DOI:
10.1039/C5RA10447E
(Paper)
RSC Adv., 2015,
5, 64976-64982
Facile fabrication of a mpg-C3N4/TiO2 heterojunction photocatalyst with enhanced visible light photoactivity toward organic pollutant degradation
Received
2nd June 2015
, Accepted 22nd July 2015
First published on 22nd July 2015
Abstract
A facile hard template approach has been developed to prepare mpg-C3N4/TiO2 composites using SiO2 nanoparticles as a hard template and cyanamide as a precursor. The samples have been well characterized using X-ray diffraction (XRD), energy-dispersive X-ray spectroscopy (EDS), transmission electron microscopy (TEM), and ultraviolet-visible diffuse reflectance spectroscopy (UV-vis DRS). The results demonstrate that TiO2 nanoparticles sized 5–10 nm were distributed on the surface of mpg-C3N4 to form the mpg-C3N4/TiO2 heterojunction photocatalyst. In this way, the mpg-C3N4/TiO2 heterojunction catalyst has a porous structure and large surface area, which increases the contact area for pollutants. Rhodamine B (RhB) was used as a representative organic pollutant to evaluate the photocatalytic activity of the samples under visible light irradiation. The results show that the as-prepared mpg-C3N4/TiO2 heterojunction catalyst has a significantly enhanced visible light photocatalytic activity compared to pure mpg-C3N4 and that the degradation rate was 1.6 times higher than that of pure mpg-C3N4. The increased photocatalytic activity of the mpg-C3N4/TiO2 heterojunction catalyst can be attributed to the formation of the heterojunction between mpg-C3N4 and TiO2, which suppresses the recombination of photoinduced electron–hole pairs. Furthermore, based on systematic characterization and discussion, a possible photocatalytic mechanism for the excellent photocatalytic performance was proposed.
Introduction
In recent decades, environmental problems such as organic pollutants and toxic water pollutants produced by some industries have become more and more harmful to human health.1–3 Photocatalysis is highly expected to be a useful green technology for solving many current environmental and energy issues through its efficiency and broad applicability.4 With the steadily and fast growing fields of nanoscience and nanotechnology, various nanostructured semiconductor materials such as metal oxides, nitrides and sulfides have been exploited as photocatalysts for photocatalytic degradation of organic pollutants.5 Notably, TiO2 is widely studied due to its favorable optical and electronic properties, long-term stability, low cost, and nontoxicity. However, with a bandgap of 3.2 eV, TiO2 could only utilize radiation with a wavelength less than 390 nm, which only takes up 5% of the solar light. The narrow optical absorption range and the highly efficient recombination of photogenerated electrons and holes are the main factors responsible for the low energy efficiency.6 Therefore, lots of efforts have been devoted to extend the absorption edge of TiO2, such as the doping of TiO2 with metallic or nonmetallic elements to increase the visible light absorbance,7–9 and coupling with other semiconductors to increase the separation efficiency of photogenerated electron–hole pairs during photocatalysis.10,11 Considering that most light sources include plenty of visible light, coupling a wide-bandgap semiconductor with a small-bandgap inorganic semiconductor as a visible light sensitizer is a key method for enhancing the photocatalytic energy efficiency to develop new photocatalysts with good photogenerated charge separation as well as a wide response wavelength range.
