DOI:
10.1039/C5RA10781D
(Paper)
RSC Adv., 2015,
5, 68668-68675
Hydrothermal synthesis of gold polyhedral nanocrystals by varying surfactant concentration and their LSPR and SERS properties†
Received
7th June 2015
, Accepted 3rd August 2015
First published on 6th August 2015
Abstract
Several polyhedral gold nanocrystals (Au NCs) whose geometric morphology belongs to the Platonic solid and Archimedean solid groups are synthesized by modulating the cetyltrimethylammonium bromide (CTAB) concentration in the designed hydrothermal processes. The mechanism of growth of these polyhedral Au NCs is revealed via crystallographic analyses, which suggest that their morphologies mainly depend on the diminishing of the surface energy of the specific facets in the CTAB-directive shape-control process. Furthermore, the localized surface plasmon resonance (LSPR) properties of the as-prepared polyhedral Au NCs are numerically assessed using the finite element method (FEM). The calculations show that these polyhedral Au NCs exhibit geometry-dependence plasmonic properties: specifically, the enormous local field enhancements occur around their vertices and corners. The SERS spectra of 4-mercaptobenzoic acid molecules adsorbed on these polyhedral Au NCs display superior SERS activity with an enhancement factor of 105 to 106 at the excitation wavelength of 785 nm. Particularly, the truncated tetrahedron and bitetrahedron show higher SERS enhancement factors due to their LSPR being closer to the excitation wavelength of 785 nm. Thus, the facile surfactant-assisted synthesis offers the opportunity to design and optimize the shape-dependent SERS activity of polyhedral Au NCs with respect to possible applications in bio-sensing and imaging.
1. Introduction
Noble metal nanocrystals (NCs) have attracted enormous attention and widespread research interest due to their unique chemical and physical properties and potential applications.1–6 Especially, the localized surface plasmon resonance (LSPR) property is one of the current prevalent issues, owing to the intriguing optical characteristics arising from the collective oscillation of electrons in the conduction band of metals upon laser irradiation which leads to electromagnetic field enhancement of several orders of magnitude.3,7,8 As the most famous practical consequence of the plasmonic coupling effect,9 the surface enhanced Raman scattering (SERS) technology has been implemented in ultra-sensitive detection,10–13 because it can amplify the spectroscopic signatures and offer structural information of target molecules adsorbed on the surface of metal nanostructures.14,15 As is well known, the LSPR of noble metal NCs exhibits a strong dependence on their size, shape and chemical composition.3,16 Naturally, the SERS activity of noble metal NCs is highly reliant upon their geometry, such as intrinsic enhancement of the local surface plasmon “hot spots” which are generated from a huge electric field at large curvatures of surfaces.17 Consequently, it is significant to design and prepare the noble metal NCs with excellent controlled morphology for achieving stronger electric field enhancement and SERS efficiency.18,19
Recently, great efforts have been made to search for the method of fabricating a multiplicity of geometric structures of noble metal NCs,20–22 such as cube,23 triangular,24 prism,25 sphere26 and rod.26 Among them, the isotropic shaped NCs have been systematically studied by many research groups,20 and the extensive studies are moving the focus towards to anisotropic structures.16,27,28 For example, the synthesis of complicated polyhedral NCs,29–35 including platonic solids36 (tetrahedron, cube, octahedron, dodecahedron, and icosahedron) and Archimedean solids26 (cuboctahedron, truncated cuboctahedron, truncated octahedron and truncated tetrahedron).37–44 These solids are categorized in terms of the ancient Greek scientists and very mathematical aesthetics and symmetry in geometry.45 Various synthetic strategies such as polyol reduction,38 plasmon46 and photon driven synthesis47 and seed-assisted growth1 have been used to achieve the geometry-control of polyhedral Au NCs with the aim to deliberately tailor their plasmonic characteristics.48 However, the above synthesis routes need the multi-step and intricate rinsing process, or assisted extra-medium. It is often desirable to realize convenient morphological control of Au NCs with simple synthetic progress in water-soluble environment.49
In this paper, we report a facile hydrothermal method for synthesizing colloidal Au NCs of diverse geometric features using CTAB as a structure-directing agent. By varying the molar ratio between CTAB and Au precursor, the morphology of Au NCs can be adjusted from high degree polygons, cuboctahedron, and octahedron to truncated tetrahedron and bitetrahedron, while their LSPR bands continually change from 561 to 627 nm. And the critical role of CTAB in synthetic process has been investigated in the formation of polyhedral Au NCs. In addition, by means of the finite element method (FEM) calculation and Raman measurement, the plasmonic behaviours of these polyhedral Au NCs such as their geometry-dependence electric field enhancement and SERS activities are analysed. At the incident laser of 785 nm, the polyhedral Au NCs have higher SERS efficiencies since the LSPR band approaching to the irradiate wavelength. Overall, this proposed surfactant-assisted synthesize procedure allows us to precisely fabricate the controllable topography of Au NCs and tailor their plasmonic properties for further SERS-based biological applications.
