DOI:
10.1039/C5RA10240E
(Paper)
RSC Adv., 2015,
5, 68500-68509
Synthesis and characterization of diphenylcarbazide-siliceous mesocellular foam and its application as a novel mesoporous sorbent for preconcentration and trace detection of copper and cadmium ions†
Received
30th May 2015
, Accepted 3rd August 2015
First published on 6th August 2015
Abstract
We are introducing diphenylcarbazide functionalized siliceous mesocellular foam as a novel mesoporous solid-phase for the extraction of heavy metal ions including copper(II) and cadmium(II). The synthesized mesoporous sorbent was characterized using Fourier transform infrared spectrometry, scanning electron microscopy, elemental analysis, nitrogen adsorption–desorption isotherms and thermal analysis. Determination of the extracted ions was performed by flame atomic absorption spectrophotometry. Effects of pH value, adsorption and desorption time, type, concentration and volume of the eluent, breakthrough volume, and effect of potentially interfering ions were studied. Under optimized conditions, the extraction efficiency is >98%, and the limits of detection are 0.1, and 0.04 μg L−1 for the ions of copper and cadmium, respectively, and the adsorption capacities for these ions are 160 and 190 mg g−1. The obtained data for adsorption capacity of the sorbent shows the high tendency of the sorbent toward the mentioned target ions. Finally, this sorbent can be used as a simple, rapid, reliable, selective and sensitive method for the determination of trace levels of copper(II) and cadmium(II) in different water samples.
1. Introduction
Heavy metals such as cadmium and copper ions are common pollutants of water, food, soil and biological samples and have generated intense research interest due to their toxicity to humans, animals, and other living creatures.1–4 Hence, the development of fast, reliable and effective analytical methods for the determination of trace amounts of cadmium and copper in real samples is an important area of research.5–9
Several analytical techniques, including flame atomic absorption spectrometry (FAAS), electrothermal atomic absorption spectrometry (ETAAS), inductively coupled plasma optical emission spectrometry (ICP-OES) and inductively coupled plasma-mass spectrometry (ICP-MS) and electroanalytical instruments are widely used for the determination of trace amounts of heavy metal ions in various matrices.10–16 However, the direct determination of trace amounts of these ions in real samples using these techniques is challenging due to matrix effects and the need for extremely low detection limits. Hence, sample pre-treatment, such as preconcentration of the analyzed elements and matrix separation, is often necessary.17,18
A number of separation and preconcentration procedures, such as solid phase extraction (SPE),19,20 coprecipitation,21 cloud point extraction (CPE)22 and liquid–liquid extraction (LLE),23 have been developed and used for the enrichment and separation of heavy metals at trace levels in various environmental samples. Among these, solid phase extraction is widely accepted as an ideal, and a powerful technique because of its simplicity, high enrichment factor, low cost, low or no consumption of organic solvents, ease of automation and ability to be coupled with various modern detection techniques.24
Siliceous mesostructured cellular foams (MCF) is a novel mesoporous silica material template by oil-in-water microemulsions with high surface areas and well defined pore structures have attracted considerable attention for applications in catalysis, adsorption and separations.25 Compared to MCM-41 and SBA-15, MCFs prepared with the microemulsion templating process by using 1,3,5-trimethylbenzene (TMB) as the organic swelling agent has a well-defined ultra large pores (24–42 nm), a narrow pore size distribution, and three dimensional (3-D) pore structures. Furthermore, MCFs with uniform spherical pores interconnected by windows of around 9–22 nm have high BET surface areas (up to 1000 m2 g−1). Their 3-D pores are substantially larger than those of the ordered counterparts SBA-15 and MCM-41. MCFs can provide more favorable conditions for mass diffusion.26
In this work, we have prepared organic–inorganic based conjugate material as an adsorbent for efficient extraction and preconcentration of cadmium and copper ions from water solutions. For preparation of the new class conjugate adsorbent, a specific functional group of organic ligand (diphenylcarbazide) was successfully incorporated into siliceous mesocellular foam. The synthesized sorbent was characterized by Fourier transform infrared spectroscopy, scanning electron microscopy, elemental analysis, nitrogen adsorption–desorption isotherm and thermal and elemental analyses. The effects of pH, flow rates, type, concentration and volume of eluent for simultaneous elution of copper and cadmium ions, break through volume and effect of coexisting ions on the separation and determination of these heavy metals were investigated. The developed method was applied for determination of copper and cadmium ions in several real samples, and the accuracy of the method was confirmed by standard reference material.
2. Experimental
2.1. Chemicals
Triblock copolymer poly(ethylene oxide)-b-poly(propylene oxide)-b-poly(ethylene oxide), Pluronic P123 (MW = 5800), was purchased from Aldrich Chemical Inc. Tetraethyl orthosilicate (TEOS), ammonium fluoride, 1,3,5-trimethylbenzene (TMB), hydrogen chloride (HCl), (3-chloropropyl)triethoxysilane, 1,5-diphenylcarbazide, triethylamin (TEA) were purchased from Aldrich Chemical Inc. Toluene and acetone ware obtained from Mujallali Company (Tehran, Iran). All chemicals were used as received without any further purification.