Recently, graphitic carbon nitride (g-C3N4), a polymeric metal-free semiconductor with a band gap of about 2.70 eV, has been a focus of the photocatalytic fields such as water splitting and/or organic pollutant decomposition owing to its unique two-dimensional structure, excellent chemical stability and tunable electronic structure.12–14 However, the photocatalytic efficiency of g-C3N4 is still limited due to the high recombination rate of the photogenerated electron–hole pairs. Several methods have been developed to improve the photocatalytic performance of g-C3N4, for instance, dye sensitization, transition metal doping and coupling with a semiconductor.15–20 Specifically, g-C3N4 has a highest occupied molecular orbital (HOMO) which is located at −1.12 eV and is more negative than the conduction band (CB) of the common wide bandgap semiconductor photocatalysts such as ZnO and TiO2, which is favorable for forming heterojunctions with the wide bandgap semiconductors and thus for extending their visible light response.21 In particular, the fabrication of heterostructured composites by combining g-C3N4 with other semiconductors can not only restrict efficiently the recombination of photogenerated charge carriers, but can also endow the composites with novel characteristics or some enhanced properties from the synergistic effects or antagonistic effects.22,23 In addition, previous reports indicate that the porous structure introduced in g-C3N4 by soft or hard templates would make it possess an increased surface area and provide more active sites for adsorption and photocatalytic reactions, which are also beneficial to the enhancement of photocatalytic efficiency.24–26
In this paper, we demonstrate a facile hard template method to synthesize mpg-C3N4/TiO2 heterostructures. Herein, mesoporous g-C3N4 (mpg-C3N4) with an open crystalline pore wall and a large surface area was used as a support to disperse TiO2 nanoparticles. The mesoporous structure can in principle enhance the light harvesting ability and the reactant adsorption capability of the material due to its large surface area and multiple scattering effects. The photocatalytic activity of the mpg-C3N4/TiO2 heterostructures was carefully investigated using the degradation of Rhodamine B (RhB) dye under visible light irradiation. The results indicate that the as-prepared mpg-C3N4/TiO2 heterostructures exhibit a much higher visible light photocatalytic activity than that of bare mpg-C3N4 or TiO2 nanoparticles, which is attributed to the improvement in the separation efficiency of the photogenerated electron–hole pairs in the mpg-C3N4/TiO2 composites. Meanwhile, we also propose a possible mechanism for the enhanced activity of the mpg-C3N4/TiO2 composites on account of the experimental results.
Experimental section
Materials
Cyanamide (CN-NH2, 50%), a 40% dispersion of SiO2 particles (12 nm, Ludox HS40), titanium oxide (TiO2, 5–10 nm) and ammonium bifluoride (NH4HF2) were purchased from Aladdin Chemical Regent Co., Ltd (Shanghai, China). All the reagents in this study are analytically pure and were used without further purification.
Preparation of the mpg-C3N4/TiO2 heterostructures
In a typical procedure, 8.0 g of cyanamide (50% solution) was added dropwise to 24 g of a 40% dispersion of 12 nm SiO2 particles, which were used as a hard template, and then 0.5 g of TiO2 powder was added into the above solution with ultrasonication. The mixture was heated at 80 °C with stirring to evaporate the water. The resulting white powder was then heated at a rate of 2.3 °C min−1 over 4 h to reach a temperature of 550 °C, and then kept at this temperature for another 4 h. The brown-yellow product was treated with 4 M NH4HF2 for two days to remove the silica template. The powder was then centrifuged and washed with distilled water and ethanol several times. Finally the mpg-C3N4/TiO2 was dried at 70 °C under vacuum overnight. Meanwhile, a pure mpg-C3N4 sample was synthesised without the addition of TiO2 according to the previous report.27
Sample characterization
X-ray diffraction (XRD) patterns were recorded on an X-ray diffractometer (SmartLab, Rigaku) using Cu Kα radiation in the angle range of 10–80° (2θ). Then energy-dispersive X-ray spectroscopy (EDS) was used to analyze the elements of the as-prepared samples. The FT-IR spectra (KBr disc) of the as-prepared samples were recorded on a BRUKER-ALPHA FT-IR spectrometer. Transmission electron microscopy (TEM) and high resolution TEM (HRTEM) images were obtained with a JEM-2100 high-resolution transmission electron microscope. X-ray photoelectron spectroscopy (XPS) measurements were performed with a Kratos Axis Ultra DLD spectrometer equipped with a monochromatic Al Kα X-ray source (1486.6 eV). The nitrogen adsorption and desorption isotherms were measured at 77 K on an ASAP 2020 (Micromertics USA). UV-vis diffuse reflectance spectra (UV-vis DRS) were recorded on UV-vis spectrometer (UV-3650, Shimadzu) with an integrating sphere attachment. Here, BaSO4 was used as the reflectance standard material. The PL spectra were measured at room temperature on a Shimadzu RF-5301 fluorescence spectrophotometer with a 325 nm excitation wavelength.
Photocatalytic activity test
The photocatalytic reactions were carried out at room temperature in a Pyrex reactor with refluxing water to keep the temperature constant. A 500 W Xe lamp (Philips) was used as a simulated solar light source (UV-visible light), and a 420 nm cutoff filter was mounted on the lamp to eliminate infrared irradiation. Rhodamine B (RhB) with a concentration of 10 mg L−1 was used as the contaminant. In order to obtain an optimally dispersed system and reach complete adsorption–desorption equilibration, 100 mg of photocatalyst powder was dispersed in 100 mL of reaction solution by ultrasonicating for 10 min, and then the suspension was magnetically stirred in the dark for 1 h. Subsequently, the above suspension was irradiated using the 500 W xenon lamp. At regular intervals, a 3 mL portion of the suspension was sampled and filtered through a 0.45 μm PTFE syringe filter to get a clear liquid. Quantitative determination of the RhB in the supernatant was then performed by measuring the intensity of the absorption peak at 553 nm with a UV-vis spectrophotometer (UV-3600; Shimadzu).