2. Materials and methods
2.1 Materials
Hydrogen tetrachloroaurate (III) trihydrate (HAuCl4·3H2O) was acquired from Sigma Aldrich. Cetyltrimethylammonium bromide (CTAB) and trisodium citrate (Na3C6H5O7·2H2O) were purchased from Aladdin. 4-Mercaptobenzoic acid (4-MBA) was issued by J&K Chemical. Milli-Q water (18.2 MΩ cm resistivity) was used for all solution preparations. All chemicals were of analytical grade and used as-received. Glassware were cleaned by aqua regia and rinsed with deionized water several times prior to the experiments.
2.2 Synthesis of polyhedral Au NCs
The synthesis of polyhedral gold nanostructures was accomplished via a modified hydrothermal process.50 In our work, the different molar ratios of [CTAB]/[HAuCl4] (CTAB
:
Au = 15–75) were used to synthesize a diverse of polyhedral Au NCs. In a typical synthesis of the Au high degree polygons, 10 ml of 15 mM CTAB, 200 μl of 0.01 M HAuCl4, and 50 μl of 100 mM trisodium citrate aqueous solution were sequentially added and mixed to a 15 ml Teflon-lined stainless-steel autoclave, sealed and then maintained at 110 °C for 12 h after the oven had reached this temperature. Then the autoclave was cooled to room temperature naturally. The resulting Au NCs were harvested by centrifugation at 8000 rpm for 20 min and washed with deionized water for another round of centrifugation to remove excess CTAB. Finally the Au NCs were re-dispersed in deionized water for later characterization. The similar procedures were followed to synthesize other Au NCs with different shapes, except that the CTAB concentration was gradually decreased and lead to different molar ratio of CTAB and HAuCl4 in reaction solution, for example, the truncated cuboctahedron (13.5 mM, CTAB/Au = 67.5), cuboctahedron (12.0 mM, CTAB/Au = 60), octahedron (10.5 mM, CTAB/Au = 52.5), truncated tetrahedron and bitetrahedron (4.5 mM, CTAB/Au = 22.5).
2.3 Synthesis of 4-MBA-labelled Au NCs
The SERS performances of the as-synthesized polyhedral Au NCs were evaluated with 4-MBA Raman molecules. Firstly, the sample solutions were prepared by respectively adding 20 μl of 1 mM 4-MBA solution to the above purified 5 ml of polyhedral Au NCs solutions under stirring, and the resultant solutions were agitated for 5 h. Afterwards, the mixtures were centrifuged at 10
000 rpm for 20 min to remove unbound 4-MBA molecules. Then the sediments at the bottom of centrifuge tubes were re-dispersed in 5 ml deionized water as 4-MBA-labelled polyhedral Au NCs solutions for SERS measurement.
2.4 Instrumentation
UV-vis absorption spectra were monitored with a spectrometer (TU1901, Pgeneral). SU-70 FESEM instrument was used to record the scanning electron microscopy (SEM) images under an accelerating voltage of 5 kV. Transmission electron microscope (TEM), high-resolution transmission electron microscope (HRTEM) images, selective area electron diffraction (SAED) pattern and energy-dispersive X-ray analysis (EDAX) were obtained with TEM (JEM-2100F, JEOL) operated at accelerating voltage of 200 kV. The SERS properties of the samples were examined by a Raman spectrometer (BWS415, B&W Tek Inc.) using a 785 nm semiconductor laser as the excitation source. Raman spectrographs were recorded with a laser power at the sample position of 49.55 mW and an accumulation time of 10 s. The scattered radiation of the colloid solution and the substrate was collected by a 20× objective lens with numerical aperture (NA) of 0.4 and a 40× objective lens with NA of 0.65, respectively. The wavelength dispersion was performed using a 1200 lines per mm grating, and then passed through a slit with 20 μm width to the charge-coupled device (CCD) (2048 × 2048 pixels) detector. All the analysis was carried out at room temperature.
2.5 Calculation
The electromagnetic properties of polyhedral Au NCs were numerically simulated by using the commercial FEM software package (COMSOL Multiphysics 4.4 with the radio frequency module) in an appropriate discrete spatial grid. In our calculations, the frequency dependent dielectric functions for gold are taken from the experimental bulk result of Johnson and Christy.51 The surrounding medium is presumed to be water with the refractive index 1.33. According to the dimensions extracted from the TEM and SEM images in our experiments, three dimension nanostructure models were constructed to simulate the optical absorption spectra and the distributions of electric near-field intensities of the polyhedral Au NCs.