2.2. Instrumentation
Copper and cadmium concentration was determined by an AA-680 Shimadzu (Kyoto, Japan) flame atomic absorption spectrometer (FAAS) in an air–acetylene flame, according to the user's manual provided by the manufacturer. Copper and cadmium hollow cathode lamps (HCL) were used as the radiation source with wavelengths of 324.8 and 228.8 nm, respectively. The pH was measured at 25 ± 1 °C with a digital Metrohm 827 Ion Analyzer (Herisau, Switzerland) equipped with a combined glass–calomel electrode. Scanning electron microscopy (SEM) was performed with a JEOL JSM-7400F electron microscope. The nitrogen adsorption–desorption isotherms were obtained using a BELSORP-mini system; the sample was degassed at 150 °C for 10 h before analysis. Thermal analysis (TGA-DTA) was carried out on a Bahr STA-503 instrument in air at a heating rate of 10 °C min−1 up to 800 °C. The nitrogen and sulfur contents in the modified MCF were obtained with a Thermo Finnigan Flash-1112EA microanalyzer. Infrared spectra were recorded (KBr pellets) on an 8700 Shimadzu Fourier transform infrared (FT-IR) spectrophotometer.
2.3. Synthesis of spherical siliceous mesocellular foam
Spherical MCF sample was synthesized by modifying the conventional MCF synthesis method.27 In a typical preparation, 4.0 g of triblock copolymer Pluronic P123 were dissolved in an acidic solution (10 mL of HCl (37%) and 65 mL of H2O). A total of 4.0 g of 1,3,5-trimethylbenzene (TMB) were then added, and the resulting solution was heated to 37–40 °C with vigorous stirring for 2 h to synthesize the microemulsion (template). A total of 9.2 mL of tetraethoxysilane (TEOS) was then added and stirred for 5 min. The solution was transferred to an autoclave and aged at 40 °C for 20 h under a quiescent condition. A total of 46 mg of NH4F was added, and the mixture was aged at 100 °C for another 24 h. The resulting precipitate was filtered, washed with water and ethanol, and dried. The white powder obtained was calcined in air at 550 °C for 6 h.
2.4. Preparation of diphenylcarbazide functionalized MCF
In this approach, in a typical reaction,28 1.0 g of MCF was suspended in 80 mL dried toluene, and 3-chloro-propyltriethoxysilane (4.0 mL) was added to mixture and was refluxed for 48 h under nitrogen atmosphere. The resulted white solid was suspended in 100 mL of toluene and triethylamine mixture (3
:
1 v/v) and an excess amount of 1,5-diphenylcarbazide (1.0 g) solved in the minimum amount of acetone and then added to the reaction mixture. After 24 h reflux the resulted solid was removed from solvent by filtration, washed with methanol and acetone and then dried at room temperature. FT-IR spectroscopy, SEM, elemental and thermal analysis, and BET surface area measurement confirmed the synthesis of MCF-diphenylcarbazide.
2.5. Real sample pretreatment
The tested water samples were tap water (Tehran, Iran), sea water (Persian Gulf and Caspian Sea) and lake water. The water samples were collected in polyethylene bottles. They were cleaned with acid bath, and then filtered through nylon filters (Millipore, 0.22 μm) before the analysis. Certified reference material (0.1 g) were digested using 8 mL mixture of 5% aqua regia with the assistance of a microwave digestion system. Digestion was carried out for 2 min at 250 W, 2 min at 0 W, 6 min at 250 W, 5 min at 400 W, and 8 min at 550 W, and the mixture was then vented for 8 min. The residue from the digestion, as well as a controlled digestion was then diluted with deionized water. Finally, the pH of each solution was adjusted to 7.0 by drop wise addition of HCl and NaOH for separation and preconcentration of cadmium and copper ions from the water samples.
2.6. Solid phase extraction procedure
Batch experiments were used for adsorption and desorption studies. The dried synthesized sorbent (10 mg) was immersed in a solution containing 2 mg L−1 concentration of copper and cadmium ion. The pH of solution was adjusted to 7.0. After stirring for 5 min, the solution was centrifuged and ultrapure water was used for washing of adsorbed copper and cadmium ions. The solid mesoporous sorbent was suspended in 2 mL of HCl (1 mol L−1) as the eluent and stirred for 2 min. The resulted solution was subsequently centrifuged and separated, and the concentration of copper and cadmium ions was measured by FAAS.