Results and discussion
Structure and morphology
Fig. 1 shows the XRD patterns of the bare mpg-C3N4, TiO2 and the mpg-C3N4/TiO2 heterojunctions. As can be seen, the bare mpg-C3N4 shows two pronounced diffraction peaks of the (100) and (002) planes at 2θ ≈ 13.1° and 27.2°, which can be ascribed to the characteristic inter-layer structural packing and the interplanar stacking peaks of the aromatic systems, respectively.28 In the pure TiO2 sample, the strong diffraction peaks at 2θ = 25.34°, 37.82°, 48.08°, 53.94°, 55.10°, 62.78° and 68.80° can be assigned to the (101), (004), (200), (105), (211), (204) and (116) crystal planes of pure TiO2 with an anatase phase (JCPDS 21-1272), respectively.29 The mpg-C3N4/TiO2 heterojunction samples exhibit diffraction peaks corresponding to both mpg-C3N4 and TiO2, reflecting the presence of two phases, and no other impurity peaks were observed. In addition, EDS analysis was employed to investigate the composition of the heterojunctions (in Fig. 1b), where the appeared peaks confirmed that the product was only composed of C, N, O and Ti elements.
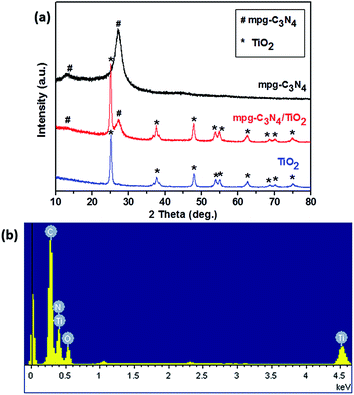 |
| Fig. 1 XRD patterns of (a) mpg-C3N4, TiO2 and the mpg-C3N4/TiO2 heterojunctions, and an EDS spectrum of (b) the mpg-C3N4/TiO2. | |
The morphologies of the mpg-C3N4, pure TiO2 and mpg-C3N4/TiO2 heterojunctions were investigated using transmission electron microscopy (TEM) and high resolution TEM (HRTEM), as shown in Fig. 2. As shown in Fig. 2a, mpg-C3N4 displays a 2D lamellar structure and many spherical pores with a mean diameter of about 12 nm are observed on the surface. These pores exactly reflect the geometric properties of the original template of SiO2 particles with a mean diameter of 12 nm. Fig. 2b displays the irregular spheres of the TiO2 particles with diameters in the range of 5–10 nm. For the mpg-C3N4/TiO2 heterojunctions (Fig. 2c), the TiO2 nanoparticles are found embedded in the mpg-C3N4 lamellar structure. The corresponding HRTEM image which is shown in Fig. 2d clearly reveals the close interfacial connections between mpg-C3N4 and the TiO2 nanoparticles. By measuring the lattice fringes in the HRTEM image, the resolved interplanar distance of 0.35 nm agrees well with the lattice spacing of the (101) plane of anatase TiO2. These observations suggest the formation of a heterojunction between TiO2 and mpg-C3N4 which would be an ideal system to achieve improved electron–hole separation via a smooth charge transfer process.
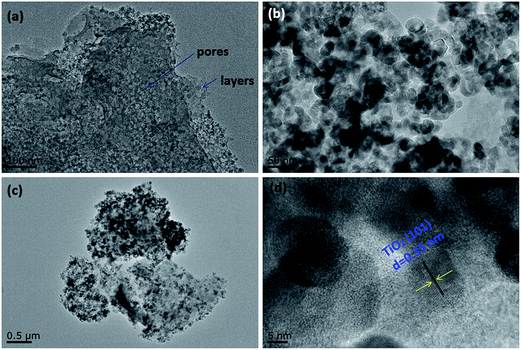 |
| Fig. 2 Typical TEM images of (a) mpg-C3N4, (b) pure TiO2 and (c) the mpg-C3N4/TiO2 heterojunctions, and an HRTEM image (d) of the as-prepared mpg-C3N4/TiO2 heterojunctions. | |
In order to investigate the interactions between the TiO2 and mpg-C3N4 in the as-prepared mpg-C3N4/TiO2 heterojunctions, FTIR spectra were obtained and are presented in Fig. 3. The pure mpg-C3N4 shows characteristic IR peaks similar to those in previous results.18,30 The broad peak at 3000–3600 cm−1 is contributed by N–H stretching;31 the strong band at 1240–1640 cm−1 is attributed to the typical stretching vibrations of C–N bonds and C@N heterocycles;32,33 and the band near 808 cm−1 can be attributed to the characteristic bending mode of triazine units.34 It can be seen that a band around 500–700 cm−1 is clearly shown in the FTIR spectrum of TiO2, which corresponds to the Ti–O–Ti stretching vibration.35 The major characteristic peaks of TiO2 and mpg-C3N4 almost all appear in the mpg-C3N4/TiO2 sample, further indicating the formation of mpg-C3N4/TiO2 heterojunctions.