3. Results and discussion
3.1 Characterization of the polyhedral Au NCs
The morphologies of polyhedral Au NCs synthesized at different CTAB concentrations were analyzed via SEM. Fig. 1 presents the SEM images of a diverse of polyhedral Au NCs with uniform in size and shape. It was found that the Au NCs went through a shape evolution by simply diminishing the CTAB concentration from 15 to 4.5 mM. As seen in Fig. 1(a), at a high concentration of CTAB (15.0 mM, CTAB/Au = 75), the high degree polygons (quasi-sphere) are obtained with an average size of 82 nm. And the truncated cuboctahedron with an approximate size of 78 nm is observed in Fig. 1(b) for the concentration of CTAB of 13.5 mM. As decreasing the concentration of CTAB to 12.0 mM, the cuboctahedron as shown in Fig. 1(c) were produced with a mean size of 74 nm. At the concentration of CTAB of 10.5 mM, the octahedral shaped Au NCs were synthesized with an average size of 71 nm. Upon further decreasing the concentration of CTAB from 9 to 6 mM, we discovered that accompanying with the formation octahedron, a significant proportion of truncated tetrahedron and bitetrahedron were generated as byproduct50 and their proportion gradually increased as shown in Fig. S1 (ESI†). Eventually, the truncated tetrahedron and bitetrahedron became the dominated products at a lower CTAB concentration of 4.5 mM, which are shown in Fig. 1(e) and (f) with average particle sizes of 96 and 92 nm, respectively. In a word, the shapes of these polyhedral Au NCs were evolved from high degree polygons at high concentrations of CTAB, cuboctahedron and octahedron at moderate decreasing concentrations of CTAB to a mixture of truncated tetrahedron and bitetrahedron in the presence of low concentration CTAB.
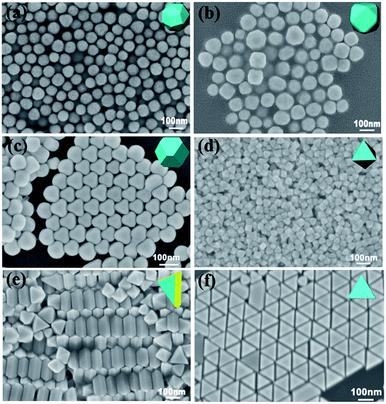 |
| Fig. 1 SEM images of the as-synthesized polyhedral Au NCs. (a) High degree polygons, (b) truncated cuboctahedron, (c) cuboctahedron, (d) octahedron, (e) truncated bitetrahedron and (f) truncated tetrahedron. The inset shows the corresponding drawings of the geometric morphology. | |
Generally, noble metal NCs exhibit very gorgeous color due to the plasmonic property of highly dependent on the crystal size and shape.16 Fig. 2(a) shows the photograph of colloidal polyhedral Au NCs solutions. The color of solutions gradually changed from blue-gray, purplish, to pink red with CTAB concentration increased. The UV-vis absorption spectra of these polyhedral Au NCs are shown in Fig. 2(b). With regard to the absorption spectrum of the high degree polygons Au colloid, it shows a LSPR peak at 561 nm, analogous to that of spherical gold nanoparticles with similar size.23 As decreasing the concentration of CTAB, the LSPR bands of the as-formed Au colloidal solutions display the red-shifts from 571 nm of the truncated cuboctahedron, 574 nm of cuboctahedron to 579 nm of the octahedron. When the CTAB concentration was less than 9 mM, however, the LSPR bands of Au colloidal solutions were further red shifts accompanying with raising of shoulders around 625 nm, which is most likely to arise from co-existing triangular particles shown in Fig. S1 (ESI†).24 Eventually, the shoulder was developed into a new LSPR peak at 632 nm at the case of 4.5 mM CTAB, which could be attributed to the plasmonic role of the truncated tetrahedral and bitetrahedral Au NCs. In addition, the relationship of LSPR peak and the concentration of CTAB is plotted as shown in Fig. 2(c), is clearly demonstrated the shape-dependence LSPR characteristic of Au NCs.
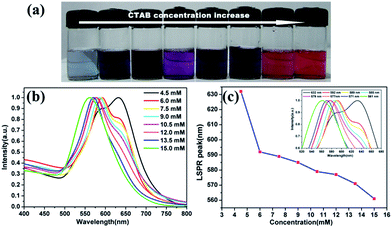 |
| Fig. 2 (a) True color pictures and (b) UV-vis absorption spectra of Au NCs colloidal solutions prepared with different concentration of CTAB, and (c) the positions of LSPR peaks corresponding to the concentrations of CTAB, the inset is partial enlargement of absorption spectra. | |
On the other hand, the crystallography of the as-synthesized polyhedral Au NCs was investigated for providing further insights into the effect of CTAB concentration on the polyhedral structures. Among them, the high degree polygon, the octahedron, the truncated tetrahedron and bitetrahedron were analyzed in details as three typical polyhedral Au structures. Firstly, the high degree polygons are formed under the reacting condition of 15 mM CTAB. The images of SEM and TEM in Fig. 3(a) and (b) exhibited the polygonal structures are consist of more than 10 facets and can also regard as pseudo-spheres with the average diameter of 77 ± 5.0 nm. Fig. 3(c) is the corresponding SAED pattern of individual high degree polygons, which further confirm its single-crystalline structure comprised of two main crystal facets {111} and {100}.36 Secondly, when the concentration of CTAB is 9 mM, octahedral