3. Results and discussion
3.1. Characterization of the synthesized sorbent
MCF has a unique three-dimensional pore structure, whose ultra large cell-like pores (20–50 nm) are interconnected by windows of a smaller opening (9–26 nm).27,29,30 The pore size and window size of MCF could be easily controlled by using organic swelling agent (TMB) and inorganic mineralizing agent (NH4F). However, conventional MCF consists of large, irregular particles of tens of micrometers, the preparation procedure applied in this article results in spherical MCF particles compared to the original recipe for MCF synthesis. This strategy in the synthesis leads to synthesize MCF with spherical morphology compared to conventional MCF with long straight cylinders morphology. Actually emulsion made from oil-to-polymer (TMB/P123) ratios above 0.2, would finally make spherical cells.29 A schematic synthesis of the sorbent applied in this study is represented in Fig. 1 which contains three parts; MCF synthesis, functionalization MCF with (3-chloropropyl) triethoxysilane (MCF-Cl) and reaction of diphenylcarbazide with MCF-Cl to produce (MCF-DPC). MCF synthesis in first part of scheme has several stages containing emulsion formation, the silica source putting on the template and its hydrolysis under acidic condition and the elimination of the organic part with calcination. The pore size, window size, total pore volume and surface area of the spherical MCF sample prepared were shown in Table 1 which characterized by N2 adsorption–desorption isotherm. Furthermore FT-IR analysis of MCF showed a FTIR spectrum typical of silica (Fig. 2a). Spectrum presents the typical Si–O–Si bands of the inorganic framework: symmetric vibration mode around 800 cm−1 and asymmetric stretching vibration around 1080–1100 cm−1.31 The absorption peak at 960 cm−1 is attributed to the bending vibration of the Si–OH bands and the bands at around 1700 and 3400 cm−1 can be assigned to the water stretching modes and bending vibration modes of the free or absorbed water, respectively.32 To functionalize MCF with diphenylcarbazide, hydroxy groups on the synthesized MCF surface was firstly reacted with silanol groups of (3-chloropropyl) triethoxysilane as a linker which is a well-established method to prepare the functionalized silica.33 It must be mentioned that there are hydroxyl groups in pore and also window parts, so the functionalization is occurred in both. Modification with (3-chloropropyl) triethoxysilane, which is performed by condensation of –OH on MCF and ethoxy groups of linker, is presented in the second part of scheme (only the two groups of linker in the pore site as an example is shown). After modification, new bands in FT-IR spectrum at 2850–3000 cm−1 were observed, which associated with C–H vibration (Fig. 2b). Last part of the scheme contains reaction of MCF-Cl with 1,5-diphenylcarbazide by the remove of HCl, which is trapped by TEA in order to increase the reaction yield. The presence of bands at 1250–1550 cm−1 confirmed 1,5-diphenylcarbazide groups' immobilization on MCF-Cl (Fig. 2c).
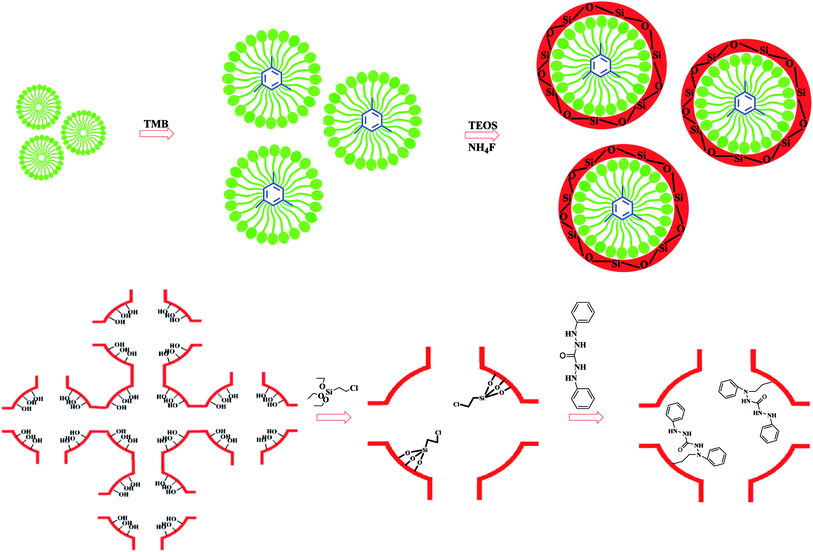 |
| Fig. 1 Schematic diagram of the synthesized MCF-DPC sorbent. | |
Table 1 The textural properties of MCF-DPC sorbent
|
Pore sizea (nm) |
Window sizea (nm) |
Pore volume (cm3 g−1) |
Surface area (m2 g−1) |
Ref. |
The pore size and window size were derived from the adsorption branch and desorption branch of the N2 sorption isotherm, respectively, according to a simplified Broekhoff-de Boer method (BdB-FHH). |
MCF |
29.4 |
17 |
2.1 |
557.3 |
34 |
MCF-DPC |
25 |
16 |
1.95 |
480 |
This work |
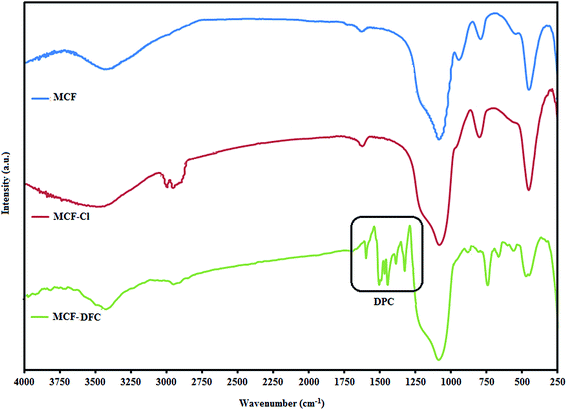 |
| Fig. 2 FT-IR spectra of (a) pure MCF, (b) MCF-Cl and (c) MCF-DPC. | |
The electron microscopy studies were conducted to gain insight in the morphology of MCF-DPC. Fig. 3 shows its image that clearly confirmed a spherical particle of MCF-DPC with a size of about 5 μm in diameter along with some agglomeration of these particles. Furthermore, SEM indicates that the MCF structure after two stages of modification hasn't changed.