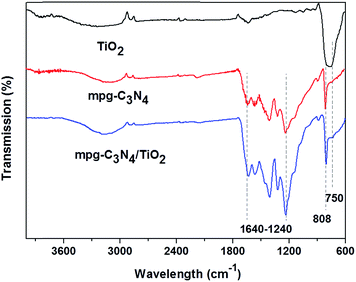 |
| Fig. 3 FTIR spectra of the as-prepared mpg-C3N4, TiO2 and mpg-C3N4/TiO2 heterojunctions. | |
XPS measurements were carried out to obtain information on the oxidation state and surface chemical composition of the mpg-C3N4/TiO2 heterojunction sample. The survey spectrum in Fig. 4a shows peaks of Ti, O, C, and N for the mpg-C3N4/TiO2 composite. Fig. 4b shows a high resolution spectrum of the Ti 2p transition. The Ti 2p peak is composed of spin doublets and the Ti 2p peak has two main components as determined by the fitting of the curve. The values of the binding energies (BE) obtained for the Ti 2p3/2 peaks of 455.6 eV and 457.1 eV verify the presence of Ti–N and an intermediate phase, respectively.36 Accordingly Ti 2p1/2 peaks were observed at 461.4 eV and 462.8 eV, respectively. The intermediate phase could be attributed to Ti2O3 or oxynitrides,37 although their existence is still debated.38,39 The XPS results together with those from the TEM and FTIR studies clearly reveal the formation of a composite material with chemically bound interfaces between mpg-C3N4 and TiO2.
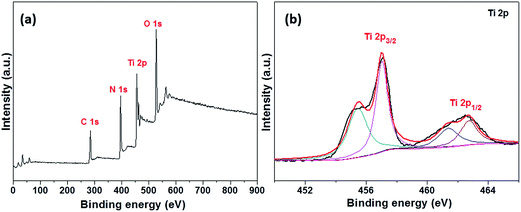 |
| Fig. 4 (a) XPS survey scan of the mpg-C3N4/TiO2 heterojunctions, and the corresponding Ti 2p high-resolution spectrum (b). | |
In order to obtain more information on the porous structures, the as-prepared mpg-C3N4/TiO2 heterojunction sample was examined using N2 sorption analysis. The N2 adsorption–desorption isotherms of the sample are illustrated in Fig. 5. It can be observed that the mpg-C3N4/TiO2 heterojunction sample has a type IV isotherm and type H3 hysteresis loop according to the IUPAC classifications, which indicates a mesoporous structure of the sample.40 This is almost consistent with the TEM observations. Based on the desorption curve, the Brunauer–Emmett–Teller (BET) surface area of the porous mpg-C3N4/TiO2 sample was calculated to be 106.42 m2 g−1, the total pore volume was 0.3105 cm3 g−1, and the average pore diameter was 10.4 nm.
