DOI:
10.1039/C5RA10603F
(Paper)
RSC Adv., 2015,
5, 61051-61059
PEG–PLGA–PLL nanoparticles in combination with gambogic acid for reversing multidrug resistance of K562/A02 cells to daunorubicin
Received
19th June 2015
, Accepted 9th July 2015
First published on 10th July 2015
Abstract
The present chemotherapy in malignancy treatment, including leukaemia, is plagued by one main problem: multidrug resistance (MDR) which is often related to excessive expression of adenosine triphosphate-dependent efflux pump. In recent years, attempts have been focused on searching competitive inhibitors. However, high toxicity and severe side effects were the main impediments for the application of inhibitors. Therefore, this study investigated a new strategy to utilise polyethylene glycol–polylactic-co-glycolic acid–poly-L-lysine (PEG–PLGA–PLL) as a delivery system to combine with gambogic acid (GA) with the purpose of delivering daunorubicin (DNR) to the target location to reserve MDR with maximised therapeutic efficacy and minimised side effects. The anti-tumour ability of DNR in the presence of GA–PEG–PLGA–PLL was evaluated by methyl thiazolyl tetrazolium (MTT) assay, flow cytometry and 4′,6-diamidino-2-phenylindole (DAPI) staining. The possible signalling pathway was demonstrated by quantitative real-time polymerase chain reaction (QPCR) and Western blot assay. The results showed that the intracellular concentration of DNR can evidently increase with the presence of GA–PEG–PLGA–PLL, and the resistance of K562/A02 cells to DNR can be reversed when combined with GA–PEG–PLGA–PLL, which acted as a delivery system. Thus, anti-tumour efficiency was promoted. Overall, GA–PEG–PLGA–PLL can be a promising agent in reversing MDR of K562/A02 to DNR.
1. Introduction
Myeloproliferative disease leukaemia is characterised by hyper-proliferation of stem cells, which are subsequently differentiated into peripheral white blood cells. In 2010, approximately 281
500 people died of leukaemia globally,1 and this disease became the 11th most common cause of cancer-related death.2 Bone marrow transplant is an effective therapy, but the search for appropriately matched bone marrow donors is an arduous process, and the therapy is an expensive procedure. Thus, chemotherapy remains one of the most useful approaches to treat leukaemia, although this treatment presents significant drawbacks such as systemic side effects, including renal toxicity, bone marrow suppression and gastrointestinal reaction. Moreover, prolonged chemotherapy can result in multidrug resistance (MDR), which is characterised by cross-resistance to a wide variety of anticancer drugs with unrelated structures and functions.3 Thus, MDR is one of the major obstacles for effective chemotherapy.
Daunorubicin (DNR) is an effective chemotherapeutic agent which is widely used to treat leukaemia. However, DNR lacks specificity to cancer cells and can induce severe side effects. The development of MDR to DNR also hampered the clinical application of DNR. Therefore, new strategies are needed to overcome drug resistance and selectively deliver chemotherapeutic drugs to the tumour area with the aim of improving therapeutic efficacy.
The natural product gambogic acid (GA, C38H44O8) is the active ingredient of gamboge, the resin purified from Garcinia hanburyi.4,5 GA has demonstrated diverse effects such as anti-oxidant, anti-inflammatory and anti-infection properties.6 GA has also been used as a promising anticancer agent to inhibit the growth of various cancer cells;7–9 studies have shown that GA can enhance the apoptosis of MG-63 and K562/A02 cells when combined with cisplatin (CDDP) and DNR, respectively, indicating the synergistic effect of GA with CDDP and DNR.10,11 Substantial research has stated that GA can resynthesise cancer cells which have required resistance to anticancer drugs, such as GC-823/Doc cell lines of gastric cancer to docetaxel12 and K562/A02 cell lines of leukaemia to adriamycin.13 However, the clinical application of GA has been impeded because of poor aqueous solubility, severe side effects and toxicity in normal cells and tissues. Thus, GA should be modified with particular materials.
Recently, many strategies have been utilised to enhance the lethal effects of chemotherapeutic agents to tumour cells which can target specific location. Among the various approaches, nanocarriers have received much attention.14 As one of the several types of nanocarriers, nanoparticle (NP) delivery systems provide many advantages, such as small size, less toxicity, increased drug efficacy and enhanced drug solubility and stability because of the unique properties of these systems.15 In this study, we used polyethylene glycol (PEG), polylactic-co-glycolic acid (PLGA) and poly-L-lysine (PLL) to prepare PEG–PLGA–PLL as the drug delivery system and combine with GA to synthesise GA–PEG–PLGA–PLL. The abilities of GA–PEG–PLGA–PLL to enhance the therapeutic efficacy of DNR and resynthesise the K562/A02 cells to DNR were subsequently evaluated. To gain further understanding of the mechanism, the possible signalling pathway was also investigated.
We initially proposed PEG–PLGA–PLL as a new drug delivery system in combination with GA to overcome drug resistance of the DNR-resistant cancer cell line K562/A02 by resynthesising the cells to DNR, as well as decrease the systemic side effects by enhancing the intracellular concentration.