Au NCs with rounded apexes were mass synthesized. Fig. 4(a) and (b) give the SEM and TEM images of a large quantity of octahedral Au NCs with an average edge length 46 ± 3 nm. Based on simulation of FEM, the optical spectra in Fig. 4(c) well display the uniform in size of the octahedral Au NC due to the theoretical calculation is almost in accordance with experimental measure. The HRTEM image of a single octahedral Au NC and its corresponding SAED pattern along the [111] zone axis are shown in Fig. 4(d) and (e) respectively. Because the pattern was recorded by focusing the electron beam on one of the triangle faces of an Au octahedron, the SAED spots with the hexagonal symmetry was indexed to {220} reflections, revealing that octahedral Au NC is a single crystal with the {111} facets as the exposed surfaces.37
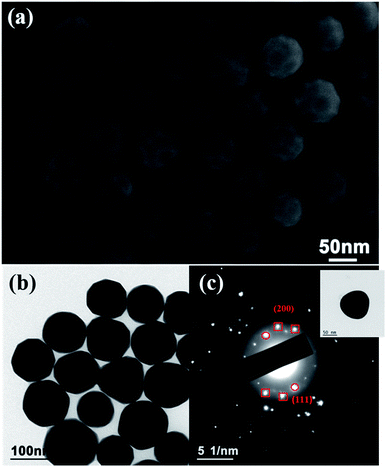 |
| Fig. 3 (a) SEM, (b) TEM images, and (c) corresponding SAED patterns of the high degree polygons. Inset in (c) is HRTEM of an individual high degree polygon. | |
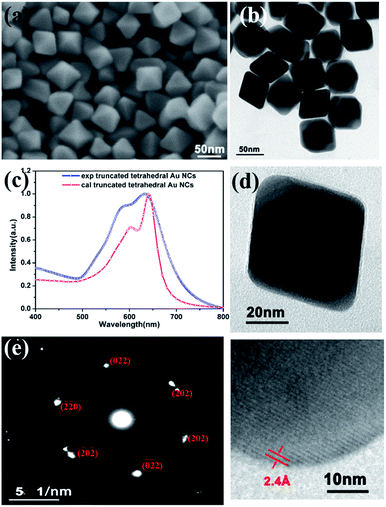 |
| Fig. 4 (a) SEM and (b) TEM images of octahedral Au NCs; (d) HRTEM image, (e) the corresponding SAED pattern along the [111] zone axis and (f) the partial enlarged HRTEM image of a single Au the octahedron; (c) the optical spectra of the octahedrons. | |
And, Fig. 4(f) further indicates a lattice spacing of 2.4 Å indexing to {111} planes of the face-centered cubic (fcc) gold according to the JCPDS no. 87-0720.50 Thirdly, as decreasing of the concentration of CTAB to 4.5 mM, the truncated tetrahedron and bitetrahedron were obtained. As shown in Fig. 5(a) and (c), the as-synthesized Au NCs are triangular structures and seem like triangular prism, however, the clearly slanted sides of the crystals indicate that rather than being flat prisms, these shapes are more accurately described as the tetrahedron with a truncated corner, or the planar twinned analogue of the single crystalline truncated tetrahedron.38 The optical spectra of the triangular-like Au NCs were also analyzed by comparing the experimental data with the corresponding theoretical results. As seen in Fig. 5(b), the positions of LSPR peaks in two spectra are almost the same. It should be noted that, the calculated spectrum shows two narrow LSPR bands, which are different from the experimental results because of only simulating an individual module.37 Fig. 5(d) and (e) are the HRTEM image of a single truncated tetrahedral Au NC and its corresponding SAED pattern, which confirms that the surface of the tetrahedron is single-crystalline structure and comprises of the crystal faces {111} and {100}.
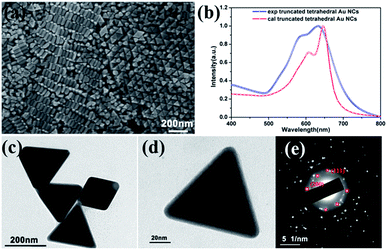 |
| Fig. 5 (a) SEM and (c) TEM images of the truncated tetrahedron and bitetrahedron Au NCs; (d) HRTEM image and (e) the corresponding SAED pattern of a single truncated Au tetrahedron; (b) the optical spectra of the truncated tetrahedron and bitetrahedron. | |
3.2 Growth mechanisms of the polyhedral Au NCs
For the growth procedure of the gold nanostructures, many factors such as materials and growth kinetics are considered to be crucial for reaching the final shapes of the resultant NCs.40,51,52 In our synthesis process of the polyhedral Au NCs, the surface-capping agent, reducing agent, temperature and the oxidative etching are believed to play key roles.2,31,38 First of all, from the analysis in Section 3.1, it is obvious that the concentrations of CTAB greatly influence the shape and surface structure of the Au NCs. As well known, for the fcc gold crystals, its surface energies of the low-index crystallographic facets are given in the order γ{111} < γ{100} < γ{110}, so that the key of shape engineering is able to alter the distribution of exposed crystallographic facets which determine the final shape of nanostructure by promoting selective growth in certain crystallographic directions.21 Among these three low-index crystallographic facets, {111} facets have a close-packed arrangement of atoms and are the most thermodynamically favoured facets.48 In the hydrothermal environment, the higher reaction temperature make the thermodynamically stable structures with low-index facets, such as {111}-favour Au NCs, to be easily formed. Considering of our experiment, these fabricated polyhedral Au NCs are mainly consist of the facets {111} and {100}. Therefore, it is believed that CTAB as surfactant is responsible for altering the energy of Au {100} facet relative to others. A systematic shape evolution scheme of the polyhedral Au NCs is illustrated in Fig. 6 from which the synthetic procedure by modulating CTAB concentration can be visualized. Actually, CTAB molecules were represented as micelles in solution and adsorbed on the {100} facets of