsorption capacity.27–32
Table 5 A comparison between the proposed solid phase and previously applied sorbents
System |
Element |
Detection technique |
pH |
LOD (ng mL−1) |
RSD% |
DLR (ng mL−1) |
BVa (mL) |
ACb (mg g−1) |
Ref. |
Breakthrough volume. Adsorption capacity. |
Poly hydroxybutyrate-b-poly (ethylene glycol) |
Cu |
FAAS |
6.0 |
0.09 |
2.1 |
0.3–80 |
1300 |
95 |
27 |
Pb |
— |
— |
— |
— |
— |
— |
Ag |
— |
— |
— |
— |
— |
— |
Fe3O4@SiO2@IIP |
Cu |
FAAS |
— |
— |
— |
— |
— |
— |
28 |
Pb |
7.5 |
— |
— |
— |
300 |
19.61 |
Ag |
— |
— |
— |
— |
— |
— |
DBDC chelates on Dowex Optipore V-493 |
Cu |
FAAS |
— |
— |
— |
— |
— |
— |
29 |
Pb |
6.0 |
0.65 |
<5 |
0.43–0.65 |
250 |
7.3 |
Ag |
— |
— |
— |
— |
— |
— |
Fe3O4@PTh NPs |
Cu |
ICP-OES |
4.0 |
0.5 |
2.9 |
1.0–100 |
100 |
— |
30 |
Pb |
— |
— |
— |
— |
— |
— |
Ag |
4.0 |
0.2 |
4.2 |
0.75–100 |
100 |
— |
Alumina-coated magnetite nanoparticles |
Cu |
FAAS |
— |
— |
— |
— |
— |
— |
31 |
Pb |
— |
— |
— |
— |
— |
— |
Ag |
6.5 |
0.56 |
3.1 |
2–100 |
500 |
11.6 |
Fe3O4@SiO2@H2Dz |
Cu |
ICP-OES |
7.0 |
0.01 |
2.4 |
0.5–100 |
100 |
20.5 |
32 |
Pb |
7.0 |
0.01 |
2.0 |
0.5–100 |
100 |
60.9 |
Ag |
— |
— |
— |
— |
— |
— |
Fe3O4@SiO2@SiC3H6NH2/P2ATP |
Cu |
FAAS |
7.0 |
0.4 |
2.2 |
1–200 |
500 |
68.1 |
This work |
Pb |
7.0 |
2.1 |
2.4 |
3–250 |
500 |
78.2 |
Ag |
7.0 |
1.1 |
2.7 |
2–250 |
500 |
52.3 |
4. Conclusions
In this study, for the first time, magnetic nanoparticles modified with poly(2-amino thiophenol) were utilized as an adsorbent for the simultaneous separation of ultra-trace amounts of lead, copper and silver ions. This method is simple, rapid and reliable and is found to be a selective and sensitive method for the determination of trace levels of lead, copper and silver ions. The most important characteristic of the magnetic nanoparticles modified with poly(2-amino thiophenol) is that they show excellent selectivity toward lead, copper and silver ions over other ions under the optimized conditions. Convenient data were found for the detection limit and the pre-concentration factor in the determination of the target ions and it was confirmed that this method using magnetic nanoparticles modified with poly(2-amino thiophenol) has high potential for the extraction of metal ions.
References
- M. Behbahani, M. Najafi, M. M. Amini, O. Sadeghi, A. Bagheri and M. Salarian, Microchim. Acta, 2013, 180, 911 CrossRef CAS.
- M. Behbahani, M. Barati, M. Kalate Bojdi, A. R. Pourali, A. Bagheri and N. Akbari Ghareh Tapeh, Microchim. Acta, 2013, 180, 1117 CrossRef CAS.
- M. Behbahani, M. Taghizadeh, A. Bagheri, H. Hosseini, M. Salarian and A. Tootoonchi, Microchim. Acta, 2012, 178, 429 CrossRef CAS.
- M. Salarian, A. Ghanbarpour, M. Behbahani, S. Bagheri and A. Bagheri, Microchim. Acta, 2014, 181, 999 CrossRef CAS.
- M. Behbahani, A. A. Akbari, M. M. Amini and A. Bagheri, Anal. Methods, 2014, 6, 8785–8792 RSC.
- M. Behbahani, P. Ghareh Hassanlou, M. M. Amini, F. Omidi, A. Esrafili, M. Farzadkia and A. Bagheri, Food Chem., 2015, 187, 82 CrossRef CAS PubMed.
- M. A. Saito and D. L. Schneider, Anal. Chim. Acta, 2006, 565, 222 CrossRef CAS PubMed.
- R. Shukla and G. N. Rao, Talanta, 2002, 57, 633 CrossRef CAS.