2. Materials and methods
2.1 Materials
All reagents used in this study were of analytical grade and required no further purification. PEG–PLGA–PLL was provided by Nanjing University. GA (Kanion Pharmaceutical Co., Ltd., Jiangsu, China) was dissolved in dimethyl sulfoxide (DMSO; Sigma-Aldrich, St Louis, MO, USA), stored at −20 °C and then diluted as needed in Roswell Park Memorial Institute (RPMI) 1640 medium (Thermo Fisher Scientific, Waltham, MA, USA). The cytotoxicity assay kit and Annexin V-Fluorescein isothiocyanate (FITC) apoptosis detection kit were obtained from Beyotime Biotechnology Co., Ltd. (Nantong, China).
2.2 Cells and cell culture
Leukaemia MDR cell line K562/A02 was cultured in a complete medium (RPMI 1640 supplemented with 10% foetal bovine serum, 2 mM L-glutamine, 100 mU ml−1 penicillin and 100 μg ml−1 streptomycin) at 37 °C in a humidified atmosphere of 5% CO2. Every after 3 days, 1 μg ml−1 DNR was added into the medium to maintain the MDR phenotype. The cells were passaged every 2 days to 3 days. Prior to the use of cells in the experiments, the cells were cultured in drug-free medium for 10 days. The cell survival rate was >98%.
2.3 Synthesis of PEG–PLGA–PLL and preparation of GA–PEG–PLGA–PLL
PEG–PLGA–PLL was prepared by Nanjing University as the previous paper.16 The GA–PLGA–PLL–PEG was prepared using a modified double-emulsion (water-in-oil-in-water) solvent evaporation/diffusion method. Briefly, 100 mg of PLGA–PLL–PEG was dissolved in 10 ml organic mixture of DCM and ACE with various ratios (from 5
:
0 to 2
:
1, v/v) containing Tween-80 (5%, v/v) as an emulsifier. GA was dissolved in 2 ml of distilled water, and then emulsified in the polymer solution through homogenization for 1 min × 2. The primary W/O emulsion was further added to 40 ml of external water with homogenization (3 min) to achieve the stable double emulsion (W/O/W). The resulting emulsion was dropped gradually into 200 ml of the aqueous solution with different surfactants (Pluronic F68, PVA, and gelatin) under steady stirring to make the nanoparticles solidify. The residual organic solvents were evaporated under negative pressure and the nanoparticles suspending in emulsion were collected by ultracentrifugation at 15
000 rpm and washed with distilled water three times. Finally, the products were dried by lyophilization and stored at 4 °C.
2.4 Characterization of PEG–PLGA–PLL and GA–PEG–PLGA–PLL and drug release
To characterize the microstructure of GA–PEG–PLGA–PLL nanocomposites, the transmission electron microscope (TEM) images were obtained using a high resolution transmission electron microscope (HRTEM, Hitachi Ltd, Tokyo, Japan). The mean particle size and zeta potential of the PEG–PLGA–PLL and GA–PEG–PLGA–PLL at 22 °C were determined by using a Zetasizer 3000HS system (Malvern Instruments, Malvern, UK). Meanwhile, drug loading efficiency and drug entrapping efficiency were calculated. Drug loading efficiency was defined as the ratio of the amount of GA encapsulated in the PEG–PLGA–PLL to the total amount of PEG–PLGA–PLL, and drug entrapping efficiency mean the actual amount of GA encapsulated into PEG–PLGA–PLL vs. the theoretical of GA in PEG–PLGA–PLL.
The releasing capacity of GA from GA–PEG–PLGA–PLL was investigated at pH 6.0 (pH of the environment around the tumor), and pH 7.4 (pH of physiological blood). In brief, the GA–PEG–PLGA–PLL were dispersed in PBS (pH 7.4, 5 ml) and transferred into the dialysis bag. The dialysis bag was immersed in 95 ml PBS of pH 6.0 and 7.4, respectively. Then, the release medium was continuously agitated with stirring speed 100 rpm at 37 °C. 2 mL Two milliliters of the external medium was collected and replaced with the same fresh PBS at predetermined time intervals. The amount of released GA in the medium was analysed by HPLC.
2.5 In vitro cytotoxicity studies
Standard methyl thiazolyl tetrazolium (MTT) assay was used to evaluate the cell viability. Briefly, the exponentially growing K562/A02 cells were seeded into a 96-well plate (1 × 104 cells per well) and treated with different groups. After incubation at 37 °C in a humidified atmosphere with 5% CO2 for 24, 36 and 48 h, MTT solution (20 μl, 5 mg ml−1) was added to each well, and the cells were incubated for additional 4 h. The media were then removed after centrifugation at 800 rpm for 5 min. DMSO was added with a density of 150 μl per well, and the system was shaken for 2 min. Cytotoxic activity was measured by reading the optical density (OD) on a microplate reader at 540 nm wavelength. Cell viability (%) was calculated as the OD of test group/OD of control group × 100%. Each sample was analysed in triplicate.
2.6 Cellular uptake
To demonstrate whether GA and GA–PEG–PLGA–PLL can increase the intracellular concentration of DNR, the density of DNR in K562/A02 cells was measured using the autofluorescence properties of the drug by flow cytometry (FCM). K562/A02 cells in different groups (control, DNR, DNR + GA and GA–PEG–PLGA–PLL + DNR) were cultured for 48 h in a complete medium. Afterwards, the responding cells were collected, washed and suspended with 200 μl of PBS. FCM was used to quantitatively measure the cellular uptake. The cells without treatment acted as the control group. The results were then analysed with CellQuest software.
