DOI:
10.1039/C5RA11379B
(Paper)
RSC Adv., 2015,
5, 85410-85416
A microfluidic capacitive immunosensor system for human cartilage chitinase-3-like protein 2 (hYKL-39) quantification as an osteoarthritis marker in synovial joint fluid†
Received
15th June 2015
, Accepted 28th September 2015
First published on 29th September 2015
Abstract
Worldwide, many people suffer from osteoarthritis, a painful condition that interferes with normal work and the enjoyment of life and is thus a serious personal and public problem. Good management of osteoarthritis requires methodologies for accurate and timely detection of disease onset, progression and response to treatment. Here, capacitive electrochemical immunosensors, using immobilized antibodies against the known osteoarthritis biomarker human cartilage chitinase-3-like protein 2, also known as hYKL-39, were used as novel tools for sensitive and selective osteoarthritis analysis. In the proposed procedure the current response of the sensor to a potential step application is measured; from the resultant traces, concentration-dependent capacitance changes, due to antigen binding, can be calculated. The linear range and detection limit of capacitive hYKL-39 immunosensing were respectively 0.1–1000 μg L−1 and 0.074 μg L−1. This was better than the characteristics of the enzyme-linked immunosorbent assay (ELISA) with an optical readout, which is presently the standard practice for laboratory hYKL-39 screening. In synovial fluid samples from osteoarthritis patients, capacitive hYKL-39 immunosensing reported the expected elevated hYKL-39 biomarker levels in quantitative agreement with ELISA analysis. The improved detection limit and linear range of the electrochemical hYKL-39 immunoassay combined with the potential of cheap, simple, miniaturizable, portable and automatable equipment recommend this novel approach as a promising complementary tool and ideal reference assay for osteoarthritis diagnosis and monitoring, in particular when the aim is early detection.
1. Introduction
Osteoarthritis (OA) is the degeneration of weight-bearing joints, and in its later stages it involves the breakdown of the cartilage layer at the terminals of the articulating bones; the consequential direct bone rubbing and abrasion result in acute or, in severe cases chronic, joint pain, tenderness, stiffness, locking and swelling.1 Reduced usage of sore joints is a common reaction of people with OA, and muscle and ligament weakening around the affected sites are frequently found as secondary symptoms. Limiting painful body movements hinder chronic OA patients in their ability to work, move and exercise and result in a diminished quality of life and also a burden to public health systems. Dealing with OA-related individual suffering and public socioeconomic issues involves disease management, medical care and the assessment of potential disease-modifying OA treatments, and so requires sensitive analytical methods for disease detection and clinical monitoring of the course of the disease. Presently, diagnosis of OA uses a combination of qualitative classification of the patient's pain levels and observation of clinical signs of joint movement reduction, joint enlargement/swelling and joint crepitus.2 A positive medical check is usually followed by joint inspections using X-ray radiography or ultrasound scanning or by more expensive magnetic resonance imaging (MRI), computer tomography (CT) or radionuclide bone scans (RBS). Noticeable signs of, for instance, reduced joint space width and/or cartilage splintering, perforation or thinning confirm the presence of OA; however, with common imaging methods clear morphological changes in joint structure are only visible at advanced stages of OA, rather than at earlier stages, when therapeutic interventions would be most effective.
The drawbacks of clinical radiology in detecting premature and slowly changing OA states and the high costs and limited availability of MRI/CT/RBS OA diagnostic testing prompted a search for alternatives for analysis of this defect. Progress in biomarker-based disease diagnosis and prognosis suggested that this type of approach might also be applicable to OA. Blood, urine and synovial fluid levels of the products of cartilage collagen and aggrecan metabolism, joint inflammation signalling molecules and joint glycoproteins, proteoglycans and glycation end-products have been reported as indicators of degenerative joint destruction.2–6 More precisely, C-terminal telopeptide of collagen type II (CTX-II),7 cartilage oligomeric proteins (COMPs),8 matrix metallo-proteinases9,10 and also hYKL-39 (ref. 11–13) were recognized as biomarkers for OA, through a combination of molecular biology, biochemistry and pathology. The routine method for detecting biomarkers in body fluids is the optical enzyme-linked immunosorbent assay (ELISA), which requires antibody against the indicator molecule and an enzyme-labelled secondary antibody for signal generation.