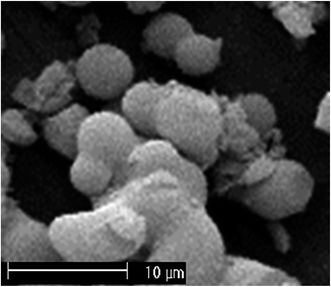 |
| Fig. 3 Scanning electron micrograph of MCF-DPC. | |
The textural properties of MCF-DPC sorbent were derived based on nitrogen adsorption–desorption data using Barrett, Joyner and Halenda (BJH) method to obtain the average pore size and window size. Specific surface area was evaluated using Brunauer, Emmett and Teller (BET) method. Meanwhile, total pore volume (Vpore, cm3 g−1) was calculated as the amount of nitrogen adsorbed at P/P0 = 0.990. The summary of the textural properties of the prepared sorbent are shown in Table 1,34 which are decreased in comparing with MCF from 29.4, 17, 2.1 and 557.3 to 25, 16, 1.95 and 480 in pore size (nm), window size (nm), pore volume (cm3 g−1) and surface area (m2 g−1) respectively, indicating that incorporation of diphenylcarbazide ligand into MCF silica supports with (3-chloropropyl) triethoxysilane as a linker. The decline in both pore and window sizes in MCF-DPC than MCF confirms the functionalization in both parts. As shown in Fig. 4, a typical type IV adsorption isotherm with a H1 hysteresis loop is obtained for MCF-DPC indicating typical mesoporous materials with large pore size and narrow pore size distributions.
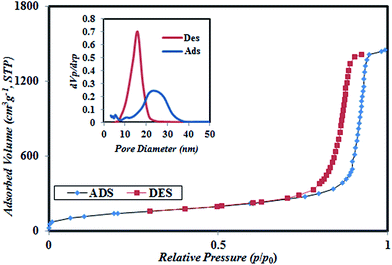 |
| Fig. 4 Nitrogen sorption isotherm and corresponding pore size and window size distributions of MCF-DPC. | |
In order to determine the loading of 1,5-diphenylcarbazide, the N content in this sample was measured by elemental analyzer (CHN). The results showed 1.93% N content which is corresponds to 0.4 mmol g−1 1,5-diphenylcarbazide loaded on the MCF mesoporous.
The thermal stability of MCF-DPC was studied by carrying out TGA-DTA analysis (Fig. 5). A weight loss occurred between 250 °C and 800 °C which is accompanied with exothermic peak at 500 °C in the DTA curve, corresponds to the oxidative decomposition of the organic part associated with 1,5-diphenylcarbazide. Furthermore, according to thermal analysis, the prepared composite is stable up to 200 °C. According to the 11% weight loss in the TGA curve, the amount of ligand introduced into MCF-DPC is 0.39 mmol g−1 which is in good agreement with the loading result of elemental analysis.
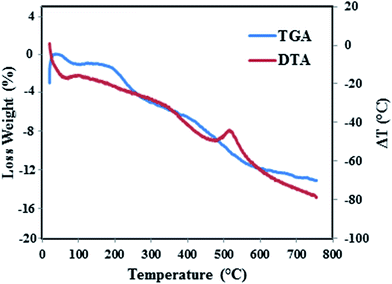 |
| Fig. 5 TGA-DTA analysis of MCF-DPC. | |
Overall, characterization demonstrated that the obtained functionalized MCF possesses excellent connectivity, large cavities, and small and-well tailored windows, which might be eminently suitable for post application like adsorption.
3.2. The effect of pH on retention of target ions by the synthesized mesoporous sorbent
To study the effect of pH on the extraction of target ions, the pH of 25 mL of different sample solutions containing 2 mg L−1 of each target ions was adjusted in the range of 2–10. Interaction between electron pair of –N of ligand on mesoporous sorbent and target ions were affective at natural pHs. The obtained results in Fig. 6 indicate that the target ions could be retained simultaneously on functionalized MCF in the pH of 7.0. The adsorption of cadmium and copper ions by the sorbent increased from pH 2.0 to 7.0 but decreased slightly from pH 8.0 to 10.0. In the low pH, the mesoporous adsorbent was positively charged (the –N group of ligand in low pH is in the form of –NH+) and an electrostatic repulsion occurred between the positively charged analytes and the adsorbent particles. Meanwhile, the observed decrease in retention percentage of cadmium and copper ions on the sorbent at the pH values higher than 7.0, is most probably due to the precipitation of target ions in the hydroxide form, which leads to decreasing the concentration of free cadmium and copper ions in sample. Also, in the presence of modified MCF by DPC in the extraction solution, other parameters can influence in the retention of target ions in different pH (such as different Kf of complexation of ligand with target ions and viscosity of the solution in different pHs). Thus, pH of 7.0 was chosen as the optimum pH for further experiments.