2.7 Apoptosis of K562/A02 cells
Cells in different groups were labelled with FITC-conjugated Annexin V and propidium iodide by using the Annexin V-FITC apoptosis detection kit in accordance with the manufacturer's instructions.
2.8 Morphological changes of K562/A02 cells
Fluorescence microscopy was used to study the morphological changes of K562/A02 cells. Briefly, the cells treated with four different groups were collected after incubation for 48 h and stained with 4′,6-diamidino-2-phenylindole (DAPI) following the manufacturer's instructions. The morphological changes were observed under a fluorescence microscope.
2.9 Quantitative real-time polymerase chain reaction (QPCR) and Western blot assay
QPCR was performed to evaluate the mRNA levels of P-gp and survivin, and the regulatory protein (P-gp, survivin) expression levels were determined by Western blot assay. The experimental procedures were conducted using conventional methods.
2.10 Statistical analysis
Data were expressed as mean ± standard deviation. Statistical analyses were performed with a parametric test (Student's t-test) by using SPSS software. A value of P < 0.05 was considered significant.
3. Results
3.1 Characterization of GA–PEG–PLGA–PLL and in vitro drug release behavior
The size distribution, zeta potential and morphology were shown in Fig. 1. The chemical structure of PEG–PLGA–PLL was showed in the Fig. 1A. The mean size and zeta potential of PEG–PLGA–PLL were 213 nm and −2.74 ± 0.28 mV, respectively (Fig. 1B). The size distribution of GA–PEG–PLGA–PLL was 219 nm, which was almost the same with PEG–PLGA–PLL, and the zeta potential was −19.16 ± 0.31 mV (Fig. 1C). Fig. 1D showed that GA–PEG–PLGA–PLL owned a spherical shape and was well-dispersed. The EE and LE of NPs-GA nanocomposites were assessed and calculated as 86.5 ± 0.7% and 4.27 ± 0.12%, respectively, showing a promising option of PEG–PLGA–PLL-loaded GA to act as an anticancer drug delivery carrier. All these results demonstrated that GA has very little effects on the size of PEG–PLGA–PLL and GA–PEG–PLGA–PLL could be stored stably.
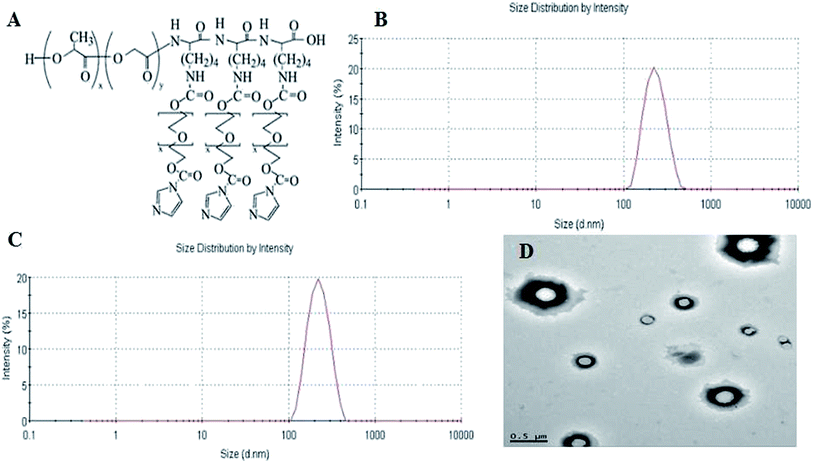 |
| Fig. 1 (A) The chemical structure of PEG–PLGA–PLL. (B) Particle size distribution of PEG–PLGA–PLL. (C) Particle size distribution of GA–PEG–PLGA–PLL. (D) Physical image of GA–PEG–PLGA–PLL under HRTEM. Abbreviations: PEG–PLGA–PLL, polyethylene glycol–polylactic-co-glycolic acid–poly-L-lysine; GA, gambogic acid; HRTEM, high resolution transmission electron microscope. | |
It could be seen from Fig. 2 that the release of GA molecules was dependent on the pH of the medium, as well as the releasing time. It was obviously that GA release was fast at pH 6.0 (i.e., surrounding the tumor sites) with approximately 83.2% of the drug released within 24 h, while it was much more sustained and slow at pH 7.4 (normal physiological conditions). The results demonstrate a pH-triggered release behavior, which can reduce the side effects greatly.
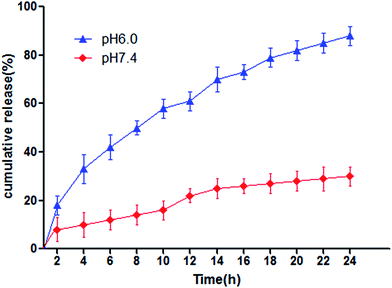 |
| Fig. 2 In vitro GA release behaviors at pH 6.0 and 7.4 respectively. Abbreviations: GA, gambogic acid. | |
3.2 In vitro cytotoxicity studies
Prior to in vivo application, the cytotoxicity of PEG–PLGA–PLL was the first-level evaluation, and MTT assay was employed to estimate the cytotoxic effect. Upon treatment with various concentrations of PEG–PLGA–PLL from 0 μg ml−1 to 100 μg ml−1 for 24, 36 and 48 h, >90% of K562/A02 cells and HELF (human embryonic lung fibroblast) survived, indicating that the blank PEG–PLGA–PLL lack cytotoxicity and are highly compatible with live cells (Fig. 3).