Detection of OA biomarkers using techniques other than ELISA is possible but has rarely been attempted. Examples are optical CTX-II and COMP microfluidic assays that work with antibody-conjugated fluoro-microbeads as functional components.14,15 For COMP an immunochromatographic strip test, with gold nanoparticle-linked biomarker antibody immobilized on the sensing surface, was also reported.16 To promote electrochemical signalling as an option for signal transduction in OA biomarker immunoassays we demonstrate here, for the first time, the suitability of flow-based capacitive immunosensing for reliable OA biomarker analysis. Because of experience in the preparation and structural and functional characterization of chitinase and chitinase-like proteins and also successful quantification of the cancer biomarker human chitinase-3-like protein 1 (hYKL-40) in blood serum,17 hYKL-39 was chosen as the biomarker in this proof-of-principle study. The requisite electrochemical immunosensors were produced by fixing anti-hYKL-39 to a thin but insulating self-assembling monolayer of thiourea/1-dodecanethiol on gold electrodes. The concentration-dependent signal in hYKL-39 quantification was the exponentially decaying capacitive current response of completed hYKL-39 immunosensors to potential steps. The performance of capacitive hYKL-39 immunosensing was tested for the determination of the OA marker in ‘spiked’ samples spanning a pathologically relevant analyte concentration range, in lysates from various human cells and in synovial fluid samples from OA patients in a local clinic. Parallel assessments of duplicates of all these samples with conventional optical ELISA confirmed the data from the novel electrochemical hYKL-39 test, proving the validity of the methodology and promoting further applications for clinical osteoarthritis diagnosis. All relevant study details are presented hereafter.
2. Experimental
2.1 Chemicals and materials
Chemicals were of analytical grade and, unless otherwise specified, obtained from SM Chemical Supplies Co. Ltd (Bangkok, Thailand) or Italmar Co. Ltd (Bangkok, Thailand). The ultrapure water for electrode cleaning and buffer preparation came from a reverse osmosis/deionization purification system and was passed through a cellulose nitrate membrane filter of pore size 0.20 μm (Whatman, GE Medical Systems Ltd, Bangkok, Thailand) and degassed before use. YKL-39 antigen and hYKL-39 antibody preparation and purification followed procedures as described in detail earlier by Ranok et al. (2013).13 Human cell lines used in this study were from the American Cell Culture Collection (Manassas, VA). Disk-shaped Au electrodes were prepared from crystalline Au rods of 3 mm diameter and 1 cm length and Au of 99.9% purity, obtained from a local gold shop (Tang Kim Heng Gold Shop, Nakhon Ratchasima, Thailand).
2.2 Capacitive hYKL-39 immunosensor preparation
Capacitive hYKL-39 immunosensors were made by a previously reported fabrication procedure.17–20 The series of mechanical and electrochemical cleaning steps, amino-thiol monolayer formation and antibody immobilization by glutaraldehyde-assisted crosslinking is illustrated in the ESI in Fig. S1.† In brief, the exposed surface of the 3 mm-diameter gold disk working electrode (Au-WE) was first freed from surface dirt and polished by intense circular rubbing on soft polishing pads soaked with alumina slurries of decreasing particle size (5, 1 and finally 0.5 micron), then 15 min ultrasonication in water and drying in a stream of inert N2 gas. Polished and dried electrodes were further electrochemically cleaned in 0.5 M H2SO4 with 25 repetitions of a 100 mV s−1 potential scan from 0 to +1.5 V vs. an Ag/AgCl reference electrode. After rinsing in water and drying with N2, the cleaned gold disks were immersed in 250 mM thiourea in water for 24 h at room temperature to allow formation of the required dense, covalently bound molecular thiol layer. This was followed by rinsing with water and drying in air in preparation for step 3, the coupling of anti-hYKL-39 to amine functionalities in the surface-anchored thiourea molecules, by glutaraldehyde-directed crosslinking. Thiolated Au-WEs were kept at room temperature for 20 min in a 5% (v/v) glutaraldehyde (GA) solution in 10 mM sodium phosphate buffer (PBS) pH 7.0. GA-treated sensors were rinsed with 10 mM PBS, pH 7.0, placed upside down in a suitable holder and 20 μL of 1 mg mL−1 anti-hYKL-39 in PBS placed on the top disk face. Antibody coupling was allowed to take place overnight at 4 °C and at completion the freshly modified sensor tips were thoroughly rinsed with PBS to remove reagent solution. Measurements of capacitive currents for hYKL-39 quantification require full insulation of the Au-WE, which is not usually achieved by simply coating with thiourea. Residual conductive gaps in the films can, however, be blocked with smaller thiols, so the anti-hYKL-39/glutaraldehyde/thiourea-modified Au-WEs were immersed for 20 min in a 10 mM solution of 1-dodecanethiol in ethanol. The success of the immunosensor preparation was checked by cyclic voltammetry of 5 mM potassium ferricyanide in 0.1 M KCl and electrochemical impedance spectroscopy (EIS) with a 5 mM mixture of ferro-/ferricyanide as redox probe in 25 mM PBS (pH 7.0).
2.3 Flow-based capacitive hYKL-39 quantification
Measurements of hYKL-39 with capacitive readout of antigen–antibody binding were executed in a microfluidic workstation as described in detail in an earlier publication17 and also by others.18–20 System modules included a three-electrode potentiostat with its software and data acquisition system (EA 163/ED410, eDAQ, Australia), a three-electrode flow cell with opposite slots for the anti-hYKL-39-modified Au-WEs and the Ag/AgCl reference electrode (RE) and stainless steel (SS) connectors for the buffer influx and outflow tubing (the outlet SS canal served also as counter electrode), a peristaltic pump (Miniplus 3, Gilson, USA) offering a constant flow of running buffer and a 6-port injection valve (Biologic MV-6, Bio-Rad, USA) for injections of μL-sized samples.