- T. C. Miller and J. A. Holcombe, Anal. Chim. Acta, 2002, 454, 137 CrossRef.
- R. L. Dutra, H. F. Maltez and E. Carasek, Talanta, 2006, 69, 488 CrossRef CAS PubMed.
- M. Haratake, K. Yasumoto, M. Ono, M. Akashi and M. Nakayama, Anal. Chim. Acta, 2006, 561, 183 CrossRef CAS PubMed.
- M. Burguera, J. L. Burguera, D. Rivas, C. Rondón, P. Carrero, O. M. Alarcón, Y. P. Peña, M. R. Brunetto, M. Gallignani, O. P. Márquez and J. Márquez, Talanta, 2005, 68, 219 CrossRef CAS PubMed.
- L. Irigoyen, C. Moreno, C. Mendiguchía and M. G. Vargas, J. Membr. Sci., 2006, 274, 169 CrossRef CAS PubMed.
- A. Bagheri, M. Behbahani, M. M. Amini, O. Sadeghi, A. Tootoonchi and Z. Dahaghin, Microchim. Acta, 2012, 178, 261 CrossRef CAS.
- M. Behbahani, S. Bagheri, M. M. Amini, H. Sadeghi Abandansari, H. R. Moazami and A. Bagheri, J. Sep. Sci., 2014, 37, 1610 CrossRef CAS PubMed.
- E. Ghorbani-Kalhor, M. Behbahani, J. Abolhasani and R. Hosseinzadeh Khanmiri, Food Analytical Methods, 2015, 8, 1326 CrossRef.
- A. Bagheri, M. Taghizadeh, M. Behbahani, A. A. Asgharinezhad, M. Salarian, A. Dehghani, H. Ebrahimzadeh and M. M. Amini, Talanta, 2012, 99, 132 CrossRef CAS PubMed.
- S. Khan, T. Gul Kazi and M. Soylak, Spectrochim. Acta, Part A, 2014, 123, 194 CrossRef CAS PubMed.
- M. R. Nabid, R. Sedghi, A. Bagheri, M. Behbahani, M. Taghizadeh, H. Abdi Oskooie and M. M. Heravi, J. Hazard. Mater., 2012, 203, 93 CrossRef PubMed.
- K. D. Kim, S. S. Kim, Y. H. Choa and H. T. Kim, J. Ind. Eng. Chem., 2007, 13, 1137 CAS.
- W. Stöber, A. Fink and E. Bohn, J. Colloid Interface Sci., 1968, 26, 62 CrossRef.
- B. Panella, A. Vargas, D. Ferri and A. Baiker, Chem. Mater., 2009, 21, 4316 CrossRef CAS.
- N. Sreeja, N. S. Binulal, M. Ullas, K. Manzoor, V. N. Shantikumar and M. Deepthy, ACS Appl. Mater. Interfaces, 2012, 4, 251 Search PubMed.
- Q. Du, W. Zhang, H. Ma, J. Zheng, B. Zhou and Y. Li, Tetrahedron, 2012, 68, 3577 CrossRef CAS PubMed.
- J. M. Zhang, S. R. Zhai, B. Zhai, Q. D. An and G. Tian, J. Sol-Gel Sci. Technol., 2012, 64, 347 CrossRef CAS.
- B. Panella, A. Vargas, D. Ferri and A. Baiker, Chem. Mater., 2009, 21, 4316 CrossRef CAS.
- M. Behbahani, J. Abolhasani, M. M. Amini, O. Sadeghi, F. Omidi, A. Bagheri and M. Salarian, Food Chem., 2015, 173, 1207 CrossRef CAS PubMed.
- M. Zhang, Z. Zhang, Y. Liu, X. Yang, L. Luo, J. Chen and S. Yao, Chem. Eng. J., 2011, 178, 443 CrossRef CAS PubMed.
- E. Melek, M. Tuzen and M. Soylak, Anal. Chim. Acta, 2006, 578, 213 CrossRef CAS PubMed.
- E. Tahmasebi and Y. Yamini, Microchim. Acta, 2014, 181, 543 CrossRef CAS.
- M. A. Karimi, S. Z. Mohammadi, A. Mohadesi, A. Hatefi, M. Mazloum-Ardakani and L. Sotudehnia Korani, Sci. Iran., Trans. F, 2011, 18, 790 CrossRef CAS PubMed.
- G. Cheng, M. He, H. Peng and B. Hu, Talanta, 2012, 88, 507 CrossRef CAS PubMed.
Footnote |
† Electronic supplementary information (ESI) available. See DOI: 10.1039/c5ra11561b |
|
This journal is © The Royal Society of Chemistry 2015 |