DOI:
10.1039/C5RA11035A
(Paper)
RSC Adv., 2015,
5, 74783-74789
Influence of alkenyl structures on the epoxidation of unsaturated fatty acid methyl esters and vegetable oils†
Received
10th June 2015
, Accepted 27th August 2015
First published on 27th August 2015
Abstract
Epoxidation of vegetable oils or fatty acid methyl esters (FAMEs) produce important monomers which are widely used as plasticizers or stabilizers in the polymer industry. However, little attention has been focused on the influence of the alkenyl structure of the fatty acid on the efficiency and selectivity of their epoxidation. In this work, the influence of the alkenyl structure (the number of double bonds) of the FAMEs on the epoxidation reaction has been investigated. Three model FAMEs with 1 to 3 double bonds were epoxidized using both a weak (formic acid) and a strong (sulfuric acid/acetic acid) acid system. It was found that FAMEs with more double bonds have higher reactivities toward the epoxidation reaction. In addition, the electron-donating effect of the double bonds on the fatty acid chain tends to stabilize the resulting epoxide adjacent to it with the weak acid system. Furthermore, FAMEs with more double bonds easily undergo side reactions with the strong acid system (H2SO4). Epoxidation of two vegetable oils with different fatty acid compositions were carried out with the same two acid catalyst systems. And the results were in agreement with those from the FAMEs. The current findings could provide useful guidance for the epoxidation of different vegetable oils with different alkenyl structure compositions.
1 Introduction
The reliance on fossil feedstocks for the production of fuels and chemicals has led to an energy shortage all over the world, as a consequence of diminishing fossil resources and high energy demand. Moreover, CO2 emission from fossil fuels has also raised global warming problems and environmental pollution. Renewable biomass has been considered as a potential candidate to solve this problem due to its large annual production and rich carbon nature.1 Among different biomass resources, vegetable oils are complex mixtures of triglycerides, which consist of a glycerol backbone and three fatty acid moieties (saturated or unsaturated).2 They are renewable, inherently biodegradable, eco-friendly and inexpensive to serve as the alternative sources to fossil feedstock for producing chemicals or fuels.3
Chemical modifications of vegetable oils are important methods to obtain industrial products.4 Transesterification of vegetable oils provides the first generation of biodiesel fuels which have already been industrialized for years.5 Besides, modification of unsaturated C
C bonds in the hydrocarbon chain of fatty acids to produce new value-added chemicals or monomers for polymers has also attracted considerable interest for many years. One of the most common approaches is the epoxidation of vegetable oils or their fatty acid esters (FAMEs) which are useful intermediates or compounds that can serve as plasticisers,6 resins,7 stabilizers in coatings8 and biofuel additives.9
Epoxidation of vegetable oils is usually carried out with peroxyacid formed in situ from organic carboxylic acid (e.g. acetic acid) and hydrogen peroxide over an acidic catalyst (e.g. mineral acids) which is known as the Prileshaiev process (Fig. 1).3 For example, Goud10 and Okieimen11 et al. reported the epoxidation of Karanja oil and rubber seed oil with hydrogen peroxide and peroxyacetic acid over sulfuric acid catalysts. As well as sulfuric acid (H2SO4), hydrochloric acid (HCl), nitric acid (HNO3) and phosphoric acid (H3PO4) were also used as catalysts for the epoxidation of vegetable oils.12 The main drawbacks of the Prileshaiev process is that the resulted epoxides can easily undergo ring-opening reactions due to the instability of the epoxide group under acidic conditions.13 Hence, tremendous research efforts have been devoted to the development of new technologies to improve the efficiency and suppress the ring-opening reactions, typically involving the exploration of new catalysts and optimization of the reaction conditions. Heterogeneous solid acid catalysts such as acidic resin,14 Ti–silicas,15 Nb(V)–silica,16 sulfated-SnO2,17 polyoxometalate18 were developed for the reaction. These advances have further broadened the choice of catalytic systems for the epoxidation of vegetable oils.