In electrochemical experiments Au-WEs with well-insulating thiol films behave as electrical capacitors; one capacitor plate is the charged Au-WE surface, the other is the layer of electrostatically attracted ions and the dielectric is the thiol film in between. The capacitor capacitance is inversely proportional to the dielectric thickness. Antibody fixation to the thiol layer and later antigen capture inserts these units between the electrode face and the ion plane forming the Helmholtz double layer, producing a thickness increase and a capacitance decrease. This effect provides a very sensitive quantification of the binding of antibody to antigen. Baseline capacitances, C, of thiol/anti-hYKL-39-modified Au-WEs and capacitance changes, ΔC, on binding of hYKL-39 to surface-anchored antibody can be quantified by the analysis of the exponentially decaying current (i) in response to repetitive 50 mV potential steps of 6.375 ms duration, before and after antigen exposure. The value of i as function of time is given by the expression:
|
ln i(t) = ln E/Rs − (1/Rs × C) × t
| (1) |
where
t is the time elapsed after the potential step was applied,
Rs the dynamic resistance of the recognition layer, and
C the total capacitance at the sensor/electrolyte interface. Linear least-squares plotting of ln
i(
t) against
t allows calculation of
Rs, from the intercept of the regression line and the known value of
E, and of
C, from the slope. While buffer was passed continuously through the electrochemical cell, a typical sample measuring cycle used a sequence of 25 potential pulses for sensor capacitance assessments, one every minute. Usually, 10 pulses were applied before and 10 after sample injection. The last 5 pulses occurred after injection of regeneration solution. For all pulses, the current response allowed computation of corresponding
C values, which, plotted
versus time, gave the baseline, the change due to sample exposure, and baseline recovery after regeneration. The measured parameter was Δ
C on hYKL-39 capture, the difference between the
C value after antigen exposure and the baseline. As shown earlier,
16–19 Δ
C scales with the concentration of injected antigen and construction of a calibration curve allows target quantification. Exposure of used hYKL-39 immunosensors to acidic regeneration buffer releases the antibody and the detector can then be reused.
Optimization trials with the capacitive hYKL-39 immunosensing procedure – for details and the data please refer to the ESI† – identified 25 mM phosphate buffer (pH 7.0), 3.2 mM HCl (pH 2.5), 100 μL min−1 and 200 μL as optimal running buffer, regeneration solution, flow rate and injected sample volume, respectively. System calibration under optimal conditions revealed the analytical dynamic range, sensitivity and detection limit. Calibrated capacitive hYKL-39 immunosensors were used to quantify analyte concentrations in Jurkat, U937 and 293t cell lysates and in synovial fluid samples that were provided by the Department of Clinical Pathology at Maharat Nakhon Ratchasima Hospital, Nakhon Ratchasima, Thailand with the informed consent of patients with OA. However, synovial fluid from healthy individuals was not available from the hospital partner for desirable controls. All experiments in this study were performed in compliance with the relevant laws and institutional guidelines of Suranaree University of Technology and permitted by its Ethics Committee for Research Involving Human Subjects, which obeys international rules in reviewing and approving procedures involving humans and animals.
2.4 ELISA assay for hYKL-39 determination
50 μL of 0.5–500 μg L−1 hYKL-39 in coating buffer (15 mM Na2CO3, 35 mM NaHCO3, pH 9.6) were loaded into standard ELISA plate wells and the protein allowed to adsorb onto the well surfaces during overnight incubation at 4 °C. Coating buffer removal was followed by 1 h of exposure of the antigen-loaded wells to blocking buffer (2% skimmed milk in PBS, pH 7.0) at 25 °C; this filled vacant protein-binding sites and prevented non-specific binding of antibody. After removal of blocking buffer wells were rinsed four times with 200 μL washing buffer (PBS with 0.05% Tween 20, pH 7.2, PBS-T) and then incubated for 1 h at 25 °C with 50 μL of anti-hYKL-39 (15 μg L−1 in blocking buffer). Wells were then washed four times with 200 μL washing buffer and incubated for further 1 h at 25 °C with 50 μL of horseradish peroxidase-conjugated goat anti-rabbit IgG (GenScript USA Inc., Piscataway, NJ), the stock (1 mg L−1) diluted 1
:
2000 in PBS, pH 7.2. Lastly, the plate was washed three times with 200 μL washing buffer. Colour was developed by addition of 100 μL 3,3,5,5-tetramethylbenzidine standard reagent solution (TMB, Invitrogen, Frederick, CA) and then 15 min dark incubation at 25 °C. The reaction was stopped by adding 100 μL of 1 N HCl. The intensity of the developed colour was determined spectrophotometrically using a Biochrome Anthos MultiRead 400 Microplate Reader (Biochem, United Kingdom) at 450 nm.