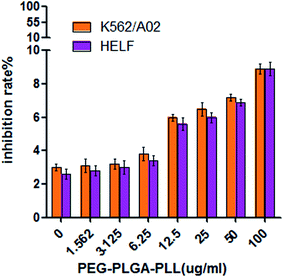 |
| Fig. 3 The cytotoxicity of PEG–PLGA–PLL for K562/A02 and HELF cells in vitro for 48 h (P > 0.05). Abbreviations: PEG–PLGA–PLL, polyethylene glycol–polylactic-co-glycolic acid–poly-L-lysine; HELF, human embryonic lung fibroblast. | |
Subsequently, the in vitro growth inhibitory effect of GA on the K562/A02 cells was evaluated. The results (Fig. 4) showed that GA increased the cytotoxicity in a dose- and time-dependent manner, and the treatment of cells with 0.01562, 0.03125 and 0.0625 μM showed no significant effect on cell viability with the growth inhibition of cells under 10% after inhibition for 24, 36 and 48 h. In this study, GA was acted as a reversal agent, so in order to avoid the inhibitory effect of GA on K562/A02 cells we used 0.0625 μM for further study.
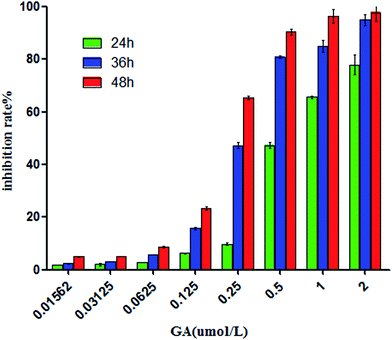 |
| Fig. 4 The cytotoxicity of GA for K562/A02 cells at 24 h, 36 h and 48 h. (P < 0.05). Abbreviations: GA, gambogic acid. | |
We then evaluated the cell viability of K562/A02 cells treated with DNR. With increasing time of incubation and concentration of DNR, the lethality increased, suggesting a time- and dose-dependent effect of DNR in vitro (Fig. 5). Inhibiting concentration of 50% (IC50) after 48 h treatment was calculated as 29.52 ± 1.47 μg ml−1. Afterwards, we investigated whether the addition of GA can increase DNR sensitivity. The results showed that when K562/A02 cells were treated with DNR at different concentrations with the presence of 0.0625 μmol L−1 GA, the viability of cells decreased compared with DNR treatment alone, and the IC50 value of DNR at 48 h was 17.76 ± 1.25 μg ml−1, which indicated the ability of GA to reduce the IC50 of DNR in K562/A02 cells (Fig. 5). We also calculated the drug resistance reserve index (RI) of GA as 1.66 by the following equation: IC50 of therapeutic drug/IC50 of therapeutic drug with reversal agent. These results demonstrated that GA can enhance the cytotoxicity of DNR, which will aid in improving the anti-cancer therapeutic effect of DNR and can desensitise the MDR of K562/A02 cells to DNR simultaneously.
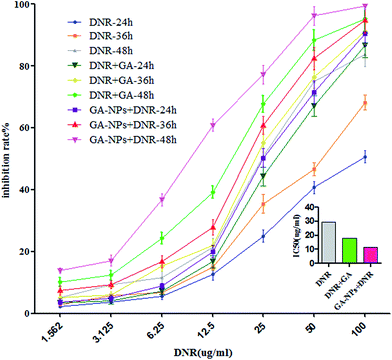 |
| Fig. 5 The cytotoxicity of DNR,DNR + GA and GA–PEG–PLGA–PLL + DNR for K562/A02 cells (P < 0.05). Inset: the IC50 of DNR, DNR + GA and GA–PEG–PLGA–PLL + DNR for K562/A02 cells after incubation for 48 h (P < 0.05). Abbreviations: DNR, daunorubicin; GA, gambogic acid; NPs, polyethylene glycol–polylactic-co-glycolic acid–poly-L-lysine (PEG–PLGA–PLL). | |
To evaluate the effect of GA–PEG–PLGA–PLL to the viability of K562/A02 cells, we combined the GA–PEG–PLGA–PLL with various densities of DNR from 1.562 μg ml−1 to 100 μg ml−1 to treat K562/A02 cells. With the presence of GA–PEG–PLGA–PLL, the mortality of K562/A02 cells increased remarkably, and the IC50 of DNR at 48 h was 11.57 ± 1.06 μg ml−1, which was significantly lower than that with DNR treatment alone (Fig. 5). Moreover, the RI of GA–PEG–PLGA–PLL + DNR was calculated as 54% higher than that of GA with DNR, which increased to 2.55. These data suggested that PEG–PLGA–PLL can effectively enhance the cytotoxicity of DNR in growth inhibition of K562/A02 cells and synergistically affect the GA in desensitisation of MDR cells.
3.3 Cellular uptake
The intracellular concentration of DNR was evaluated by FCM. DNR + GA and GA–PEG–PLGA–PLL + DNR can evidently enhance the intracellular density of DNR, with 1.56- and 2.40-fold relative fluorescence intensity compared with the naked DNR treatment (Fig. 6).