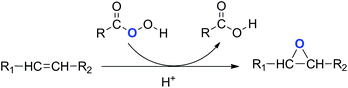 |
| Fig. 1 The main reaction route of epoxidation. | |
Oleic (C18:1), linoleic (C18:2) and linolenic (C18:3) acids are three typical unsaturated fatty acids in vegetable oils that contain 1 to 3 double bonds. During our study on the epoxidation of vegetable oils, we noted that vegetable oils containing more linolenic fatty acid composition tend to give inferior epoxidation results (low epoxides yield and complex side products). Vegetable oils from various plants contain different fatty acid compositions including these three fatty acids. Thus, it's important to find out general principles of the unsaturated fatty acid epoxidation regarding the relationship between the unsaturated alkenyl structure and their epoxidation efficiency. This would help to design more efficient catalytic systems for the epoxidation of vegetable oils with different fatty acids composition. However, to the best of our knowledge, there is little in the literature related to the influences of the alkenyl skeleton with different numbers of C
C bonds on the efficiency and product selectivity of the epoxidation of vegetable oils.
Herein, we reported the epoxidation of three unsaturated fatty acid methyl esters (methyl oleate, methyl linoleate, methyl linolenate) derived from vegetable oils to investigate the influences of the alkenyl structure on the epoxidation reaction. From the practical point of view, the two most commonly used liquid acids catalysts of different acid strength, sulfuric acid (SA) and formic acid (FA), were selected as the catalysts in this study aiming to provide some insightful information on the common methods of the epoxidation of vegetable oils. Different analysis methods, iodine value, oxirane content, FTIR, 1H-NMR and ESI-MS, were used to characterize the epoxidation products. Finally, two vegetable oils with different alkenyl fatty acids compositions were epoxidized with the aforementioned acid catalyst systems to further verify the results from the epoxidation of the FAMEs.
2 Experimental
2.1 Materials
Methyl oleate (MO, Sigma-Aldrich, 99%), methyl linoleate (ML, TCI, >95%) and methyl linolenate (MLN, Sigma-Aldrich, ≥99%) are the model compounds used for this experiment. Glacial acetic acids (AR), formic acids (AR), hydrogen peroxide (30 wt%) and sulfuric acid (98%) were purchased from Nanjing Chemical Reagent Co., Ltd. Wij's solution was bought from Tianjin weiyi Chemical Technology Co., Ltd. Olive oil and linseed oil were purchased from local market. All other reagents used were purchased from local chemical reagent company and used without further purification.
2.2 Epoxidation of FAMEs and vegetable oils
A typical procedure for the epoxidation of FAMEs was as follows: 4 mmol methyl oleate, 6 mmol HCOOH (or 0.41 mmol H2SO4, 6 mmol CH3COOH) were firstly added to a 100 mL three necked round-bottom flask equipped with a magnetic stirrer. The flask was then purged with nitrogen to exclude air and placed in a temperature controlled oil bath. The mixture was then stirred and heated to 30 °C. Then, 21 mmol H2O2 (30 wt%) was added, dropwise, to the reaction at a slow rate to maintain the temperature of the reaction system within 30 ± 1 °C. The whole reaction time was 5 h. Upon the end of the reaction, the reaction mixture was extracted twice with ethyl acetate (2 × 20 mL). The organic phase was washed with saturated sodium bicarbonate (3 × 15 mL) and saturated sodium chloride (3 × 15 mL) for three times, respectively. The resulting organic phase was then dried with anhydrous magnesium sulfate. After filtration, the solvent ethyl acetate was removed by vacuum evaporator.
The epoxidation of vegetable oils were followed the same procedures as the epoxidation of FAMEs except that the amount of reagents used were as follows: 3 g olive oil or linseed oil reacted with 0.6 g (13 mmol) FA (or 0.06 g (6.1 mmol) SA, 0.79 g (13.1 mmol) AA) and 5.19 g (∼46 mmol) H2O2 (30 wt%).
Silica coated aluminium thin layer chromatography (TLC) plates were used for separating the reaction mixtures. The eluent was a mixture of petroleum ether and ethyl acetate in 25
:
1 (vol/vol) ratio for epoxidized methyl oleate and in 10
:
1 (vol/vol) ratio for epoxidized methyl linoleate. The chromogenic reagent was potassium permanganate solution.
Iodine values (IVO) of the FAMEs and vegetable oils were expressed as gram per 100 gram sample (g per 100 g) and were determined according to Wij's method.19 The amount of oxirane oxygen (OO) was measured by the method with hydrobromic acid in acetic acid solution.20
The theoretical percentage of oxirane oxygen (OOthe) in 100 g sample was determined by the following expression.21
where
AI (126.9) and
AO (16.0) are the atomic weights of iodine and oxygen, respectively and IV
O is the initial iodine value of the samples before epoxidation.
The conversion of substrate (C) of the epoxidation substrate was determined as follows:22
where IV
O is the initial iodine values of the samples before epoxidation, IV
ae is the iodine values of substrate after epoxidation.