3. Results and discussion
The protein hYKL-39 is often referred to as a pseudo-chitinase since it has structural and sequence similarity to bacterial chitinases, but although it binds chitooligosaccharides it has no catalytic activity.13,21 A distinctive feature of hYKL-39 is its potential as OA biomarker, and the possible benefits of analysis of hYKL-39 in body fluids for understanding the onset and progression of OA has led to the search for hYKL-39 detection schemes. As mentioned above, ELISA is the standard means of hYKL-39 quantification. Here we explored the feasibility of hYKL-39 analysis by capacitive immunosensing, a technique that was previously shown to work well for other disease biomarkers. We first describe hYKL-39 immunosensor preparation and then discuss the parameters of the proposed capacitive immunosensor scheme for specific hYKL-39 detection. We then report hYKL-39 analysis in synovial joint fluid from OA patients and in cell lysate, which was used to prove real sample quantification capability and clinical applicability either as an independent quantification scheme or as a novel auxiliary reference tool for the validation of measures obtained by standard optical ELISA screening.
3.1 Quality checks of hYKL-39 immunosensor
Ferricyanide cyclic voltammograms (CVs) for a Au-WE at different stages of modification are shown in the ESI as Fig. S2.† At the bare gold surface, forward and backward potential scans produced symmetrical current traces, indicating reversible analyte oxidation and reduction. Decreases in the cathodic and anodic peak currents were observed after reaction of the working electrode with thiourea, then glutaraldehyde and finally anti-hYKL-39, but even after immobilization of the bulky antibody the modified Au-WE retained significant electrochemical activity. To abolish current flow completely and limit electrode activity virtually to capacitive behaviour, the sensor surface was treated in a final step with 1-dodecanethiol, a linear thiol that filled the gaps left between anchored antibody molecules and established the tight insulation required for successful operation of capacitive immunosensing. The attainment of a proper interface for capacitive immunosensing was evident in CVs showing current lines with no significant redox current waves and in EIS measurements with ferro/ferricyanide as redox probe, which produced Nyquist plots typical of coated electrodes (ESI, Fig. S3†).
3.2 Analytical performance of capacitive hYKL-39 immunosensing
Fig. 1A shows the typical response of hYKL-39 immunosensors when sample (here: 100 μg L−1 hYKL-39 in running buffer) is injected and the sensor capacitance, C, is recorded as a function of time. The two hYKL-39 additions produced well-defined stepped increases in C, indicating efficient capture of the antigen by immobilized anti-hYKL-39. Regeneration between individual injections effectively restored the baseline capacitance signal and the size of the capacitance change agreed well for the two additions. To identify the linear calibration range, hYKL-39 standard solutions of 1 ng L−1 to 10 mg L−1 were quantified by the immunosensor system, using the optimal condition parameter set. A typical calibration curve is shown in Fig. 1B, a plot of the capacitance change, normalized to the geometric sensor area, against the logarithm of hYKL-39 concentration. Triplicate measurements of all analyte concentrations showed linearity of the signal response between 0.1 and 1000 μg L−1; the linear regression line drawn by standard software followed the equation y = (16.12 ± 0.44)log(x) + (137.2 ± 2.45) with a correlation coefficient of 0.99. As expected for binding to an immunosensor surface with a fixed number of adsorbed antibody molecules, the capacitance change in response to increasing antigen concentrations reached a plateau, here at concentrations above 1 mg L−1. The limit of detection (signal-to-noise = 3) of capacitive hYKL-39 electroanalysis was 0.074 ± 0.02 μg L−1. Two hYKL-39 homologues, hYKL-40 and hAMCase (human acidic mammalian chitinase), were tested in the capacitive immunosensor system for cross-reactivity in the concentration range used for hYKL-39 (1 ng L−1 to 10 mg L−1). Neither antigen produced a significant signal change over the concentration range tested (Fig. 1B). Interference even by closely related proteins could thus be excluded.