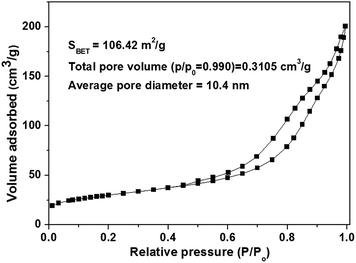 |
| Fig. 5 N2 adsorption–desorption isotherms of the as-prepared mpg-C3N4/TiO2 heterojunctions. | |
Fig. 6a shows UV-vis diffuse reflectance spectra of the mpg-C3N4, TiO2 and mpg-C3N4/TiO2 heterojunction samples. For comparison, the absorption spectra of all the samples were obtained under identical conditions. As illustrated in Fig. 6a, for pure TiO2 the absorption occurs at wavelengths shorter than 390 nm, consistent with the intrinsic band gap of anatase TiO2 (about 3.2 eV), while the absorption intensity of the pure mpg-C3N4 rises rapidly at about 450 nm, in good accordance with the band gap of g-C3N4 (2.7 eV).41 Compared with pure TiO2, the mpg-C3N4/TiO2 heterojunctions show an additional absorption in the region of visible light, attributed to the existence of mpg-C3N4. Fig. 6b illustrates the PL emission spectra of the as-prepared samples obtained using an excitation wavelength of 325 nm. PL spectral analysis was applied to investigate the recombination rate of the photogenerated electron–hole pairs. It is generally believed that a lower PL emission intensity is an indication of a lower recombination of photogenerated electron–hole pairs. As can be seen, pure mpg-C3N4 has a strong peak around 460 nm in the PL spectrum, which can be attributed to the band–band PL phenomenon, i.e. the emission light energy is approximately equal to the bandgap energy of g-C3N4. Once the TiO2 nanoparticles were anchored on the mpg-C3N4 nanosheets, the emission intensity of the PL spectrum decreased significantly, suggesting that the mpg-C3N4/TiO2 heterojunctions have a lower recombination rate of photo-generated electron–hole pairs than mpg-C3N4. This result shows a good agreement with those of other heterojunction semiconductors.22,23
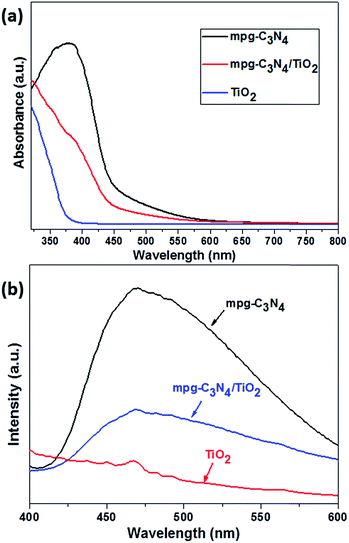 |
| Fig. 6 Optical properties of mpg-C3N4, TiO2 and the mpg-C3N4/TiO2 heterojunctions. (a) UV-vis diffuse reflectance spectra and (b) PL emission spectra obtained using an excitation wavelength of 325 nm. | |
Photocatalytic activity
The Fig. 7a inset shows the RhB adsorption capacities of the samples, for the adsorption process in the dark. The absorbance values of the RhB decreased by 31.6%, 35.7% and 36.8% due to absorption of RhB molecules by the TiO2, mpg-C3N4 and mpg-C3N4/TiO2 samples, respectively. The photocatalytic activity of the mpg-C3N4, TiO2 and mpg-C3N4/TiO2 heterojunctions was examined using visible-light degradation of RhB molecules in aqueous solution, where C is the concentration of RhB remaining in the solution after the irradiation time t, and Co is the initial concentration of RhB. The results are shown in Fig. 7a. The blank experiment demonstrates that decomposition of RhB is inappreciable within the test period in the absence of photocatalysts, suggesting that the photolysis of RhB is negligible. As can be seen from Fig. 7a, the pure TiO2 and mpg-C3N4 sample displayed a certain photocatalytic efficiency of 21% and 88% after visible-light irradiation for 100 min, respectively. As expected, the mpg-C3N4/TiO2 heterojunctions exhibited higher photocatalytic activity than the pure mpg-C3N4 sample, and provided 96.5% degradation of the RhB under visible light irradiation. Fig. 7b reveals that the photocatalytic degradation of RhB on the different catalysts fits pseudo-first-order kinetics, ln(Co/C) = kt, where C is the concentration of RhB at time t, Co is the initial concentration of the RhB solution, and the slope k is the apparent reaction rate constant. The results imply that the k value for the mpg-C3N4/TiO2 sample is higher than those of the pure mpg-C3N4 and TiO2. It can be found that the k value of the mpg-C3N4/TiO2 sample (0.0335 min−1) is about 1.6 times that of mpg-C3N4 (0.0214 min−1) and about 14 times that of TiO2 (0.0024 min−1). These results indicate that the formation of the heterojunction structure of mpg-C3N4/TiO2 could greatly enhance the photocatalytic efficiency of singular mpg-C3N4 and TiO2. For evaluating the recyclability of the mpg-C3N4/TiO2 sample, additional experiments were carried out to degrade RhB under visible light four times in a cycle (Fig. 8). The photocatalytic activity of the mpg-C3N4/TiO2 heterojunction sample for RhB degradation decreased from 96.5% to 88.5% after four cycling runs, which demonstrates that the mpg-C3N4/TiO2 heterojunction sample has a high stability in the photocatalytic process under visible light irradiation.