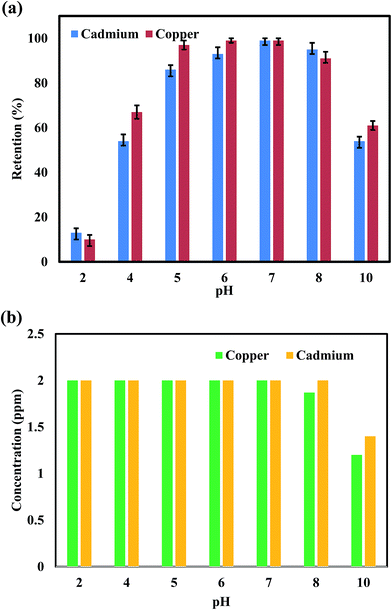 |
| Fig. 6 (a) The effect of solution's pH on the retention of target ions by the mesoporous sorbent. (b) The results for the samples with only copper and cadmium ions (without adsorbent) at different pHs as a control (2 mg L−1 of target ions was analysis without adsorbent in different pHs and the obtained concentration was presented). | |
3.3. Effect of the adsorbent amounts
Compared to conventional sorbents, MCFs offer a significantly higher surface area-to-volume ratio and a short diffusion route, which results in high extraction capacity, rapid extraction dynamics and high extraction efficiencies. Therefore, satisfactory results can be obtained with fewer amounts of these adsorbents. For the optimization of the amount of adsorbent, 5, 10, 15 and 20 mg of the modified MCF were tested. In the present work, by increasing amounts of the modified MCF due to increase in the surface area and accessible sites to the adsorption of the analytes, the extraction efficiency increased. Quantitative extraction of the target ions was achieved using only 10 mg of the modified MCF. At higher amounts of the adsorbent, the extraction efficiency was almost constant. Therefore, 10 mg of the sorbent was chosen for further studies.
3.4. Equilibrium sorption time
In order to investigate the effect of shaking time on the extraction efficiency, extraction experiments were carried out at 2, 5, 10 and 20 min time intervals. According to the results, an equilibration time of about 5 min was required for quantitative extraction of the target ions from solution into mesoporous solid phase. Thus, the mixtures have been shaken for 5 min to reach equilibrium in the subsequent experiments.
3.5. Desorption condition
A series of selected eluent solutions, including HNO3, HCl, HNO3
:
HCl and CH3COOH were used for elution of target ions from the modified MCF. The results show that 1 mol L−1 HCl is a suitable and effective eluent to simultaneous elute the target ions from modified MCF. The effect of eluent volume on the recovery of target ions was also studied (Table 2). Also the results show, quantitative recovery could be obtained with 2.0 mL of 1 mol L−1 HCl. Therefore, 2.0 mL volume of eluent for desorption of target ions was used in the following experiments.
Table 2 The effect of type, concentration, volume and time of the elution step on the extraction recovery of cadmium and copper ions
Eluent |
Concentration (mol L−1) |
Desorption time (min) |
Volume (mL) |
Ra (%) ± Sb |
Cadmium |
Copper |
Recovery. Standard deviation. |
HNO3 |
2 |
10 |
5.0 |
87.0 ± 1.4 |
93.0 ± 1.2 |
HCl |
2 |
10 |
5.0 |
99.0 ± 1.0 |
99.0 ± 1.0 |
CH3COOH |
2 |
10 |
5.0 |
48.0 ± 1.1 |
40.0 ± 1.3 |
HNO3 : HCl |
0.5 : 0.5 |
10 |
5.0 |
89.0 ± 1.3 |
87.0 ± 1.2 |
HCl |
1.5 |
10 |
5.0 |
99.0 ± 1.0 |
99.0 ± 1.0 |
HCl |
1 |
10 |
5.0 |
99.0 ± 1.0 |
99.0 ± 1.0 |
HCl |
0.5 |
10 |
5.0 |
86.0 ± 1.2 |
82.0 ± 1.0 |
HCl |
0.25 |
10 |
5.0 |
65.0 ± 1.3 |
70.0 ± 1.2 |
HCl |
1 |
10 |
4.0 |
99.0 ± 1.0 |
99.0 ± 1.0 |
HCl |
1 |
10 |
3.0 |
99.0 ± 1.0 |
99.0 ± 1.0 |
HCl |
1 |
10 |
2.0 |
99.0 ± 1.0 |
99.0 ± 1.0 |
HCl |
1 |
10 |
1.5 |
90.0 ± 1.2 |
75.0 ± 1.3 |
HCl |
1 |
5 |
2.0 |
99.0 ± 1.0 |
99.0 ± 1.0 |
HCl |
1 |
3 |
2.0 |
99.0 ± 1.0 |
99.0 ± 1.0 |
HCl |
1 |
2 |
2.0 |
99.0 ± 1.0 |
99.0 ± 1.0 |
Desorption times were evaluated in the range of 2–10 min. The results showed that the time of 2 min is sufficient for simultaneous desorption of the target ions by 2.0 mL of the 1 mol L−1 HCl.