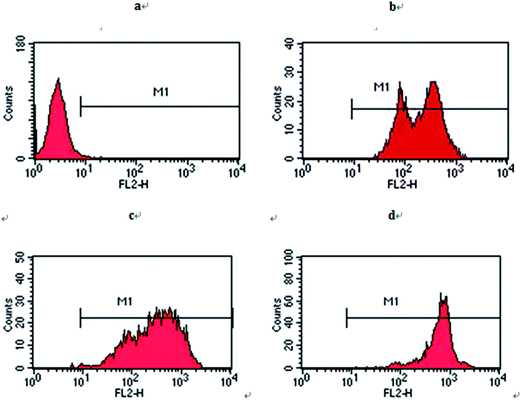 |
| Fig. 6 The intracellular concentration of DNR with different treatment evaluated by FCM. (a) control group; (b) DNR; (c) DNR + GA; (d) GA–PEG–PLGA–PLL + DNR. Abbreviations: DNR, daunorubicin; FCM, flow cytometry; GA, gambogic acid; PEG–PLGA–PLL, polyethylene glycol–polylactic-co-glycolic acid–poly-L-lysine. | |
3.4 Apoptosis of K562/A02 cells
FCM was used to quantitatively investigate the apoptosis of K562/A02 cells (Fig. 7). After incubation for 48 h, the total apoptosis was 5.12% ± 0.17% in the blank group. Additionally, after GA treatment at 0.0625 μM concentration, the apoptosis increased to 8.28% ± 0.36% but showed no significant difference with the control group, suggesting that GA at this density cannot induce apoptosis of K562/A02 cells. When the cells were cultured with DNR and DNR + GA, the apoptosis increased to 20.28% ± 0.45% and 42.26% ± 2.30%, respectively. Furthermore, when K562/A02 cells were treated by GA–PEG–PLGA–PLL and DNR, the rate of cell apoptosis increased to 72.44% ± 1.84%, which indicated a significant change compared with the control group. These results demonstrated that GA can increase the ability of DNR to induce apoptosis of K562/A02 cells. Moreover, when GA–PEG–PLGA–PLL was used with DNR, the apoptosis of cells remarkably increased, suggesting that PEG–PLGA–PLL can be utilised as a drug delivery system.
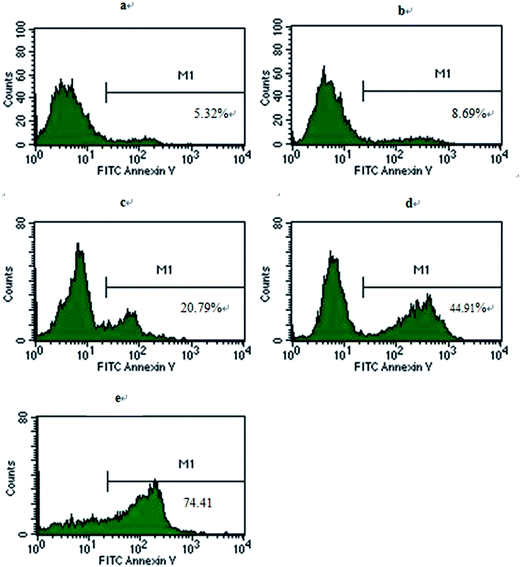 |
| Fig. 7 The apoptosis of K562/A02 cells with different treatment evaluated by FCM. (a) Control group; (b) GA; (c) DNR; (d) DNR + GA; (e) GA–PEG–PLGA–PLL + DNR. Abbreviations: FCM, flow cytometry; GA, gambogic acid; DNR, daunorubicin; PEG–PLGA–PLL, polyethylene glycol–polylactic-co-glycolic acid–poly-L-lysine. | |
3.5 Morphological changes in K562/A02 cells
To further determine whether PEG–PLGA–PLL and GA merely inhibited cell growth or induced cell death, morphological changes were observed under a fluorescence microscope after staining with DAPI. The cells treated with DNR showed morphological changes, such as condensation of chromatin, and the changes were more drastic when the cells were incubated with GA and DNR; these severe changes included shrinking of cells and alteration of membrane integrity (Fig. 8). Moreover, the cytoskeleton collapsed, and the total number of cells decreased. When the cells were treated with GA–PEG–PLGA–PLL + DNR, the morphological changes became more significant than those of any other groups, and the number of cells decreased.
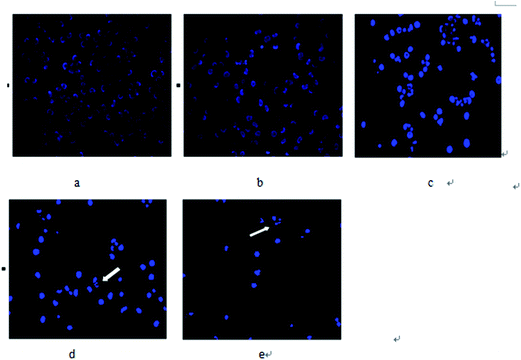 |
| Fig. 8 Morphological changes of K562/A02 cells with different treatment evaluated by fluorescence microscope. (a) control group; (b) GA; (c) DNR; (d) DNR + GA; (e) GA–PEG–PLGA–PLL + DNR. Abbreviations: GA, gambogic acid; DNR, daunorubicin; PEG–PLGA–PLL, polyethylene glycol–polylactic-co-glycolic acid–poly-L-lysine. | |
3.6 QPCR assay and Western blot assay
To obtain further insights into the mechanism of DNR, GA, DNR + GA and GA–PEG–PLGA–PLL with DNR in inducing the apoptosis of K562/A02 and reversing the resistance of K562/A02 to DNR, we detected the expression of P-gp and survivin by qPCR and Western blot.