gold nuclei to lower the energies of {100} facets and slow the growth of that face for the exposed of the {100} facets.54 With a high molar ratio of [CTAB]/[HAuCl4], there is enough micelles to fully coat of the crystal faces of gold nuclei so that the growth takes place on the facets {111} and {100} roughly at the same rate.53 Consequently, the high degree polygons with the bisection mixture of {111} and {100} facets were obtained. Further decreasing molar ratio of CTAB and gold precursor, results in a sequence decrement of {100} facets, thus the {111} facets became the major favour-facet of the Au NCs ranging from cuboctahedron, octahedron with different degree truncation to truncated tetrahedron and bitetrahedron. Progressively, at extremely low molar concentrations of CTAB such as 3 and 1.5 mM, the surface energy of the {100} facets is not able to be declined and the growth is taken along [100] direction to produce the triangular and hexagonal Au nanoplates with a larger area {111} faces shown in Fig. S2 (ESI†).24
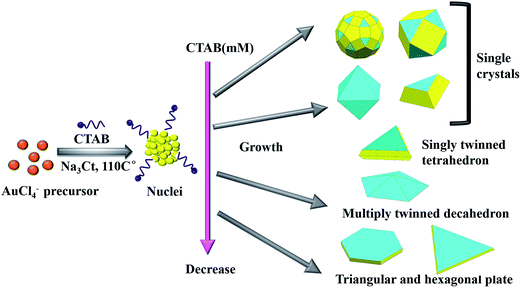 |
| Fig. 6 Schematic of the crystal growth mechanism of polyhedral Au NCs. The blue and yellow colours represent the {111} and {100} facets, respectively. | |
Besides, the roles of trisodium citrate and oxygen should not be neglected in the process of crystal shape evolution. At our preparing conditions, trisodium citrate is not only used to reduce the gold precursor into Au atoms which further cluster and form into small Au nuclei,24,50 but also to promote the formation of {111} facets of nanocrystals and in favour of the growth of nanoplates. Furthermore, as the oxidative etching agent, existing of O2 over the reaction solution could be expected to result in dissolution of Au and completion with the reduction of Au ions, leading to the slow nucleation and the growth of crystals.35,42,51 And the etching effect also caused dissolving of the higher active multiply and twinned crystals while remaining single-crystal particles.1,2,43,52 After all, by controlling the rate of gold atom added on the crystal surface through synergistic effect of the above discussed factors, the relative growth rates along [100] and [111] directions can be tuned to form particular shapes Au NCs.3,27,29
3.3 SERS performances of the polyhedral Au NCs
Generally, SERS phenomenon is usually described by two main enhancement mechanisms, the electromagnetic and the chemical enhancement where the former plays the dominating role.8 As a suitable method, FEM is usually used to numerically analyse the electromagnetic problems of complex metallic nanostructures on an appropriate discretized spatial grid. To pre-estimate the SERS behaviour of the as-prepared polyhedral Au NCs, models of the truncated Au octahedral and tetrahedral nanostructures were constructed and their near-field distributions were simulated by FEM. Firstly, for the incident plane-wave polarized along x axis at 785 nm wavelength, the enhanced near-field distribution of an individual truncated Au octahedron with the edge length of 45 nm has been calculated as shown in Fig. 7(a)–(c) under the cross-sectional views along x–y, x–z and y–z planes, respectively. As anticipated, the enhanced near-field distribution of octahedron is not uniform across the entire surface of nanostructure, but rather the areas of the most enhanced electric field are located in the vicinities around the tips and edges of the gold octahedron.9 On the other hand, as shown in Fig. 8, the enhanced near-field distribution of an individual truncated Au tetrahedron with an edge length of 90 nm was analogous to that of the octahedral Au NCs, i.e. the truncated Au tetrahedron generates stronger polarized light-induced electric field located at the edges and corners as well. In addition, the planar twinned analogue of the truncated tetrahedron, a truncated Au bitetrahedron model has also been simulated and the enhanced near-field distributing has shown in Fig. S3 (ESI†). And the high degree polygons can be regard as quasi-spheres, so the enhanced near-field distribution may resemble to that of Au nanospheres with the same dimension was shown in Fig. S4 (ESI†). While comparing with the maximum values of enhanced electric field intensity of the high degree polygons and truncated Au octahedron, the truncated Au tetrahedron and bitetrahedron exhibit stronger electric field enhancements under the excitation light of 785 nm. Next, the SERS performance of the as-prepared polyhedral Au NCs was valued by measuring the Raman spectrum of active molecular 4-MBA with 785 nm semiconductor laser as the excitation source. As shown in Fig. 9, the characteristic fingerprint bands of pure 4-MBA are located at ∼1078 and 1590 cm−1 which are ascribed to the