The yield of epoxidized products (Y) was calculated as follows. (Y):22
where OO
exp was the actual percentage of the oxirane oxygen obtained in the experiment.
The amount of the ring-opening products and all other unidentified products from side reactions was determined as the difference between the conversion of the epoxidation reaction and the yield of the epoxidized products (C−Y).
2.3 Identification of reaction products
The resulting reaction mixtures were analyzed by gas chromatography-mass spectroscopy (GC-MS) and GC. The GC-MS (Agilent 7890A, Agilent 5975C MSD) and GC (Agilent 7890A) instruments were equipped with a DB-5 capillary column (30 m × 0.25 mm × 0.25 μm, Agilent). The temperature of column increased from 200 °C to 250 °C at a 5 °C min−1 rate. The temperature of the injector was 250 °C. Sample was diluted in ethyl acetate prior to injection.
The separated products from TLC were characterized by the nuclear magnetic resonance (NMR, BruckerAvance III 600 Hz) and high resolution mass spectroscopy (MS, Bruker VPEXII) with EI mode.
Fourier transform infrared spectroscopy (FT-IR) spectra were obtained by using Thermo Nicolet 380 coupled with Ezominic software. Sample was smeared as a thin layer on KBr plates. Then IR analysis was implemented between frequency range 4000–400 cm−1.
3 Results and discussion
3.1 Epoxidation of three FAMEs
The epoxidation reactions of three unsaturated FAMEs (MO, ML and MLN) were carried out using H2O2 as the oxidant over SA and FA catalysts. The results of the epoxidation were listed in Table 1. It should be noted that unlike acetic acid (AA) in the SA-catalysed system, FA serviced as both the acid catalyst and the active oxygen carrier for the epoxidation in the FA-catalysed system. The epoxidation results exhibited obvious trends as the number of double bonds varied in the FAMEs with the FA catalysed system. The IVO increased with the increasing number of double bonds in the FAMEs' skeleton, as expected. The conversion of the double bonds in MO, ML, and MLN were 66.21%, 74.84% and 75.35%, respectively. Both the OOexp values and the yield of the epoxidized FAMEs increased from 3.50 to 8.57 and from 50.51% to 69.45% as the number of double bonds increased from 1 to 3 in the starting materials, respectively. It is well known that the epoxidation reaction is always associated with side reactions, such as hydroxylation, oxidation, oxygenation, and dimer formation.13 It is worth noting that the yield of the side products (C−Y) decreased substantially as the number of the double bonds increased. It has been reported that during the epoxidation of alkenes, the electron-donating groups adjacent to the alkenyl groups could enhance the activity of the alkenyl group toward the epoxidation, and moreover, stabilize the resultant epoxides from decomposition.23 Similarly, it can be speculated that FAMEs with more double bonds would have higher reactivities due to the electron-donating effects from the adjacent carbon and double bond. In addition, when one of the double bonds converted to epoxides, the adjacent double bond and the carbon in the middle (an allyl group) served as a weak electron-donating group, which would help to promote the efficiency of the reaction and to stabilize the resulting epoxides.
Table 1 Epoxidation of FAMEs with two acidsa
Entry |
Systems |
Substrate |
IVO (g/100 g) |
IVae (g/100 g) |
C (%) |
OOthe (%/100 g) |
OOexp (%/100 g) |
Y (%) |
C−Y (%) |
Conditions: (C C) : FA/AA : H2O2 = 1 : 1.5 : 5.25 (molar ratio), 30 °C, 5 h. 2 wt% SA. FA: formic acid, SA: H2SO4, MO: methyl oleate, ML: methyl linoleate, MLN: methyl linolenate. IVo: iodine values. IVae iodine values after epoxidation. C: conversion of substrate. OOthe theoretical percentage of oxirane oxygen. OOexp experimental percentage of oxirane oxygen. Y: the yield of epoxidized products. C−Y: others. |
1 |
FA |
MO |
118.13 |
39.93 |
66.21 |
6.93 |
3.50 |
50.51 |
15.70 |
2 |
ML |
173.62 |
43.69 |
74.84 |
9.87 |
6.39 |
64.74 |
10.10 |
3 |
MLN |
223.36 |
55.06 |
75.35 |
12.34 |
8.57 |
69.45 |
3.66 |
4 |
SA/AAb |
MO |
118.13 |
48.92 |
58.59 |
6.93 |
2.96 |
42.71 |
15.88 |
5 |
ML |
173.62 |
54.32 |
68.71 |
9.87 |
3.30 |
33.43 |
35.28 |
6 |
MLN |
223.36 |
48.65 |
78.22 |
12.34 |
0.78 |
6.32 |
71.90 |
Table 2 Epoxidation of vegetable oils with different fatty acid compositionsa
Oil |
Systems |