We first examined the mRNA expression of P-gp and survivin in K562/A02 cells by using qPCR. The expression levels of survivin and P-gp decreased with the treatment of DNR + GA, and the suppression became more significant in the group of GA–PEG–PLGA–PLL with DNR (Fig. 9). The consequence corresponded to the Western blot assay, which showed that the group of GA with DNR and the group of GA–PEG–PLGA–PLL with DNR significantly downregulated the expression levels of P-gp and survivin protein compared with the control group treated only by GA or DNR (Fig. 10 and 11). These results suggested that GA–PEG–PLGA–PLL induced the apoptosis of K562/A02 cells and desensitised K562/A02 cells to DNR in the P-gp and survivin pathway.
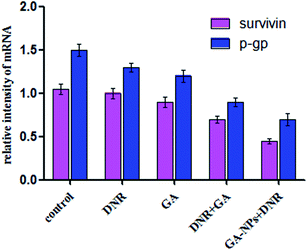 |
| Fig. 9 The expression of survivin and P-gp with different treatment evaluated by qPCR. Abbreviations: DNR, daunorubicin; GA, gambogic acid; NPs, polyethylene glycol–polylactic-co-glycolic acid–poly-L-lysine (PEG–PLGA–PLL); qPCR, quantitative real-time polymerase chain reaction. | |
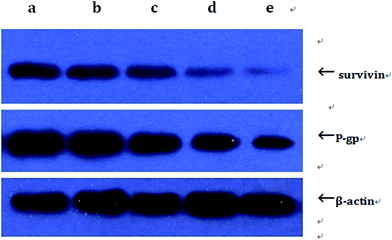 |
| Fig. 10 The expression of survivin and P-gp with different treatment evaluated by Western blot. (a) control group; (b) DNR; (c) GA; (d) DNR + GA; (e) GA–PEG–PLGA–PLL + DNR. Abbreviations: DNR, daunorubicin; GA, gambogic acid; PEG–PLGA–PLL, polyethylene glycol–polylactic-co-glycolic acid–poly-L-lysine. | |
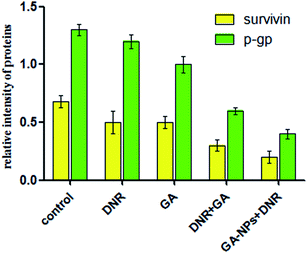 |
| Fig. 11 The expression of survivin and P-gp with different treatment evaluated by Western blot. Abbreviations: DNR, daunorubicin; GA, gambogic acid; NPs, polyethylene glycol–polylactic-co-glycolic acid–poly-L-lysine (PEG–PLGA–PLL). | |
4. Discussion
With the increasing incidence of leukaemia, many novel therapeutic drugs have been utilised in clinics. However, the phenomenon of MDR is the main obstacle for effective treatment. Despite much progress in understanding the pathophysiology of MDR to leukaemia and innovations to overcome MDR, no strategy is completely effective. Thus, every effort should be dedicated to overcome MDR. Moreover, multiple studies which demonstrated the efficacy of drugs carried by nanomaterials in desensitised resistance cells have inspired the current research.
Our study confirmed that GA can be encapsulated into the PEG–PLGA–PLL and have little effect on the PEG–PLGA–PLL, moreover, it can release much more in the environment of low pH. In additon, GA can enhance the therapeutic efficiency and desensitise the K562/A02 cell line to DNR with the presence of PEG–PLGA–PLL. Both growth inhibition to tumour cells and reverse index increased distinctively. Several studies have also verified this finding. Tianliang et al. demonstrated that when the concentration of GA was ≤0.0625 μmol L−1, no inhibition effect on proliferation of K562/A02 cells was found, which correspond with our current study. Additionally, the apoptotic index significantly increased after 48 h treatment with ADM combined with 0.0625 μmol L−1 GA−1. In conclusion, GA can enhance the sensitivity of K562/A02 to ADM and reserve the MDR.13
The prominent function of PEG–PLGA–PLL can be attributed to its inherent property.17 PLL is a linear polypeptide with the amino acid lysine as the repeat unit, and cationic polypeptide PLL can bind to the negatively charged cells, such as malignant cells; thus, PLL complexes demonstrate a target activity towards the tumor.18 Furthermore, cationic PLL can adjust and improve the performance of the carrier by introducing side chains or specific-targeted moiety to modify the NPs.19,20 The quick elimination of PLL from the circulation by reticuloendothelial system and undesirable cytotoxicity induced by high dose of PLL hindered the application.21 Therefore, PEG was modified to increase the circulation time and alleviate adverse effects. With water-soluble PEG, the circulation time of PEG–PLGA–PLL within the body can be prolonged by escaping from phagotrophy of the reticuloendothelial system.22–25 With approval from the Food and Drug Administration, the component of PLGA, which exhibits good biodegradability and biocompatibility, is often used as a drug delivery system because of its high drug encapsulation efficiency and drug loading efficiency.26,27 Owning to the prolonged circulation time and treatment targeting, the proliferation of K562/A02 cells were significantly inhibited with the treatment of GA–PEG–PLGA–PLL compared with naked DNR and DNR + GA, and a prominent decrease in side effects was observed. Moreover, PEG–PLGA–PLL and GA induced cell death instead of merely inhibiting cell growth. Finally, GA–PEG–PLGA–PLL lacked cytotoxicity, which was a prerequisite for successful further application.