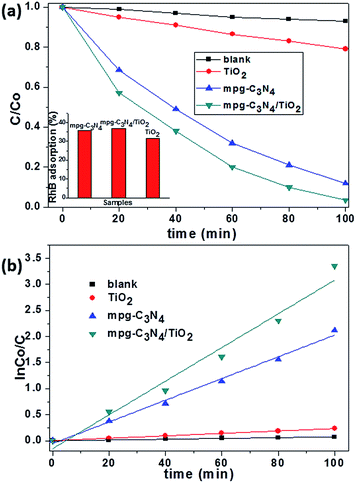 |
| Fig. 7 Photocatalytic activities (a), adsorption capacities (inset), and kinetics (b) of the mpg-C3N4, TiO2 and mpg-C3N4/TiO2 heterojunctions for degradation of RhB under visible light irradiation. | |
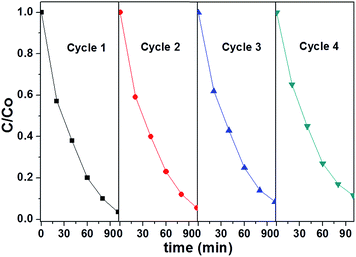 |
| Fig. 8 Four cycles of photocatalytic degradation of RhB by the mpg-C3N4/TiO2 heterojunctions under visible light irradiation. | |
Proposed mechanism for the enhanced photocatalytic activity
On the basis of the above results and discussion, a photocatalytic mechanism for the reaction under visible light irradiation was tentatively proposed and is schematically illustrated in Fig. 9. When the as-prepared mpg-C3N4/TiO2 heterojunctions are irradiated with visible light, the electrons get promoted from the valence band (VB) to the conduction band (CB) in mpg-C3N4, providing a route for the electronic transition. According to previous studies, the CB and VB edge potentials of mpg-C3N4 were at −1.12 and 1.57 eV, respectively.21 The CB and VB edge potentials of TiO2 were at −0.29 and 2.91 eV, respectively.42 The CB edge potential of mpg-C3N4 is more negative than that of TiO2, allowing the excited electron on the surface of mpg-C3N4 to transfer easily to TiO2 via the well-built heterojunction. The recombination of the photogenerated charge is inhibited and the photocatalytic activities are enhanced effectively for TiO2 which provides a site for electron translation. Then, the electrons stored in the CB of TiO2 are trapped by the air near the surface of the TiO2 to form reactive ˙O2−. Besides, the EVB (g-C3N4, +1.57 eV vs. SHE) values were lower than the standard redox potential of ˙OH/H2O (+2.68 eV vs. SHE), indicating that the photogenerated holes on mpg-C3N4 could not oxidize H2O to the active species ˙OH.43,44 Therefore, h+ on the VB of mpg-C3N4 would react with RhB directly. On the other hand, as the electrons in the CB of TiO2 (−0.29 eV) are located at more negative potential positions than the O2/˙O2− couple (−0.046 eV), electrons stored in the CB of TiO2 are trapped by O2 dissolved in the solution near the surface of the TiO2 to generate reactive superoxide radical ions (˙O2−), while the holes in the VB of mpg-C3N4 directly oxidize the pollutants.45 Furthermore, the previously mentioned characterization details indicate that coupling TiO2 with mpg-C3N4 produces a well-contacted solid–solid heterojunction interface between the mpg-C3N4 and TiO2 semiconductor particles, which promotes effective interfacial electron transfer and separation of the photogenerated charge carriers, significantly enhancing photocatalytic activity.46
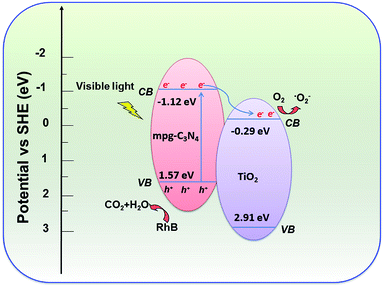 |
| Fig. 9 Proposed photocatalytic mechanism for the degradation of RhB over the mpg-C3N4/TiO2 heterojunctions under visible light irradiation. | |
Conclusions
In summary, we have successfully fabricated mpg-C3N4/TiO2 heterojunctions using SiO2 nanoparticles as a hard template and cyanamide as a precursor via a facile hard template approach. The obtained mpg-C3N4/TiO2 heterojunctions exhibited enhanced photocatalytic activity toward RhB degradation under visible light irradiation compared to that of pure mpg-C3N4 and TiO2 nanoparticles. This enhancement of the photocatalytic activity has been demonstrated to be due to the high separation and easy transfer of photogenerated electron–hole pairs at the heterojunction interfaces derived from the match in energy levels between the TiO2 and mpg-C3N4. Thus, the mpg-C3N4/TiO2 heterojunctions can be a promising candidate for efficient visible light driven photocatalytic systems for organic pollution residue removal.