3.6. Effect of sample volume
Due to the low concentrations of trace metals in real samples, by using samples with large volumes, the trace metals in these volumes should be taken into smaller volumes for high preconcentration factor. Hence, the maximum sample volume was optimized by the investigation of the recovery of target ions in various synthetic samples, volumes in the range of 25–600 mL containing 0.01 mg of targets were used to study. In the optimization of the sample volume, 10 mg of the sorbent was used. The recovery of targets from different volumes of aqueous solutions were shown in Fig. 7. The recovery was found to be stable until 300 mL and was chosen as the largest sample volume to this work.
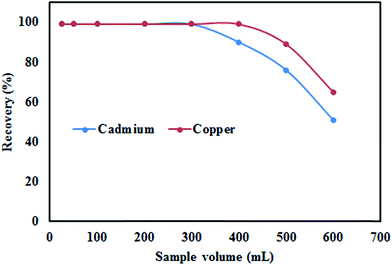 |
| Fig. 7 The effect of sample volume on the recovery of target ions by the mesoporous sorbent. | |
3.7. Effect of potentially interfering ions
Because of the presence of other elements in real samples, the determination and preconcentration of target ions is difficult. So, the effects of common coexisting cations and anions on the adsorption of the target ions on the modified MCF were investigated. In these experiments, 150 mL of solution containing 0.003 mg target ions were added to interfering cations and anions and treated according to the recommended procedure. The results in Table 3 show that the vast majority of transition, alkaline, and earth alkaline metals do not interfere at environmentally relevant concentrations. This is due to the low capacity or rates of adsorption for interfering ions under optimum condition. Thus, these results confirm that the procedure using modified MCF is independent of matrix interferences.
Table 3 The effect of potentially interfering ions on the extraction recovery of cadmium and copper by the synthesized mesoporous sorbent
Foreign ion |
Tolerable concentration ratio X/cadmium and copper ions |
Ra (%) ± Sb |
Cadmium(II) |
Copper(II) |
Recovery. Standard deviation. |
K+ |
10 000 |
99.0 ± 2.0 |
99.0 ± 2.0 |
Na+ |
10 000 |
99.0 ± 1.0 |
99.0 ± 2.0 |
Ag+ |
1000 |
97.0 ± 1.0 |
96.0 ± 1.0 |
Cs+ |
1000 |
97.1 ± 1.2 |
96.5 ± 1.1 |
Ca2+ |
1000 |
97.0 ± 1.1 |
96.5 ± 0.9 |
Mg2+ |
1000 |
96.2 ± 1.4 |
98.5 ± 0.6 |
Al3+ |
1000 |
97.5 ± 1.1 |
97.2 ± 1.1 |
Fe2+ |
900 |
97.1 ± 1.4 |
98.5 ± 1.0 |
Ni2+ |
600 |
96.5 ± 1.5 |
97.1 ± 0.9 |
Mn2+ |
800 |
97.2 ± 1.2 |
97.5 ± 0.5 |
Pb2+ |
600 |
97.4 ± 0.8 |
98.2 ± 1.4 |
Cr3+ |
700 |
96.5 ± 1.1 |
97.2 ± 1.4 |
CO32− |
1000 |
98.4 ± 0.9 |
98.0 ± 1.2 |
SO42− |
1000 |
97.0 ± 1.4 |
97.0 ± 1.5 |
CrO42− |
800 |
97.0 ± 1.2 |
97.0 ± 1.4 |
PO43− |
800 |
98.0 ± 1.1 |
97.0 ± 1.2 |
Cl− |
3000 |
96.0 ± 1.2 |
97.0 ± 1.5 |
3.8. Maximum adsorption capacity
In order to determine how much sorbent was required to quantitatively remove a specific amount of a metal ion from the solution, the capacity of the sorbent was calculated. To evaluate this factor, 25 mL of a solution containing 2 mg target ions underwent the extraction procedure, and the maximum capacity was calculated (with 10 mg of the synthesized sorbent). The obtained capacities of modified MCF were found to be 190 and 160 mg g−1 for cadmium and copper ions, respectively. In order to evaluate the maximum adsorption capacity, the difference between concentration of the solution before and the concentration of the solution after solid phase extraction procedure by the sorbent was calculated.
3.9. Langmuir isotherm models
In this work, Langmuir isotherms were used to measure the adsorption of copper and cadmium ions onto DPC-MCF surface. The Langmuir adsorption model is a theoretical equation and applicable to homogeneous binding sites and assumes that the molecules are adsorbed at a fixed number of well-defined sites, each of which can only hold one molecule. These sites are also assumed to be energetically equivalent and distant to each other; therefore, there are no interactions between molecules adsorbed on adjacent sites. The amount of Cu(II) and Cd(II) bound by the modified mesoporous sorbent was calculated according to the following formula:
where Q is the amount of adsorbed Cu(II) and Cd(II) (mg g−1); C0 and C are the initial and final concentrations of Cu(II) and Cd(II) (mg L−1), respectively; V is the volume of mixture (mL); and W is the amount of sorbent (g).