The possible pathway leading to the apoptosis of K562/A02 cells was also investigated (Fig. 12). An affinity relationship was reported among P-gp, survivin and MDR.28–30 P-gp is an integral membrane protein with an important function in mediating classical MDR and functions as an adenosine triphosphate-dependent efflux pump. Therefore, the concentrations of cytotoxic agents within tumour cells are reduced, enabling the tumour cells to survive despite the chemotherapeutic treatment. P-gp was discovered for the first time in 1976 by Juliano et al., and they described P-gp as responsible for conferring MDR upon mutant-cultured cancer cells that developed resistance to cytotoxic drugs.31 Further reports have indicated that one prototypical MDR to anticancer agents was mediated by overexpressed P-gp, and this overexpression is usually considered as one of the major mechanisms contributing to MDR.32 The tumour cells with highly overexpressed P-gp are often related to inefficacy treatment, which showed an unidentified relationship with reoccurrence.
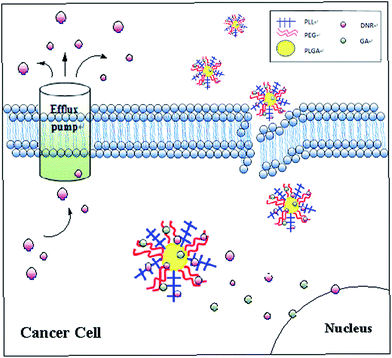 |
| Fig. 12 The distinguished improvement in anticancer activity of DNR delivered by GA–PEG–PLGA–PLL. On the left, DNR was removed from the cell via efflux pumps. On the right, GA–PEG–PLGA–PLL enabled DNR to pass the efflux pumps, then DNR and GA were released within the cells and entered the nucleus which caused apoptosis of K562/A02. | |
Survivin, a member of the inhibitor of apoptosis protein family, blocks apoptosis induced by various nonrelated apoptosis triggers, including different anticancer agents. In a variety of cancer cells, the expression of survivin was significantly higher after treatment with chemotherapeutic drugs, which participated in the resistance to chemotherapy.33 Studies have also suggested that survivin serves a key function in MDR in the presence of P-gp. The current results showed that the concentration of DNR within K562/A02 cells was greater in the group of GA–PEG–PLGA–PLL than in the groups treated with only DNR or DNR + GA. This finding indicated that the decreased expression of P-gp and survivin induced high levels of DNR in target cells. Therefore, reversed resistance of K562/A02 cells to DNR enhanced the efficiency of treatment.
5. Conclusion
PEG–PLGA–PLL was responsible for enhanced therapy related to prolonged circulation time and increased intracellular concentration of DNR, which also minimised the side effect with the tumour target. The decreased expression of P-gp and surviving, which was strongly associated with MDR, presented the possible mechanism of GA in desensitising K562/A02 cells to DNR. In conclusion, these findings identify PEG–PLGA–PLL in combination with GA as an effective tumour-targeted delivery tool which can overcome the resistance of K562/A02 cells to DNR and alleviate systemic side effects.
Conflict of interest
The authors declare no conflict of interest.
Acknowledgements
This work was supported by the National Natural Science Foundation of China (81400162), the Natural Science Foundation of Jiangsu Province (BK20140100) and Innovation Program of Jiangsu Province (SJZZ15_0029).
Notes and references
- R. Lozano, M. Naqhavi, K. Foreman, S. Lim, K. Shibuya, V. Aboyans, J. Abranham, T. Adair, R. Aggarwal, S. Y. Ahn, M. Alvarado, H. R. Anderson, L. M. Anderson and K. G. Andrews, Lancet, 2012, 380, 2095–2128 CrossRef.
- D. Colin, B. Cynthia, D. Alan and J. L. Christopher, Global Programme on Evidence for Health Policy Discussion Paper No. 13, World Health Organization.
- P. P. Wu, S. Li and H. J. Zhang, Drug Des., Dev. Ther., 2014, 8, 2431–2438 CAS.
- O. Chantarasriwong, A. Batova, W. Chavasiri and E. A. Theodorakis, Chemistry, 2010, 16, 9944–9962 CrossRef CAS PubMed.
- D. K. Yin, Y. Yang, H. X. Cai, F. Wang, D. Y. Peng and L. Q. He, Mol. Pharm., 2014, 11, 4107–4117 CrossRef CAS PubMed.
- A. Panthong, P. Norkaew, D. Kanjanapothi, T. Taesotikul, N. Anantachoke and V. Reutrakul, J. Ethnopharmacol., 2007, 111, 335–340 CrossRef PubMed.
- X. Wang, Y. Chen, Q. B. Han, C. Y. Chan, H. Wang, Z. Liu, C. H. Cheng, D. T. Yew, M. C. Lin, M. L. He, H. X. Xu, J. J. Sung and H. F. Kung, Proteomics, 2009, 9, 242–253 CrossRef CAS PubMed.