Acknowledgements
The authors are grateful for the financial support of the National Natural Science Foundation of China (grant no. 21376051, 21306023, 21106017), the Natural Science Foundation of Jiangsu (grant no. BK20131288), the Fund Project for Transformation of Scientific and Technological Achievements of Jiangsu Province of China (grant no. BA2014100) and the Fundamental Research Funds for the Central Universities (3207045301, T15192014, KYLX_0161).
References
- J. M. Hall-Spencer, R. Rodolfo-Metalpa, S. Martin, E. Ransome, M. Fine, S. M. Turner, S. J. Rowley, D. Tedesco and M.-C. Buia, Nature, 2008, 454, 96–99 CrossRef CAS PubMed.
- M. A. Shannon, P. W. Bohn, M. Elimelech, J. G. Georgiadis, B. J. Mariñas and A. M. Mayes, Nature, 2008, 452, 301–310 CrossRef CAS PubMed.
- C. J. Vörösmarty, P. McIntyre, M. O. Gessner, D. Dudgeon, A. Prusevich, P. Green, S. Glidden, S. E. Bunn, C. A. Sullivan and C. R. Liermann, Nature, 2010, 467, 555–561 CrossRef PubMed.
- M. R. Hoffmann, S. T. Martin, W. Choi and D. W. Bahnemann, Chem. Rev., 1995, 95, 69–96 CrossRef CAS.
- M. D. Hernandez-Alonso, F. Fresno, S. Suarez and J. M. Coronado, Energy Environ. Sci., 2009, 2, 1231–1257 CAS.
- V. Subramanian, E. Wolf and P. V. Kamat, J. Phys. Chem. B, 2001, 105, 11439–11446 CrossRef CAS.
- L. Fan, J. Dongmei and M. Xueming, J. Alloys Compd., 2009, 470, 375–378 CrossRef CAS PubMed.
- S.-Z. Chen, P.-Y. Zhang, D.-M. Zhuang and W.-P. Zhu, Catal. Commun., 2004, 5, 677–680 CrossRef CAS PubMed.
- F. Dong, W. Zhao and Z. Wu, Nanotechnology, 2008, 19, 365607 CrossRef PubMed.
- R. Jaiswal, N. Patel, D. Kothari and A. Miotello, Appl. Catal., B, 2012, 126, 47–54 CrossRef CAS PubMed.
- X. Cheng, X. Yu, Z. Xing and J. Wan, Energy Procedia, 2012, 16, 598–605 CrossRef CAS PubMed.
- Z. Zhao, Y. Sun and F. Dong, Nanoscale, 2015, 7, 15–37 RSC.
- X. Wang, K. Maeda, X. Chen, K. Takanabe, K. Domen, Y. Hou, X. Fu and M. Antonietti, J. Am. Chem. Soc., 2009, 131, 1680–1681 CrossRef CAS PubMed.
- J. Yu, S. Wang, B. Cheng, Z. Lin and F. Huang, Catal. Sci. Technol., 2013, 3, 1782–1789 CAS.
- K. Takanabe, K. Kamata, X. Wang, M. Antonietti, J. Kubota and K. Domen, Phys. Chem. Chem. Phys., 2010, 12, 13020–13025 RSC.
- Z. Ding, X. Chen, M. Antonietti and X. Wang, ChemSusChem, 2011, 4, 274–281 CAS.
- Y. Di, X. Wang, A. Thomas and M. Antonietti, ChemCatChem, 2010, 2, 834–838 CrossRef CAS PubMed.
- Y. Wang, R. Shi, J. Lin and Y. Zhu, Energy Environ. Sci., 2011, 4, 2922–2929 CAS.
- Y. Wang, X. Bai, C. Pan, J. He and Y. Zhu, J. Mater. Chem., 2012, 22, 11568–11573 RSC.
- C. Pan, J. Xu, Y. Wang, D. Li and Y. Zhu, Adv. Funct. Mater., 2012, 22, 1518–1524 CrossRef CAS PubMed.