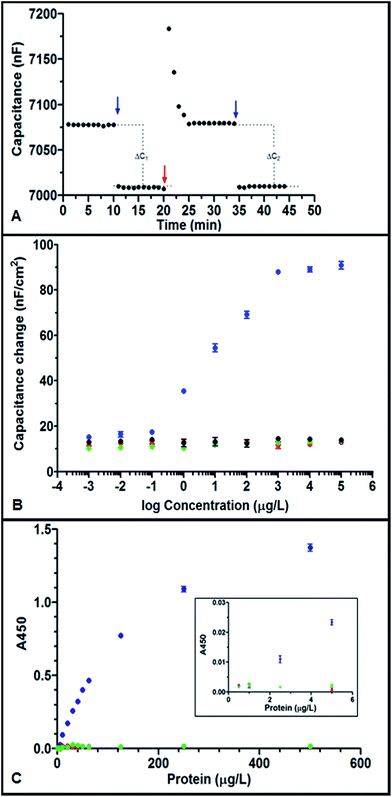 |
| Fig. 1 (A) Capacitance response of anti-hYKL-39-modified electrode. The baseline signal of the carrier buffer is initially recorded. 1st injection of 100 μg L−1 of hYKL-39 (left blue arrow) triggered antigen binding and a decrease of capacitance (ΔC1). Injection of the regeneration buffer (red arrow) removed hYKL-39 from the immunosensor surface and the capacitance returned to initial baseline. 2nd injection of 100 μg L−1 of hYKL-39 (right blue arrow) produced capacitance change ΔC2. (B) Concentration dependence of the capacitance change for a hYKL-39 immunosensor elicited by hYKL-39 (blue curve), hYKL-40 (red curve) and hAMCase (green curve) exposure. (C) Enzyme-linked immunosorbent assay (ELISA) of hYKL-39. Binding of anti-hYKL-39 IgG (50 μL, 15 μg L−1) to different amounts of immobilized hYKL-39 (blue trace), hYKL-40 (red trace) and hAMCase (green trace). The inset is a zoom of the signal response at low concentration of the antigens. | |
The specificity of the anti-hYKL-39 serum was also confirmed with a standard optical ELISA experiment. Fig. 1C shows binding of the hYKL-39 antibody to its target hYKL-39 and the reaction with hYKL-40 and hAMCase, for concentrations up to 500 μg L−1. For hYKL-39 (blue trace) triplicate recordings of the absorbance signal for the distinct biomarker levels showed linearity from 2.5–125 μg L−1, with a regression line correlation coefficient of 0.99 and pronounced levelling of the response at higher concentrations, typical of specific antibody–antigen interaction. In contrast hYKL-40 (red trace) and hAMCase (green trace) generated no signal above background level, excluding their recognition by anti-hYKL-39.
Obviously, the linearity of the electrochemical hYKL-39 screen extends at the upper and lower ends beyond those of the optical assay. The notably improved lower limit of linear response is a particular asset of the new procedure, as the OA biomarker can be quantified at concentrations beyond the reach of current ELISA. Detection closer to the onset of joint cartilage destruction thus is feasible and may permit better defect monitoring and control.
3.3 Signal stability of capacitive hYKL-39 immunosensing
Signal reproducibility and sensor stability over an extended time are crucial for analytical laboratory applications. The hYKL-39 immunosensors were therefore tested in the flow cell by monitoring, over 4 days, the capacitance changes for 57 sequential injections of 10 μg L−1 hYKL-39. At the end of each measuring cycle 200 μL of 3.2 mM HCl was injected for sensor regeneration. The stability of the sensor was assessed from plots of the capacitance change recovery ((ΔCinjection #(n+1)/ΔCinjection #n) × 100) vs. injection number. An example of such a trace is shown as ESI Fig. S11.† For 49 sample injections the regeneration treatment was sufficient to maintain the response of a hYKL-39 sensor within 95% of the capacitance change for first antigen exposure; with subsequent sample injections, however, the response declined. Apparently, the electrode coating is able to withstand the mechanical impact of the buffer flow and the cyclic variations in the chemical environment from running to regeneration buffer for several days.
3.4 Capacitive and ELISA hYKL-39 immunosensing in test samples
The measured capacitance and absorbance changes for individual model samples spiked with hYKL-39 in the concentration range 0.5–150 μg L−1 was used to derive their OA biomarker content, from pre-determined calibration curves. The averaged data from triplicate measurements of each sample, together with the equivalent information from ELISA, are listed in Table 1. Both methods offered recoveries close to 100%; however in contrast to capacitive immunosensing, ELISA failed to quantify hYKL-39 in samples at the lowest and highest analyte concentrations tested, as expected from the differences in detection limit and linear range seen in the calibration trials discussed in Section 3.2.