ν(C–C) benzene ring-breathing modes.55 Especially, for the truncated tetrahedral and bitetrahedral Au NCs synthesized at 4.5 mM CTAB concentration, its SERS signals are the strongest among the 4-MBA labeled polyhedral Au NCs. This is presumably related to the electric near-field enhancement affected by the plasmon resonance behavior of Au NCs. With the formation of triangular analogue structures at a lower concentration of CTAB, the SERS intensity increases as a better resonance coupling between the LSPR peak around 632 nm and the incident laser at 785 nm.56 The above experimental results are consistent with the enhanced electric near-field intensity calculated by FEM. To see the SERS activities of as-prepared polyhedral Au NCs quantitatively, the enhancement factor (EF) is further estimated by comparing SERS signals with normal Raman intensities obtained from a pure 4-MBA solution. Since the benzene ring C–C stretching mode showed larger intensities, most likely due to the stronger coupling between the transition dipole moment and the local electric field, the intensity of SERS peak at 1078 cm−1 was utilized to calculate the EF value. The calculations are following the equation EF = (ISERS/Ibulk) × (Nbulk/NSERS), where ISERS and Ibulk are the integrated intensities of a characteristic band from SERS and from bulk Raman spectra, respectively; Nbulk is the number of bulk molecules probed in the bulk sample; and NSERS is the number of molecules adsorbed on Au NCs. The Raman spectrum of the 4-MBA solution (10 mM) was used to assess the “bulk” values of the EF. The same calculation method has been stated in our previous work,57 and the detailed numerical calculation process were shown in ESI.† Thus, corresponding to the SERS spectra in Fig. 9, the EF values of the polyhedral Au NCs colloid samples were given as EF1 = 7.51 × 105, EF2 = 9.15 × 105, EF3 = 1.01 × 106, EF4 = 1.10 × 106, EF5 = 1.29 × 106. It reveals all of the polyhedral Au NCs display well SERS activities and the coupling between LSPR and the excitation laser plays an important role in determining SERS enhancement. Therefore, it should be expected that Au polyhedral NCs can serve as superior SERS probes with favorable excitation laser.
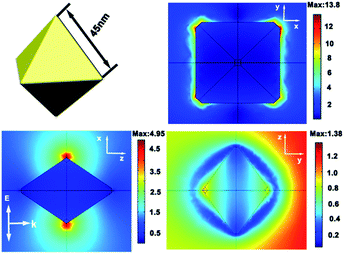 |
| Fig. 7 (a) 3D model of the truncated Au octahedron and the corresponding distributions of near-field enhancement in the tangent planes along (a) x–y plane, (b) x–z plane and (c) y–z plane. The coordinate axes and the propagation direction (k vector) and the polarization (E field vector) are shown in white arrows. | |
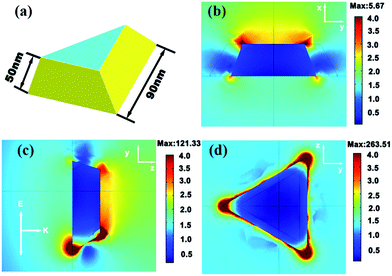 |
| Fig. 8 (a) 3D model of the truncated Au tetrahedron and the corresponding distributions of near-field enhancement in the tangent planes along (a) x–y plane, (b) x–z plane and (c) y–z plane. | |
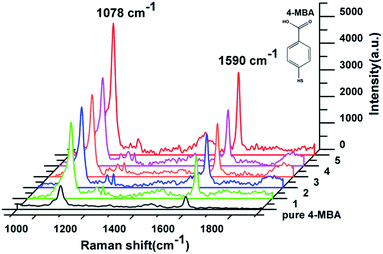 |
| Fig. 9 Raman spectra of 4-MBA labelled polyhedral Au NCs. Labels 1–5 in the y axis correspond to high degree Au polygon, Au truncated cuboctahedron, Au cuboctahedron, Au truncated octahedron and Au truncated bitetrahedron and tetrahedron, respectively. | |
4. Conclusions
In summary, six classes of well-defined shapes of polyhedral Au NCs have successfully been fabricated through a facile hydrothermal strategy via gradually changing the concentration of CTAB, and the shape-dependent optical properties of these polyhedral Au NCs have systematically been investigated. We have also analyzed elaborately on the formatting mechanism of Au NCs and disclosed that the growth system is {111}-favor Au NCs process. Then the LSPR and the SERS properties were simulated and measured by FEM and 4-MBA molecules adsorbed on the colloidal polyhedral Au NCs, respectively. The results show that SERS enhancement factors have been achieved on the order of 105 to 106, and the stronger SERS enhancement was observed under near-infrared (785 nm) excitation because the laser wavelength approaches the plasmon coupling band of the Au NCs. Therefore, the controllable synthesis of polyhedral Au NCs provides an effective strategy to architecturally design geometry-dependent noble metal materials and tailor their enhancement feature. Furthermore, Au NCs with effective SERS enhancement may lead to potential application such as SERS-based spectroscopy detection of a wide variety of biological molecules.
Acknowledgements
This work was supported by the National Natural Science Foundation of China (Grant No. 61138003, 61275153, 61320106014 and 11404177); Ministry of Science and Technology of China under National Basic Research Program of China (973) Grant No. 2015CB352004; the Natural Science Foundation of Zhejiang (Grant No. LY12A04002) and K. C. Wong Magna Foundation of Ningbo University, China.