- C. Li, Q. Qi, N. Lu, Q. Dai, F. Li, X. Wang, Q. You and Q. Guo, Biochem. Cell Biol., 2012, 90, 718–730 CrossRef CAS PubMed.
- X. Zhu, H. Zhang, Y. Lin, P. Chen, J. Min, Z. Wang, W. Xiao and B. Chen, J. Chemother., 2009, 21, 666–672 CrossRef CAS PubMed.
- C. L. Ba, G. F. Guan, C. C. You, W. Zhao and K. Zhu, Orthop. J. China, 2011, 19, 2000–2002 CAS.
- X. J. Deng, Y. Gu and C. Lu, Jiangsu Med. J., 2011, 36, 2676–2678 Search PubMed.
- T. T. Wang, J. Wei, X. P. Qian, Y. T. Ding, L. X. Yu and B. R. Liu, Cancer Lett., 2009, 26, 214–222 Search PubMed.
- L. Tian, J. Liu, B. A. Chen, J. Cheng, J. H. Ding, S. Wang, G. H. Xia, F. Gao, Z. Y. Shao, H. J. Zhang, Q. L. Guo, H. W. Zhang, L. Wang, Y. Y. Ren, X. H. Cai and R. Liu, J. Exp. Hematol., 2012, 20, 252–257 CAS.
- B. Daglar, E. Ozgur, M. E. Corman, L. Uzund and G. B. Demirel, RSC Adv., 2014, 4, 48639 RSC.
- P. F. Liu, H. Yu, Y. Sun, M. J. Zhu and Y. R. Duan, Biomaterials, 2012, 33, 4403–4412 CrossRef CAS PubMed.
- L. T. Guo, B. A. Chen, R. Liu, P. Liu, G. H. Xia and Y. L. Wang, J. Nanosci. Nanotechnol., 2014, 14, 1–10 CrossRef PubMed.
- Y. F. Wang, P. F. Liu, L. H. Qiu, Y. Sun, M. Zhu, L. Gu, W. Di and Y. Duan, Biomaterials, 2013, 34, 4068–4077 CrossRef CAS PubMed.
- P. F. Liu, L. B. Qin, Q. Wang, Y. Sun, M. Zhu, M. Shen and Y. Duan, Biomaterials, 2012, 33, 6739–6747 CrossRef CAS PubMed.
- D. A. Barrera, E. Zylstra, P. T. Lansbury and R. Langer, J. Am. Chem. Soc., 1993, 115, 11010–11011 CrossRef CAS.
- D. A. Barrera, E. Zylstra, P. T. Lansbury and R. Lange, Macromolecules, 1995, 28, 425–432 CrossRef CAS.
- N. J. Caplen, E. W. Alton, P. G. Middleton, J. R. Dorin, B. J. Stevenson, X. Gao, S. R. Durham, P. K. Jeffery, M. E. Hodson and C. Coutelle, Nat. Med., 1995, 1, 39–46 CrossRef CAS PubMed.
- A. S. Karakoti, S. Das, S. Thevuthasan and S. Seal, Angew. Chem., Int. Ed., 2011, 50, 1980–1994 CrossRef CAS PubMed.
- D. P. Lankveld, R. G. Rayavarapu, P. Krystek, A. G. Oomen, H. W. Verharen, T. G. van Leeuwen, W. H. De Jong and S. Manohar, Nanomedicine, 2011, 6, 339–349 CrossRef CAS PubMed.
- L. L. Cai, N. Qiu, M. L. Xiang, R. Tong, J. Yan, L. He, J. Shi, T. Chen, J. Wen, W. Wang and L. Chen, Int. J. Nanomed., 2014, 9, 243–255 Search PubMed.
- R. A. Petros and J. M. DeSimone, Nat. Rev. Drug Discovery, 2010, 9, 615–627 CrossRef CAS PubMed.
- E. M. Enlow, C. Luft, M. E. Napier and J. M. DeSimone, Nano Lett., 2011, 11, 808–813 CrossRef CAS PubMed.
- C. T. Lo, P. R. Van Tassel and W. M. Saltzman, Biomaterials, 2010, 31, 3631–3642 CrossRef CAS PubMed.
- L. S. Karina, S. S. Paloma, N. Gabriela, M. C. Arthur, C. V. Flavia and C. M. Raquel, Leuk. Res., 2013, 37, 1350–1358 CrossRef PubMed.
- F. Liu, Z. H. Xie, G. P. Cai and Y. Y. Jiang, Biol. Pharm. Bull., 2007, 30, 2279–2283 CAS.
- X. Wang, R. X. Deng, Y. Lu, Q. Xu, M. Yan, D. Ye and W. Chen, Basic Clin. Pharmacol. Toxicol., 2013, 112, 25–33 CrossRef CAS PubMed.
- R. L. Juliano and V. Ling, Biochim. Biophys. Acta, Biomembr., 1976, 445, 152–162 CrossRef.
- G. Bahrieh, M. Erdem, E. Özgȕr, U. Gȕndȕz and H. Kȕlah, RSC Adv., 2014, 4, 44879–44887 RSC.
- F. Liu, Z. H. Xie, G. P. Cai and Y. Y. Jiang, Biol. Pharm. Bull., 2007, 30, 2279–2283 CAS.
|
This journal is © The Royal Society of Chemistry 2015 |