IVO (g/100 g) |
IVae (g/100 g) |
C (%) |
OOthe (%/100 g) |
OOexp (%/100 g) |
Y (%) |
Conditions: (C C) : FA/AA : H2O2 = 1 : 0.8 : 2.8 (molar ratio), 50 °C, 2 h. 1 wt% SA was added. |
Olive oil |
FA |
127.18 |
36.21 |
71.53 |
7.42 |
5.03 |
67.79 |
SA/AAb |
|
32.02 |
74.82 |
|
4.07 |
54.85 |
Linseed oil |
FA |
200.61 |
50.17 |
74.99 |
11.23 |
6.68 |
59.48 |
SA/AAb |
|
38.64 |
80.74 |
|
2.06 |
18.34 |
The epoxidation of FAMEs with the SA/AA system often showed significant differences from those of the FA system. The conversions of the unsaturated double bonds were similar to that from the FA-catalyzed reaction, indicating the increasing activities of the FAMEs with the increasing double bonds. However, the OOexp value and the yield of the epoxidized FAMEs displayed the opposite trends to those from the FA system. They both decreased sharply as the number of double bonds increased, especially with the epoxidation of MLN. The OOexp value and yield of epoxidized MLN were as low as 0.78 and 6.32, respectively, while these values were 8.57 and 69.45 with the FA system. In addition, all three FAMEs showed less efficient epoxidation with the SA/AA system than that with the FA system. Similar results have been reported in the literature which attributed the low epoxides yield to the strong acidic nature of SA, that could lead to severe side reactions when epoxidized FAMEs contained more epoxy groups.24 The above results indicated that the alkenyl group with different numbers of double bonds may have important influence on the epoxidation efficiency and product selectivity. To further investigate the mechanism of the epoxidation of FAMEs with different numbers of double bonds, the epoxidation reaction of the above three FAMEs were tracked and the reaction products were identified with IR, MS and NMR to evaluate the influences of these alkenyl groups on the efficiency and selectivity of the epoxidation reaction.
3.2 Tracking of epoxidation reaction
3.2.1 Epoxidation of methyl oleate. The epoxidation process of MO with one double bond in the skeleton of the fatty acid was tracked by GC and the results were shown in Fig. 2. The spectrum on the left was the epoxidation catalyzed by FA while the right was SA. After the first 1 h, three major peaks were detected in both systems and the peaks were identified by GC-MS. Peak 1 was the starting material MO (Fig. S1 and S4†). Peak 2 was the target epoxidized product (Fig. S2 and S5†). And peak 3 was fatty ketones (Fig. S3 and S5†) which were result of the rearrangement of the epoxidized product, as reported in much literature.25 As the reactions proceeded, the amount of MO gradually reduced in both systems. About 39.93% and 48.92% of the double bond remained in the FA and SA/AA systems, respectively, after 5 h (Table 1, entry 1 and 4). Meanwhile, the amounts of the epoxidized products increased gradually and reached 50.51% and 42.71% yield in the FA and SA/AA systems, respectively. Comparing the two acid catalysed systems, it can be seen that the FA system had a higher reaction rate in the formation of epoxidized products than the SA system. This could be attributed to the higher rate constant of the in situ formation of the peroxyacidin the FA system than in the SA/AA system, which was the rate-determining step of the epoxidation reaction.26 The IR spectra of the reaction mixture is shown in Fig. 3. For the starting material MO (spectrum a), the peak at 3004 cm−1 was attributed to the C
C double bond in the fatty acid skeleton. It became a small shoulder in the product mixture after the epoxidation in both systems, which implied that most double bonds were converted while only a few remained unreacted (spectrum d and g). The peak around 820–850 cm−1 represented the oxirane ring of the epoxides and it may be slightly changed due to different functional groups adjacent to the oxirane group as reported in many literature.27 No obvious peak around 3400 cm−1 (–OH stretching peak) was detected in d and g, which indicated that the ring-opening side diol products were negligible with the epoxidation of MO in both systems. Based on the above results, it can be concluded that both FA and SA/AA system were effective for the epoxidation of the FAMEs with a single double bond and gave similar performances in the reactions, albeit a little difference in the conversions and product yields.