Table 1 Determination of hYKL-39 in model samples using capacitive immunosensor (n = 3) and standard ELISA (n = 3) quantificationa
hYKL-39 concentration (μg L−1) |
Detected hYKL-39 concentration (μg L−1) |
Immunosensor |
% recovery |
ELISA |
% recovery |
n. d. = not detected. |
0.5 |
0.5 ± 0.1 |
103.9 ± 1.4 |
n. d. |
n. d. |
2.5 |
2.6 ± 0.1 |
100.7 ± 1.1 |
2.5 ± 0.6 |
99.9 ± 2.2 |
5.0 |
5.1 ± 0.1 |
100.4 ± 3.8 |
5.1 ± 0.1 |
101.5 ± 2.6 |
50.0 |
51.2 ± 0.3 |
102.4 ± 0.6 |
49.6 ± 1.5 |
99.1 ± 3.0 |
150.0 |
149.9 ± 2.4 |
100.0 ± 1.6 |
96.4 ± 0.6 |
64.3 ± 0.4 |
3.5 Capacitive and ELISA hYKL-39 immunosensing in synovial fluid
The hYKL-39 concentrations in synovial fluids from four OA patients, donated by a local hospital, were determined by capacitive immunosensing and ELISA, in comparative trials. Both procedures were also used for hYKL-39 analysis in lysates from Jurkat, U937 and 293t cells. The choice of cells was based on the facts that Jurkat and U937 cell lysates have been reported to contain detectable levels of expressed hYKL-39 while the 293t cell line does not show hYKL-39 secretion. Jurkat and U937 lysates thus offered a physiological matrix that exposed the hYKL-39 immunosensors not only to their target but also to a mixture of other biomolecules with the potential to affect the analytical signal. Analyte-free 293t cell lysates, on the other hand, served as a useful negative control. Before biomarker quantification, samples were inspected by SDS-polyacrylamide gel electrophoresis (SDS-PAGE) and immunoblotting for hYKL-39 (Fig. 2). In each case Coomassie blue staining showed a band of the same mobility as pure hYKL-39 (Fig. 2A, lane 8) in the four synovial fluids and in Jurkat and U937 cell lysates, which was confirmed by immunoblotting (Fig. 2B). Only 293t cell lysates produced no hYKL-39 signal in the immunoblot. hYKL-39 was expected in the synovial fluid of joints from positively diagnosed OA patients; its presence in the lysates of Jurkat and U937 cells, associated with innate and adaptive immune pathways, suggests that the protein may be involved in a defensive response to inflammation, however the exact mechanisms of this involvement are not yet clear and need further studies.
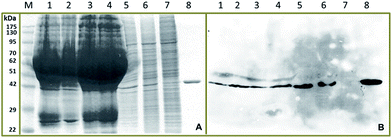 |
| Fig. 2 Clinical sample and cell lysate electrophoresis on a 10% polyacrylamide-SDS gel. Lane: M, protein markers; lanes 1–4, synovial fluid from osteoarthritis patients; lane 5, Jurkat cell lysate; lane 6, U937 cell lysate; lane 7, 293t cell lysate; lane 8, pure hYKL-39 (2 μg). (A) Coomassie-blue stained gel. (B) Immunoblot detected with anti-hYKL-39 polyclonal antibody (1 : 10 000 dilution). | |
Synovial fluids and cell lysates are complex mixtures; they were therefore diluted 10-fold with 25 mM phosphate buffer, pH 7.0 before immunosensor and ELISA analysis, in order to minimize matrix interference with signal generation. The superimposed capacitances plotted in the left part of the traces in Fig. 3, up to 10 minutes, are the baseline values before sample injection, while the capacitances plotted in the left part of the traces in Fig. 3, up to 10 minutes, are the baseline values before sample injection when the hYKL-39 immunosensor is exposed to running buffer; 200 μL aliquots of the diluted samples were then injected. In agreement with immunoblot analysis of the samples, a significant capacitance decrease was observed with all pathological synovial fluids and for the Jurkat and U937 cell lysates; synovial fluid from healthy people was not available for analysis. Injection of 293t lysate, on the other hand, led to the same small capacitance decrease as the injection of measuring buffer alone. Capacitance changes with individual samples were used to derive their content of hYKL-39, from previously determined calibration curves. The averaged data from triplicate measurements of each sample, together with the same facts from ELISA, are listed in Table 2. Regarding accuracy, the results from electrochemical hYKL-39 immunosensing were not significantly different (by t-test) from those of the ELISA immunoassay, standard deviations being small for both methods. The synovial fluid samples from the four different OA patients varied in their hYKL-39 contents, again with good agreement between electrochemical immunosensor and optical ELISA. The observed difference may be a reflection of variation in OA status of the individuals providing the samples.