Notes and references
- L. Polavarapu, S. Mourdikoudis, I. Pastoriza-Santos and J. Perez-Juste, CrystEngComm, 2015, 17, 3727–3762 RSC.
- Y. Xia, Y. Xiong, B. Lim and S. E. Skrabalak, Angew. Chem., Int. Ed., 2009, 48, 60–103 CrossRef CAS PubMed.
- C. J. Murphy, T. K. Sau, A. M. Gole, C. J. Orendorff, J. Gao, L. Gou, S. E. Hunyadi and T. Li, J. Phys. Chem. B, 2005, 109, 13857–13870 CrossRef CAS PubMed.
- M. Rycenga, C. M. Cobley, J. Zeng, W. Li, C. H. Moran, Q. Zhang, D. Qin and Y. Xia, Chem. Rev., 2011, 111, 3669–3712 CrossRef CAS PubMed.
- S. Liu and Z. Tang, J. Mater. Chem., 2010, 20, 24–35 RSC.
- G. Konstantatos and E. H. Sargent, Nat. Nanotechnol., 2010, 5, 391–400 CrossRef CAS PubMed.
- M. Fernanda Cardinal, B. Rodríguez-González, R. n. A. Alvarez-Puebla, J. Pérez-Juste and L. M. Liz-Marzán, J. Phys. Chem. C, 2010, 114, 10417–10423 CAS.
- J. B. Jackson and N. J. Halas, Proc. Natl. Acad. Sci. U. S. A., 2004, 101, 17930–17935 CrossRef CAS PubMed.
- D. Lee and S. Yoon, J. Phys. Chem. C, 2015, 119, 7873–7882 CAS.
- G. Zheng, J. Wang, L. Kong, H. Cheng and J. Liu, Plasmonics, 2012, 7, 487–494 CrossRef CAS.
- Q. Tao, S. Li, C. Ma, K. Liu and Q.-Y. Zhang, Dalton Trans., 2015, 44, 3447–3453 RSC.
- T. Jiang, L. Zhang, H. Jin, X. Wang and J. Zhou, Dalton Trans., 2015, 44, 7606–7612 RSC.
- C. Farcau, M. Potara, C. Leordean, S. Boca and S. Astilean, Analyst, 2013, 138, 546–552 RSC.
- S. Nie and S. R. Emory, Science, 1997, 275, 1102–1106 CrossRef CAS PubMed.
- J. A. Dieringer, K. L. Wustholz, D. J. Masiello, J. P. Camden, S. L. Kleinman, G. C. Schatz and R. P. van Duyne, J. Am. Chem. Soc., 2009, 131, 849–854 CrossRef CAS PubMed.
- E. Hao, G. Schatz and J. Hupp, J. Fluoresc., 2004, 14, 331–341 CrossRef CAS.
- J. M. McLellan, A. Siekkinen, J. Chen and Y. Xia, Chem. Phys. Lett., 2006, 427, 122–126 CrossRef CAS PubMed.
- A. K. Samal, L. Polavarapu, S. Rodal-Cedeira, L. M. Liz-Marzán, J. Pérez-Juste and I. Pastoriza-Santos, Langmuir, 2013, 29, 15076–15082 CrossRef CAS PubMed.
- H.-L. Wu, H.-R. Tsai, Y.-T. Hung, K.-U. Lao, C.-W. Liao, P.-J. Chung, J.-S. Huang, I. C. Chen and M. H. Huang, Inorg. Chem., 2011, 50, 8106–8111 CrossRef CAS PubMed.
- C. N. R. Rao, H. S. S. Ramakrishna Matte, R. Voggu and A. Govindaraj, Dalton Trans., 2012, 41, 5089–5120 RSC.
- L. Manna, E. Scher and A. P. Alivisatos, J. Cluster Sci., 2002, 13, 521–532 CrossRef CAS.
- Y. Liu, J. Goebl and Y. Yin, Chem. Soc. Rev., 2013, 42, 2610–2653 RSC.
- H. Wang, C. S. Levin and N. J. Halas, J. Am. Chem. Soc., 2005, 127, 14992–14993 CrossRef CAS PubMed.
- H.-C. Chu, C.-H. Kuo and M. H. Huang, Inorg. Chem., 2006, 45, 808–813 CrossRef CAS PubMed.
- C. Xue, J. E. Millstone, S. Li and C. A. Mirkin, Angew. Chem., Int. Ed., 2007, 46, 8436–8439 CrossRef CAS PubMed.
- S. Zhang, X. Kou, Z. Yang, Q. Shi, G. D. Stucky, L. Sun, J. Wang and C. Yan, Chem. Commun., 2007, 18, 1816–1818 RSC.
- S. E. Lohse, N. D. Burrows, L. Scarabelli, L. M. Liz-Marzán and C. J. Murphy, Chem. Mater., 2013, 26, 34–43 CrossRef.
- T. K. Sau and A. L. Rogach, Adv. Mater., 2010, 22, 1781–1804 CrossRef CAS PubMed.
- D. Seo, J. C. Park and H. Song, J. Am. Chem. Soc., 2006, 128, 14863–14870 CrossRef CAS PubMed.