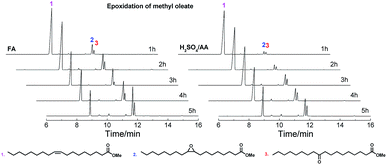 |
| Fig. 2 GC traces for the epoxidation of methyl oleate with FA and SA/AA. | |
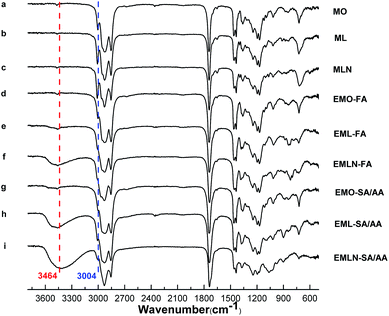 |
| Fig. 3 FT-IR patterns of MO, ML, MLN, epoxidation of FAMEs with FA (EMO-FA, EML-FA, EMLN-FA) and SA/AA (EMO-SA/AA, EML-SA/AA, EMLN-SA/AA). | |
3.2.2 Epoxidation of methyl linoleate. Fig. 4 showed the tracking of the epoxidation reaction of ML over FA and AA catalytic systems. Two major products were detected by GC, however, whose molecular ions cannot be detected by GC-MS. Thus, these two products were separated by TLC and the separated products were analyzed by 1H-NMR and MS (see ESI†). According to the results, peak 4 was the starting material (Fig. S6†) and it gradually reduced as the reaction proceeded. Peak 5 was a doublet peak corresponding to the isomerized epoxidized product with only one epoxy group (Fig. S7 and S9†). Peak 6 was also a doublet peak corresponded to the isomerized epoxidized products with two epoxy groups (Fig. S8 and S10†). Peak 7 was the side products that cannot be identified. The amount of the epoxidized products (peak 5) gradually increased as the reaction proceeded. When the reaction was stopped after 5 h, the FA system gave more epoxidized products than that from SA system. As listed in Table 1, the conversion percentages of the double bonds in ML were similar in the two acid catalytic systems (74.84% and 68.71%), however much fewer of the epoxidized products (peak 5) were detected by GC in the SA/AA system than in the FA system. This is due to the severer ring-opening reaction present in the epoxidized products in the SA/AA system than in the FA system. Further confirmation via IR analysis of the product mixture is shown in Fig. 3, the SA/AA system provided a stronger –OH stretching peak around 3464 cm−1 compared to the FA system, thus indicating the epoxidized products with two epoxy group easily underwent ring-opening and formed –OH in the presence of strong acid (Fig. 3, h). Meanwhile, these epoxidized products were more stable in the weak acid FA system and the –OH stretching peak in the IR spectrum (Fig. 3, e) were relatively weak.
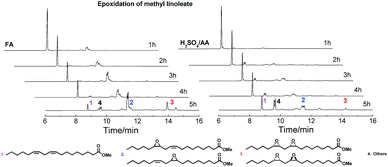 |
| Fig. 4 GC traces for the epoxidation of methyl linoleate with FA and with SA/AA. | |
3.2.3 Epoxidation of methyl linolenate. The epoxidation of MLN was carried out with the same two acid catalytic systems. As shown in Fig. 5, the results from the tracking of the reaction exhibited remarkable differences between the two acids systems. When SA was used, two groups of product peaks (9 and 10) were detected by GC in the FA reaction system. However, they were difficult to separate by TLC. Therefore, these two groups of products were submitted to MS analysis. The molecular ion peak at m/z 331.23 [M + Na] corresponded to mono-epoxidized product 9 and the molecular ion peak at m/z 347.22 [M + Na] corresponded to di-epoxidized product 10 (Fig. S11†). Both of the two groups of products had three isomers, thus they gave multiplet peaks in the GC spectrum. On the other hand, no obvious product peaks were detected in the SA/AA system, which indicated that most of the epoxidized products might be further converted to ring-opening products, such as polyols, that cannot be detected by GC due to their high polarity. IR analysis of the reaction products of MLN confirmed the above speculation by which the peak intensity around 3464 cm−1in the spectrum of epoxidized MLN with SA/AA system (Fig. 3, i) was stronger than those of all other reaction products. The peak of the epoxy group (820–850 cm−1) was very weak which is consistent with the lower OOexp in Table 1, entry 6. These results revealed that FAMEs with three double bonds easily underwent ring-opening reactions over strong acid catalysts than FAMEs with two or single double bonds. However, when the weak acid FA was used, the side reactions were greatly suppressed and more epoxides were obtained.