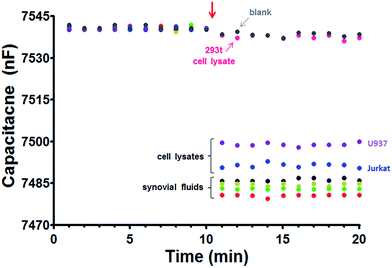 |
| Fig. 3 Cell lysate and synovial fluid analysis, by capacitive immunosensing. Sample injection is marked by a red arrow. A decrease in the sensor capacitance indicates the presence of hYKL-39, the concentration of which can be determined from the calibration curve. | |
Table 2 Determination of hYKL-39 in clinical samples and cell lysates using capacitive immunosensor (n = 3) and standard ELISA (n = 3) quantificationa
Sample |
Detected hYKL-39 concentration (μg L−1) |
Immunosensor |
ELISA |
n. d. = not detected. |
Synovial fluid #1 |
59.4 ± 2.4 |
58.3 ± 3.4 |
Synovial fluid #2 |
106.3 ± 0.8 |
107.0 ± 2.5 |
Synovial fluid #3 |
68.1 ± 1.3 |
67.7 ± 1.2 |
Synovial fluid #4 |
89.0 ± 2.7 |
88.8 ± 1.6 |
Jurkat |
26.4 ± 0.4 |
25.2 ± 3.1 |
U937 |
10.6 ± 0.3 |
9.8 ± 0.8 |
293t |
n. d. |
n. d. |
The absence of hYKL-39 from 293t cell lysates allowed generation of samples with a genuine biological matrix but ‘spiked’ with known amounts of hYKL-39, for recovery measurements. hYKL-39 concentrations of 20 and 30 μg L−1 were quantified and measurements with the capacitive immunosensor and control ELISA showed recoveries close to 100% in both cases (Table 3). Also included in Table 3 are trials with spiked synovial fluid samples. Based on a comparison with the values obtained for the non-spiked equivalents (Table 1) adequate recovery rates could be computed for the entire set of measurements and confirm the absence of confounding matrix effects. The observed competitive analytical performance in all the comparative trials reported suggest that capacitive electrochemical immunosensing is a potentially valuable option for laboratories engaged in hYKL-39-based OA analysis, either as a reference tool or, especially for real sample levels down to 10-fold below the lower limit of linear range for ELISA testing, as a stand-alone approach.
Table 3 Determination of hYKL-39 in spiked clinical samples and 293t cell lysate using capacitive immunosensor (n = 3) and standard ELISA (n = 3) quantification
Spiked concentration (μg L−1) |
Detected concentration (μg L−1) |
Immunosensor (μg L−1) |
Recovery (%) |
ELISA (μg L−1) |
Recovery (%) |
Synovial fluid #1 + 50 μg L−1 |
109.1 ± 0.1 |
99.1 ± 0.1 |
110.6 ± 1.2 |
101.1 ± 1.2 |
Synovial fluid #2 + 50 μg L−1 |
155.1 ± 1.0 |
99.2 ± 0.6 |
156.7 ± 0.8 |
99.8 ± 0.4 |
Synovial fluid #3 + 50 μg L−1 |
118.4 ± 0.6 |
100.3 ± 0.5 |
118.7 ± 1.2 |
100.8 ± 0.8 |
Synovial fluid #4 + 50 μg L−1 |
139.6 ± 0.4 |
100.4 ± 0.3 |
141.6 ± 1.2 |
102.0 ± 0.7 |
293t + 20 μg L−1 |
20.2 ± 0.4 |
100.8 ± 2.2 |
20.5 ± 0.5 |
102.4 ± 1.8 |
293t + 30 μg L−1 |
29.7 ± 0.2 |
99.1 ± 0.7 |
30.4 ± 0.5 |
101.2 ± 1.2 |
The strongest argument for choosing the electrochemical over the optical strategy is the approximately 30-fold lower detection limit, as this would facilitate recognition of osteoarthritic joint degradation close to onset of the disease, when analyte levels are expected still to be very low. Simplicity, the low cost of instrumentation, convenience in operation, the portability of the small apparatus and the feasibility of fully automated measurements are additional features that make capacitive biosensing as described herein a recommendation for in vitro hYKL-39 determinations in body fluids and cell lysates.
4. Conclusions
Attachment of an antibody directed against hYKL-39, a biomarker for osteoarthritis, to Au-WEs produced immunosensors that could measure sensitively the changes in capacitance on antibody–antigen binding, by analysis of current traces after the application of potential steps. Capacitive hYKL-39 immunosensing had a wider linear response range and, more importantly, a lower detection limit, than optical ELISA, which is currently the sole methodology for analysis of the biomarker in clinical and research laboratories. In synovial fluid from four different osteoarthritis patients the electrochemical screen detected and quantified hYKL-39 with values in accordance with those from routine ELISA. The quality of the analytical performance of this first non-optical hYKL-39 assay and the general benefits of electrochemical equipment – low cost, simplicity and ease of operation are offered together with the feasibility of automation, miniaturization, and portability – make capacitive hYKL-39 immunosensing worth further exploration, in the wide field of osteoarthritis research.
Acknowledgements
Wethaka Chaocharoen is grateful for grant CHE510767 from The Office of the Higher Education Commission, Thailand and the program “Strategic Scholarships for Frontier Research Network for the Ph.D. Program, Thai Doctoral degree”. Supporting funds came also through a Suranaree University of Technology (SUT) grant to Wipa Suginta (SUT1-102-57-24-19) and Albert Schulte (SUT1-102-57-24-01) and SUT budget allocations to the Biochemistry – Electrochemistry Research Unit and the Center of Excellence on Advanced Functional Materials. Sincere thanks go to Dr David Apps, Biochemistry Reader (retired), Centre for Integrative Physiology, Edinburgh University, Scotland for his critical manuscript reading and language improvement. We also acknowledge the support in general laboratory issues of the Biochemistry Laboratory of SUT's Center for Scientific and Technological Equipment and kind advice of Associate Professor Dr Panote Thavarungkul and colleagues from the Trace Analysis and Biosensor Research Center, Prince of Songkla University, Thailand in questions about capacitive immunosensing.