The Langmuir adsorption isotherm is expressed by following equation.
where
Q is the amount of adsorbed Cu(
II) and Cd(
II) ions on the MCF-DPC at equilibrium (mg g
−1),
Ce is the equilibrium concentration of Cu(
II) and Cd(
II) ions in solution (mg L
−1) and
Qmax and
b are Langmuir constants related to the maximum adsorption capacity and energy of adsorption, respectively. The Langmuir equation can be linearized in a normal form for the determination of Langmuir constants:
Langmuir equations for copper and cadmium at pH 7 for 25 mL of sample volume and 10 mg of the sorbent are as follows:
Y = 0.0078X + 0.0053 (R2 = 0.98) Langmuir equations for cadmium ions |
Y = 0.0082X + 0.0062 (R2 = 0.99) Langmuir equations for copper ions |
According to the relations above, adsorption capacities for Cd(II) and Cu(II) were calculated to be 188.7 mg g−1 and 161.2 mg g−1, respectively.
Furthermore, the essential characteristic of the Langmuir isotherm can be expressed in terms of a dimensionless equilibrium parameter, the separation factor (RL), which used in the following equation, RL = 1/(1 + bC0). The value of parameter RL indicates the nature of the adsorption process which irreversible (RL = 0), favorable (0 < RL < 1), linear (RL = 1), or unfavorable (RL > 1). The value of RL for the present system comes out to be 0.018 and 0.02 for cadmium and copper, respectively, communicating the favorable adsorption of the target ions onto DPC-MCF.
3.10. Freundlich isotherm model
The Freundlich isotherm model is the earliest known relationship describing the sorption process. The model applies to adsorption on heterogeneous surfaces with interaction between adsorbed molecules and the application of the Freundlich equation also suggests that sorption energy exponentially decreases on completion of the sorptional centers of an adsorbent. This isotherm is an empirical equation can be employed to describe heterogeneous systems and is expressed as follow:
where KF is the Freundlich constant (L g−1) related to the bonding energy. KF can be defined as the adsorption or distribution coefficient and represents the quantity of ions adsorbed onto adsorbent for unit equilibrium concentration. 1/nF is the heterogeneity factor and nF is a measure of the deviation from linearity of adsorption. Its value indicates the degree of non-linearity between solution concentration and adsorption as follows: if the value of nF is equal to unity, the adsorption is linear; if the value is below to unity, this implies that adsorption process is chemical; if the value is above to unity adsorption is a favorable physical process. qe = KFCe1/nF can be linearized in the logarithmic form and the Freundlich constants can be determined:
log qe = log KF + 1/nF log Ce |
The plot of log(qe) versus log(Ce) was employed to generate the intercept value of KF and the slope of 1/nF (Table 4). The correlation coefficients obtained from Freundlich model is comparable to that obtained from Langmuir model linear form. The values of Freundlich constant 1/nF is between 0 and 1.0 which also indicated that adsorption of cadmium (0.86) and copper (0.7) ions under the studied condition are suitable.
Table 4 The obtained data from Freundlich isotherm model
Ion |
Freundlich model |
R2 |
KF |
nF |
Cadmium |
Y = 0.86X + 1.92 |
0.97 |
83.2 |
1.16 |
Copper |
Y = 0.71X + 1.77 |
0.98 |
58.9 |
1.4 |
A comparison of the two isotherms based on the linear regression coefficient (R2) values showed that the sorption of target ions on DPC-MCF nearly similar results (R2) for both Langmuir and Freundlich isotherm models, under the concentration range studied.
3.11. Analytical performance
Under the optimized conditions, calibration curves were sketched for the determination of cadmium and copper ions according to the optimum extraction procedure. Linearity was maintained 0.5–200 μg L−1 for copper and 0.1–30 μg L−1 for cadmium in initial solution (150 mL of sample). The correlation of determination (R2) was 0.99 for copper and 0.99 for cadmium ions. The limit of detection, which is defined as CLOD = 3Sb/m, where Sb is the standard deviation of eight replicate blank signals and m is the slope of the calibration curve after preconcentration, for a sample volume of 150 mL, was found to be 0.1 μg L−1 for copper and 0.04 μg L−1 for cadmium ions, respectively. The relative standard deviations for eight separate experiments for determination of 7.5 μg of cadmium and copper ions in 150 mL of water was 2.5 and 3.1, respectively. Regression equation, dynamic linear range (DLR), correlation of determination (R2), preconcentration factor (PF), limit of detection (LOD) and relative standard deviation (RSD) for cadmium and copper were calculated under optimized conditions and summarized in Table 5.
Table 5 Regression equation, dynamic linear range (DLR), correlation of determination (R2), preconcentration factor (PF), limit of detection (LOD) and relative standard deviation (RSD) for copper and copper were calculated under optimized conditions
Analyte |
Regression equation |
r2 |
LOD (ng mL−1) |
DLR (ng mL−1) |
PF |
Recoveryb (%) |
RSDb (%) |
MACa (mg g−1) |
Maximum sorption capacity. Recovery and RSD is calculated for 50 μg L−1 of copper and cadmium ions. |
Copper ion |
Y = 8.53X (mg L−1) + 0.001 |
0.99 |
0.1 |
0.5–200 |
75 |
>98 |
3.1 |
160 |
Cadmium ion |
Y = 51.75X (mg L−1) + 0.0004 |
0.99 |
0.04 |
0.01–20 |
75 |
>98 |
2.5 |
190 |
Since 150 mL of the solution was preconcentrated to 2.0 mL, the approximately preconcentration factor of 75 was obtained.