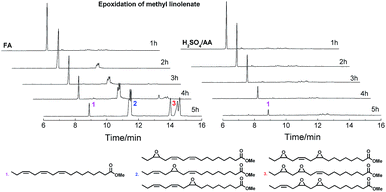 |
| Fig. 5 GC traces for the epoxidation of methyl linolenate with FA and SA/AA. | |
3.2.4 The influences of the structure on the epoxidation. From the above results, it was found that the structure of FAMEs (different number of double bonds) had a significant influence on the efficiency of their epoxidation reactions. The percentage conversions of the double bonds increased as the number of double bonds on the fatty acid skeleton increased, regardless which acid catalysts was used (from 66.21% to 75.35% in FA system and 58.59% to 78.22% in SA/AA system), indicating the FAMEs with more unsaturated bonds exhibited higher reactivities towards epoxidation. This phenomenon can be ascribed to the electron-donating effect from the adjacent double bonds. It has been reported in the literature that epoxidation can be promoted by electron-donating groups adjacent to the double bonds.23 In addition, the generated epoxy group can also be stabilized by the adjacent electron-donating groups.23b In the current systems, ML and MLN had two or three double bonds on their fatty acid skeletons. When one double bond underwent epoxidation, the nearest double bond and the carbon between them can be viewed as an allyl group which was a weak electron-donating group (Fig. 6). Therefore, FAMEs with more double bonds would have higher activities when undergoing epoxidation reaction either with a strong or weak acid catalyst.
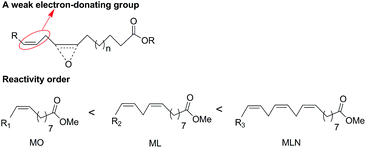 |
| Fig. 6 Electron effect and reactivities of different FAMEs. | |
Furthermore, the strength of the acid played an important role in the final product distributions of the epoxidation of the FAMEs. When FA was used as the catalyst and oxygen-carrier, the percentage conversion of double bonds and the yield of epoxidized products increased as the number of double bonds increased (Table 1, entries 1–3). However, when SA was used as the catalyst and AA was the oxygen-carrier, the conversion of double bonds still increased while the yield of the epoxidized products decreased sharply. The strong acid SA caused severe ring-opening reactions during this process, especially when the products had two or three epoxy groups (Table 1, entries 5 and 6).
Based on the above results, it can be concluded that FAMEs with more double bonds had higher activities towards epoxidation (MLN > ML > MO) in both acid catalytic systems (Fig. 6). Nevertheless, the weak acid FA can be used in the epoxidation of all three FAMEs with different double bonds while the strong acid SA was more suitable for the epoxidation of FAMEs with a single double bond.
3.3 Epoxidation of vegetable oils
Two vegetable oils, olive oil and linseed oil, with different fatty acid compositions were chosen for epoxidation to confirm the effect of fatty acid structures on the efficiency of their epoxidation reaction (Table 2). The olive oil mainly contains oleic and linoleic acid (∼63.7% and ∼25.2%, respectively) with almost no linolenic acid. Linseed oil has a composition of oleic acid ∼22.1%, linoleic acid ∼16.7%, and linolenic acid ∼52.5%. When olive oil was submitted to epoxidation reaction, the percentage conversions of the double bonds were 71.53% and 74.82% in the FA and SA/AA system, respectively. While the yield of epoxidized products in the two acid systems were 67.79% (FA) and 54.85% (SA/AA). The FA system gave a little higher product yield than the SA/AA system. The linoleic acid composition in olive oil should be the key factor for the side reactions in the SA/AA system. When linseed oil was used as the starting material, the conversions of the double bonds were higher than that from olive oil, resulting from the higher reactivity of the linolenic acid in linseed oil. The yield of the epoxidized products from linseed oil with the FA system was still at a high level (59.48%), which indicated that side reactions were suppressed to a minimum level in the weak acidic systems. However, the epoxidation of linseed oil with the SA/AA system gave a poor yield of epoxidized products. All of the above results were consistent with the results from the epoxidation of FAMEs. Therefore, it can be concluded that a weak acidic system such as FA was more preferable for the epoxidation reaction when the vegetable oil contained more linolenic acid or linoleic acid.