- X. Wang, K. Maeda, A. Thomas, K. Takanabe, G. Xin, J. M. Carlsson, K. Domen and M. Antonietti, Nat. Mater., 2009, 8, 76–80 CrossRef CAS PubMed.
- M. Xu, L. Han and S. Dong, ACS Appl. Mater. Interfaces, 2013, 5, 12533–12540 CAS.
- D. Chen, K. Wang, T. Ren, H. Ding and Y. Zhu, Dalton Trans., 2014, 43, 13105–13114 RSC.
- D. Jiang, L. Chen, J. Zhu, M. Chen, W. Shi and J. Xie, Dalton Trans., 2013, 42, 15726–15734 RSC.
- J. Hong, S. Yin, Y. Pan, J. Han, T. Zhou and R. Xu, Nanoscale, 2014, 6, 14984–14990 RSC.
- J. Shen, H. Yang, Q. Shen, Y. Feng and Q. Cai, CrystEngComm, 2014, 16, 1868–1872 RSC.
- F. Goettmann, A. Fischer, M. Antonietti and A. Thomas, Angew. Chem., Int. Ed., 2006, 45, 4467–4471 CrossRef CAS PubMed.
- S. Yan, Z. Li and Z. Zou, Langmuir, 2010, 26, 3894–3901 CrossRef CAS PubMed.
- X. Zhou, B. Jin, L. Li, F. Peng, H. Wang, H. Yu and Y. Fang, J. Mater. Chem., 2012, 22, 17900–17905 RSC.
- B. Chai, T. Peng, J. Mao, K. Li and L. Zan, Phys. Chem. Chem. Phys., 2012, 14, 16745–16752 RSC.
- Y. Yan, B. Hao, X. Wang and G. Chen, Dalton Trans., 2013, 42, 12179–12184 RSC.
- F. Dong, L. Wu, Y. Sun, M. Fu, Z. Wu and S. Lee, J. Mater. Chem., 2011, 21, 15171–15174 RSC.
- M. J. Bojdys, J. O. Müller, M. Antonietti and A. Thomas, Chem.–Eur. J., 2008, 14, 8177–8182 CrossRef CAS PubMed.
- Y. Li, H. Zhang, P. Liu, D. Wang, Y. Li and H. Zhao, Small, 2013, 9, 3336–3344 CAS.
- C. Liu, D. Yang, Y. Jiao, Y. Tian, Y. Wang and Z. Jiang, ACS Appl. Mater. Interfaces, 2013, 5, 3824–3832 CAS.
- A. Vesel, M. Mozetic, J. Kovac and A. Zalar, Appl. Surf. Sci., 2006, 253, 2941–2946 CrossRef CAS PubMed.
- J. Halbritter, H. Leiste, H. J. Mathes and P. Walk, Fresenius’ J. Anal. Chem., 1991, 341, 320–324 CrossRef CAS.
- H. C. Man, Z. D. Cui and X. J. Yang, Appl. Surf. Sci., 2002, 199, 293–302 CrossRef CAS.
- J. Kovac, G. Scarel, M. Sancrotti, M. G. Beghi, C. E. Bottani, P. M. Ossi, L. Calliari, M. Bonelli and A. Miotello, J. Appl. Phys., 1999, 86, 5566–5572 CrossRef CAS PubMed.
- K. S. Sing, Pure Appl. Chem., 1985, 57, 603–619 CrossRef CAS.
- Q. Sun, K. Lv, Z. Zhang, M. Li and B. Li, Appl. Catal., B, 2015, 164, 420–427 CrossRef PubMed.
- S. Zhao, S. Chen, H. Yu and X. Quan, Sep. Purif. Technol., 2012, 99, 50–54 CrossRef CAS PubMed.
- L. Shi, L. Liang, J. Ma, F. Wang and J. Sun, Catal. Sci. Technol., 2014, 4, 758–765 CAS.
- S. Kumar, T. Surendar, A. Baruah and V. Shanker, J. Mater. Chem. A, 2013, 1, 5333–5340 CAS.
- Y. F. Chen, W. X. Huang, D. L. He, S. T. Yue and H. Huang, ACS Appl. Mater. Interfaces, 2014, 6, 14405–14414 CAS.
- K. Kondo, N. Murakami, C. Ye, T. Tsubota and T. Ohno, Appl. Catal., B, 2013, 142, 362–367 CrossRef PubMed.
Footnote |
† S. S. Ma and J. J. Xue contributed equally to this work. |
|
This journal is © The Royal Society of Chemistry 2015 |