References
- S. Glyn-Jones, A. J. R. Palmer, R. Agricola, A. J. Price, T. L. Vincent, H. Weinans and A. J. Carr, Lancet, 2015, 386, 376–387 CrossRef CAS.
- S. Sovani and S. P. Grogan, Orthop. Nurs., 2013, 23, 25–36 CrossRef PubMed.
- M. Lotz, J. Martel-Pelletier, C. Christiansen, M. L. Brandi, O. Bruyère, R. Chapurlat, J. Collette, C. Cooper, G. Giacovelli, J. A. Kanis, M. A. Karsdal, V. Kraus, W. F. Lems, I. Meulenbelt, J. P. Pelletier, J. P. Raynauld, S. Reiter-Niesert, R. Rizzoli, L. J. Sandell, W. E. van Spil and J. Y. Reginster, Ann. Rheum. Dis., 2013, 72, 1756–1763 CrossRef CAS PubMed.
- F. J. Blanco, Osteoarthritis Cartilage, 2014, 22, 2025–2032 CrossRef PubMed.
- M. F. Hsueh, P. Önnerfjord and V. B. Kraus, Matrix Biol., 2014, 39, 56–66 CrossRef CAS PubMed.
- M. Ishijima, H. Kaneko and K. Kaneko, Ther. Adv. Musculoskeletal Dis., 2014, 6, 144–153 CrossRef CAS PubMed.
- S. J. Kim, Y. M. Park, B. H. Min, D. –S. Lee and H. C. Yoon, BioChip J., 2013, 7, 399–407 CrossRef CAS.
- S. Y. Hong, Y. M. Park, Y. H. Jang, B. H. Min and H. C. Yoon, BioChip J., 2012, 6, 213–220 CrossRef CAS.
- A. C. Bay-Jensen, Q. Liu, I. Byrjalsen, Y. Li, J. Wang, C. Pedersen, D. J. Leeming, E. B. Dam, Q. Zheng, P. Qvist and M. A. Karsdal, Clin. Biochem., 2011, 44, 423–429 CrossRef CAS PubMed.
- S. Gargiulo, P. Gamba, G. Poli and G. Leonarduzzi, Curr. Pharm. Des., 2014, 20, 2993–3018 CrossRef CAS.
- E. Steck, S. Breit, S. J. Breusch, M. Axt and W. Richter, Biochem. Biophys. Res. Commun., 2002, 299, 109–115 CrossRef CAS.
- T. Knorr, F. Obermayr, E. Bartnik, A. Zien and T. Aigner, Ann. Rheum. Dis., 2003, 62, 995–998 CrossRef CAS.
- A. Ranok, P. Khunkaewla and W. Suginta, Monoclonal Antibodies Immunodiagn. Immunother., 2013, 32, 317–325 CrossRef CAS PubMed.
- S. Y. Song, Y. D. Han, S. Y. Hong, K. Kim, S. Y. Yang, B. H. Min and H. C. Yoon, Anal. Biochem., 2012, 420, 139–146 CrossRef CAS PubMed.
- Y. M. Park, S. J. Kim, K. J. Lee, S. S. Yang, B. H. Min and H. C. Yoon, Biosens. Bioelectron., 2015, 67, 192–199 CrossRef CAS PubMed.
- Y. Sun, X. Hu, Y. Zhang, J. Yang, F. Wang, Y. Wang, R. Deng and G. Zhang, J. Agric. Food Chem., 2014, 62, 11116–11121 CrossRef CAS PubMed.
- W. Chaocharoen, W. Suginta, W. Limbut, A. Ranok, A. Numnuam, P. Khunkaewla, P. Kanatharana, P. Thavarungkul and A. Schulte, Bioelectrochemistry, 2015, 101, 106–113 CrossRef CAS PubMed.
- C. Berggren, B. Bjarnason and G. Johannsson, Electroanalysis, 2001, 13, 173–180 CrossRef CAS.
- C. Berggren and G. Johannsson, Anal. Chem., 1997, 69, 3651–3657 CrossRef CAS.
- W. Limbut, P. Kanatharana, B. Mattiasson, P. Asawatreratanakul and P. Thavarungkul, Biosens. Bioelectron., 2006, 22, 233–240 CrossRef CAS PubMed.
- M. Schimpl, C. L. Rush, M. Betou, I. M. Eggleston, A. D. Recklies and D. M. F. van Aalten, Biochem. J., 2012, 446, 149–157 CrossRef CAS PubMed.
Footnote |
† Electronic supplementary information (ESI) available. See DOI: 10.1039/c5ra11379b |
|
This journal is © The Royal Society of Chemistry 2015 |