4. Conclusions
Three typical unsaturated FAMEs (methyl oleate, methyl linoleate and methyl linolenate) were used in the epoxidation reaction with FA and SA/AA systems to investigate the influences of the alkenyl structure with different numbers of double bonds on the epoxidation efficiency and selectivity. The epoxidation reactions were tracked and the products were analyzed by various technologies. It was found that FAMEs with more double bonds have higher reactivities towards the epoxidation reaction with both acid catalysts, which may be due to the electron-donating effect of the adjacent double bonds. In addition, epoxidized FAMEs with more double bonds can easily undergo side reactions in a strong acidic system (SA/AA) and lead to a lower oxirane oxygen value. Alternatively, a weak acid (FA) exhibited better performance in the epoxidation of the three FAMEs and the side reactions were greatly suppressed in this weak acidic system. Finally, the epoxidation results of the two vegetable oils with different fatty acid compositions further confirmed the above conclusions. The current findings provide useful information on the influences of the alkenyl structure on the epoxidation reactions which may find important application in the design of new catalytic systems for the epoxidation of vegetable oils with different fatty acid compositions. Future studies will be focused on the development of some heterogeneous catalysts for the epoxidation of vegetable oils.
Acknowledgements
The authors are grateful for the financial support by the Jiangsu Specially-Appointed Professor program of the State Minister of Education of Jiangsu Province and the start up foundation of NJFU (GXL003).
Notes and references
-
(a) T. E. Bull, Science, 1999, 285, 1209 CrossRef CAS;
(b) A. J. Ragauskas, C. K. Williams, B. H. Davison, G. Britovsek, J. Cairney, C. A. Eckert, W. J. Frederick Jr, J. P. Hallett, D. J. Leak, C. L. Liotta, J. R. Mielenz, R. Murphy, R. Templer and T. Tschaplinski, Science, 2006, 311, 484 CrossRef CAS PubMed.
- Z. S. Petrović, Polym. Rev., 2008, 48, 109 CrossRef.
-
(a) S. G. Tam and W. S. Chow, Polym.-Plast. Technol. Eng., 2010, 49, 1581 CrossRef;
(b) K. Dalai, Ind. Eng. Chem. Res., 2014, 53, 18668 CrossRef.
-
(a) A. Corma, S. Iborra and A. Velty, Chem. Rev., 2007, 107, 2411 CrossRef CAS PubMed;
(b) M. Besson, P. Gallezot and C. Pinel, Chem. Rev., 2014, 114, 1827 CrossRef CAS PubMed.
-
(a) R. Mythili, P. Venkatachalam, P. Subramanian and D. Uma, Int. J. Energy Res., 2014, 38, 1233 CrossRef;
(b) N. Narkhede, S. Singh and A. Patel, Green Chem., 2015, 17, 89 RSC;
(c) Z. Helwani, M. R. Othman, N. Aziz, W. J. N. Fernando and J. Kim, Fuel Process. Technol., 2009, 90, 1502 CrossRef CAS;
(d) L. Soh, J. Curry, E. J. Beckman and J. B. Zimmerman, ACS Sustainable Chem. Eng., 2014, 2, 387 CrossRef CAS.
-
(a) H. Schuster, L. A. Rios, P. P. Weckes and W. F. Hoelderich, Appl. Catal., A, 2008, 348, 266 CrossRef CAS;
(b) J. Salimon, N. Salih and E. Yousif, Eur. J. Lipid Sci. Technol., 2010, 112, 519 CAS;
(c) J. O. Metzger, Eur. J. Lipid Sci. Technol., 2009, 111, 865 CrossRef CAS.
- M. T. Benaniba, N. Belhanceche-Bensemra and G. Gelbard, Polym. Degrad. Stab., 2003, 82, 245 CrossRef CAS.
-
(a) J. A. Sherringham, A. J. Clark and B. R. T. Keene, Lipid Technol., 2000, 12, 129 CAS;
(b) S. P. Bunker and R. P. Wool, J. Polym. Sci., Part A: Polym. Chem., 2002, 40, 451 CrossRef CAS.
- B. K. Sharma, K. M. Doll and S. Z. Erhan, Green Chem., 2007, 9, 469 RSC.
- V. V. Goud, N. C. Pradhan and A. V. Patwardhan, J. Am. Oil Chem. Soc., 2006, 83, 7 CrossRef.
- F. E. Okieimen, O. I. Bakare and C. O. Okieimen, Ind. Crops Prod., 2002, 15, 139 CrossRef CAS.
- S. Dinda, A. V. Patwardhan, V. V. Goud and N. C. Pradhan, Bioresour. Technol., 2008, 99, 3737 CrossRef CAS PubMed.