- J. H. Wu, Z. Guan, S. K. Yang, P. Yuan, Q.-H. Xu and G. Q. Xu, Nanoscale, 2013, 5, 2983–2989 RSC.
- T. T. Tran and X. Lu, J. Phys. Chem. C, 2011, 115, 3638–3645 CAS.
- J. Xiao, S. Liu, N. Tian, Z.-Y. Zhou, H.-X. Liu, B.-B. Xu and S.-G. Sun, J. Am. Chem. Soc., 2013, 135, 18754–18757 CrossRef CAS PubMed.
- Y. Yu, Q. Zhang, X. Lu and J. Y. Lee, J. Phys. Chem. C, 2010, 114, 11119–11126 CAS.
- L. Zhang, W. Niu and G. Xu, Nano Today, 2012, 7, 586–605 CrossRef CAS PubMed.
- Q. Fu, Y. Sheng, H. Tang, Z. Zhu, M. Ruan, W. Xu, Y. Zhu and Z. Tang, ACS Nano, 2015, 9, 172–179 CrossRef CAS PubMed.
- F. Kim, S. Connor, H. Song, T. Kuykendall and P. Yang, Angew. Chem., 2004, 116, 3759–3763 CrossRef PubMed.
- C. Li, K. L. Shuford, Q. H. Park, W. Cai, Y. Li, E. J. Lee and S. O. Cho, Angew. Chem., 2007, 119, 3328–3332 CrossRef PubMed.
- B. Wiley, T. Herricks, Y. Sun and Y. Xia, Nano Lett., 2004, 4, 1733–1739 CrossRef CAS.
- H.-L. Wu, C.-H. Kuo and M. H. Huang, Langmuir, 2010, 26, 12307–12313 CrossRef CAS PubMed.
- M. R. Langille, M. L. Personick, J. Zhang and C. A. Mirkin, J. Am. Chem. Soc., 2011, 133, 10414–10417 CrossRef CAS PubMed.
- C. Cao, S. Park and S. J. Sim, J. Colloid Interface Sci., 2008, 322, 152–157 CrossRef CAS PubMed.
- W. Niu, S. Zheng, D. Wang, X. Liu, H. Li, S. Han, J. Chen, Z. Tang and G. Xu, J. Am. Chem. Soc., 2009, 131, 697–703 CrossRef CAS PubMed.
- M. Kim, H. J. Park, S. W. Han, J. Park and W. S. Yun, Bull. Korean Chem. Soc., 2013, 34, 2243–2244 CrossRef CAS.
- P. Zhao, N. Li and D. Astruc, Coord. Chem. Rev., 2013, 257, 638–665 CrossRef CAS PubMed.
- P. F. Damasceno, M. Engel and S. C. Glotzer, Science, 2012, 337, 453–457 CrossRef CAS PubMed.
- C. Xue, G. S. Métraux, J. E. Millstone and C. A. Mirkin, J. Am. Chem. Soc., 2008, 130, 8337–8344 CrossRef CAS PubMed.
- M. R. Langille, M. L. Personick and C. A. Mirkin, Angew. Chem., Int. Ed., 2013, 52, 13910–13940 CrossRef CAS PubMed.
- R. Contreras-Cáceres, A. Sánchez-Iglesias, M. Karg, I. Pastoriza-Santos, J. Pérez-Juste, J. Pacifico, T. Hellweg, A. Fernández-Barbero and L. M. Liz-Marzán, Adv. Mater., 2008, 20, 1666–1670 CrossRef PubMed.
- T. Jiang, B. Wang, L. Zhang and J. Zhou, J. Alloys Compd., 2015, 632, 140–146 CrossRef CAS PubMed.
- C.-C. Chang, H.-L. Wu, C.-H. Kuo and M. H. Huang, Chem. Mater., 2008, 20, 7570–7574 CrossRef CAS.
- P. B. Johnson and R. W. Christy, Phys. Rev. B: Condens. Matter Mater. Phys., 1972, 6, 4370–4379 CrossRef CAS.
- M. R. Langille, M. L. Personick, J. Zhang and C. A. Mirkin, J. Am. Chem. Soc., 2012, 134, 14542–14554 CrossRef CAS PubMed.
- M. L. Personick and C. A. Mirkin, J. Am. Chem. Soc., 2013, 135, 18238–18247 CrossRef CAS PubMed.
- C. Bullen, P. Zijlstra, E. Bakker, M. Gu and C. Raston, Cryst. Growth Des., 2011, 11, 3375–3380 CAS.
- A. Michota and J. Bukowska, J. Raman Spectrosc., 2003, 34, 21–25 CrossRef CAS PubMed.
- Y. Hu, T. Chou, H. Wang and H. Du, J. Phys. Chem. C, 2014, 118, 16011–16018 CAS.
- Y. Liu, J. Zhou, B. Wang, T. Jiang, H.-P. Ho, L. Petti and P. Mormile, Phys. Chem. Chem. Phys., 2015, 17, 6819–6826 RSC.
Footnote |
† Electronic supplementary information (ESI) available. See DOI: 10.1039/c5ra10781d |
|
This journal is © The Royal Society of Chemistry 2015 |