-
(a) A. Campanella and M. A. Baltanás, Lat. Am. Appl. Res., 2005, 35, 205 CAS;
(b) A. Campanella and M. A. Baltanás, Lat. Am. Appl. Res., 2005, 35, 211 CAS.
-
(a) S. Dinda, V. V. Goud, A. V. Patwardhan and N. C. Pradhan, Asia-Pac. J. Chem. Eng., 2011, 6, 870 CrossRef CAS;
(b) A. Campanella and M. A. Baltanás, Chem. Eng. Process., 2007, 46, 210 CrossRef CAS.
-
(a) A. Campanella, M. A. Baltanás, M. C. Capel-Sánchez, J. M. Campos-Martín and J. L. G. Fierro, Green Chem., 2004, 6, 330 RSC;
(b) L. A. Rios, P. Weckes, H. Schuster and W. F. Hoelderich, J. Catal., 2005, 232, 19 CrossRef CAS;
(c) D. Kumar and A. Ali, Energy Fuels, 2012, 26, 2953 CrossRef CAS;
(d) M. Guidotti, E. Gavrilova, A. Galarneau, B. Coq, R. Psaro and N. Ravasio, Green Chem., 2011, 13, 1806 RSC.
- C. Tiozzo, C. Bisio, F. Carniato, L. Marchese, A. Gallo, N. Ravasio, R. Psaro and M. Guidotti, Eur. J. Lipid Sci. Technol., 2013, 115, 86 CrossRef CAS.
- A. K. R. Somidi, R. V. Sharma and A. K. Dalai, Ind. Eng. Chem. Res., 2014, 53, 18668 CrossRef CAS.
- Y. Leng, J. Zhao, P. Jiang and J. Wang, ACS Appl. Mater. Interfaces, 2014, 6, 5947 CAS.
- C. Paquot. Standard Methods for the Analysis of Oils, Fats and Derivatives Part I, Pergamon, Oxford, United Kingdom, 6th edn, 1979 Search PubMed.
- C. Paquot. Standard Methods for the Analysis of Oils, Fats and Derivatives Part -1, Pergamon Press, Germany, 1979 Search PubMed.
- A. P. Chavan and P. R. Gogate, J. Ind. Eng. Chem., 2015, 21, 842 CrossRef CAS.
-
(a) E. Milchert, A. Smagowicz and G. Lewandowski, J. Chem. Technol. Biotechnol., 2010, 85, 1099 CrossRef CAS;
(b) E. Milchert, A. Smagowicz and G. Lewandowski, Org. Process Res. Dev., 2010, 14, 1094 CrossRef CAS.
-
(a) W. Zheng, R. Tan, L. Zhao, Y. Chen, C. Xiong and D. Yin, RSC Adv., 2014, 4, 11732 RSC;
(b) A. H. Hoveyda, D. A. Evans and G. C. Fu, Chem. Rev., 1993, 93, 1307 CrossRef CAS.
-
(a) A. Campanella, C. Fontanini and M. A. Baltanás, Chem. Eng. J., 2008, 144, 466 CrossRef CAS;
(b) M. Rüsh, G. Klaas and S. Warwel, ed. G. Knothe and J. T. P. Derksen, New Oxidation Methods for Unsaturated Fatty Acids, Esters, and Triglycerides, Recent Developments in the Synthesis of Fatty Acid Derivatives, AOCS Press, Champaign, IL, 1999, p. 157 Search PubMed.
-
(a) L. A. Rios, B. A. Llano and W. F. Hoelderich, Appl. Catal., A, 2012, 445, 346 CrossRef;
(b) R. O. C. Norman and J. M. Coxon, Principles of Organic Synthesis, 1993, 3, 590 Search PubMed.
- L. H. Gan, S. H. Goh and K. S. Ooi, J. Am. Oil Chem. Soc., 1992, 69, 347 CrossRef CAS.
-
(a) N. Kim, Y. Li and S. Sun, Ind. Crops Prod., 2015, 64, 1 CrossRef CAS;
(b) P. K. Gamage, M. O'Brien and L. Karunanayake, J. Natn, Sci. Foundation Sri Lanka, 2009, 37, 229 CAS.
Footnotes |
† Electronic supplementary information (ESI) available. See DOI: 10.1039/c5ra11035a |
‡ These authors equally contributed to the work. |
|
This journal is © The Royal Society of Chemistry 2015 |