3.12. Real sample analysis
Finally, this method was performed on real samples to check its accuracy. Since the amounts of cadmium and copper ions in real samples are too low, some amount of the target ions was also spiked in these samples. To test the reliability of the method for extraction and determination of the target ions in the environmental water samples were applied. The results demonstrate that quantitative recoveries of the target ions for modified MCF with diphenylcarbazide are achievable. The results are listed in Table 6.
Table 6 Analysis of copper and cadmium ion in different food samples
Sample |
Ion |
Added (μg L−1) |
Founded (μg L−1) |
RR (%) |
RSD (%) |
Tap water |
Copper |
— |
10.2 |
— |
2.1 |
5.0 |
15.2 |
100.0 |
2.8 |
50.0 |
59.5 |
98.6 |
2.6 |
Cadmium |
— |
— |
— |
— |
5.0 |
5.1 |
102.0 |
2.9 |
50.0 |
49.8 |
99.6 |
2.9 |
Caspian sea water |
Copper |
— |
19.8 |
— |
2.6 |
5.0 |
24.7 |
98.0 |
3.1 |
50.0 |
69.9 |
100.2 |
2.8 |
Cadmium |
— |
7.1 |
— |
2.8 |
5.0 |
12.0 |
98.0 |
3.1 |
50.0 |
56.9 |
99.6 |
2.7 |
Persian Gulf water |
Copper |
— |
18.3 |
— |
1.9 |
5.0 |
23.4 |
102.0 |
2.7 |
50.0 |
68.1 |
99.6 |
2.4 |
Cadmium |
— |
6.6 |
— |
2.5 |
5.0 |
11.7 |
102.0 |
2.9 |
50.0 |
56.5 |
99.8 |
2.2 |
Lake water |
Copper |
— |
15.2 |
— |
2.1 |
5.0 |
20.3 |
102.0 |
2.8 |
50.0 |
64.9 |
99.4 |
3.1 |
Cadmium |
— |
2.3 |
— |
2.7 |
5.0 |
7.3 |
100.0 |
2.5 |
50.0 |
52.1 |
99.6 |
2.5 |
The concentration of copper and cadmium ions obtained by modified MCF was compared to the standard reference material. For this reason, the concentration of the copper and cadmium ions was determined at optimum conditions in standard reference material (Soil (NCS DC 73323)). As it can be seen in Table 1S (ESI†), good correlation was achieved between estimated content by the present method and reference materials. Therefore, modified MCF with diphenylcarbazide can be used as a reliable solid phase for extraction and determination of copper and cadmium ions in real samples.
4. Conclusions
S-MCF microparticles with a spherical morphology and a narrow particle size distribution were prepared by a simple modification of the conventional MCF synthesis. The pore sizes and surface areas of S-MCF particles could be adjusted without affecting the particle morphology. The S-MCF particles were functionalized with diphenylcarbazide groups, and applied as a new class mesoporous sorbent for simultaneous separation and trace detection of copper and cadmium ions in environmental water samples. Their high surface areas gave rise to very good retention ability, as illustrated in the separation of cadmium and copper ions. The highly interconnected porous structure and ultra large pore size allowed MCF to be used as a sorbent for rapid extraction of ions and molecules. In this work, for the first time, modified MCF with diphenylcarbazide was utilized as an adsorbent for the simultaneous separation of ultra-trace amounts of cadmium and copper ions. This method is simple, rapid and reliable and found as a selective and sensitive method for the determination of trace levels of cadmium and copper ions. The convenient data was found for adsorption capacity, detection limit and pre-concentration factor in the determination of the target ions and confirmed that this method using modified MCF with diphenylcarbazide has a high potential for extraction of metal ions. As a result, the LOD, pre-concentration factor and maximum adsorption capacity of this method is better than some of the previously reported pre-concentration methods (Table 7).35–39
Table 7 Comparison of the proposed method with previously published work related to the extraction and determination of cadmium and copper ions
Instrument |
Method |
Elements |
LOD (ng mL−1) |
PFa |
MACb |
Ref. |
Preconcentration factor. Maximum adsorption capacity. |
ICP-OES |
AEDHB-SG sorbent |
Cadmium |
0.012 |
100 |
0.40 mmol g−1 |
35 |
Copper |
0.098 |
100 |
0.56 mmol g−1 |
FAAS |
UA-IL-DLLME |
Cadmium |
— |
— |
— |
36 |
Copper |
0.17 |
100 |
— |
ICP-OES |
Morin-magnetic nanoparticle |
Cadmium |
— |
— |
— |
37 |
Copper |
0.9 |
28 |
— |
FAAS |
Chelating resin |
Cadmium |
4.2 |
100 |
— |
38 |
Copper |
— |
— |
— |
FAAS |
Modified silica gel |
Cadmium |
1.1 |
27 |
0.067 mmol g−1 |
39 |
Copper |
1.0 |
27 |
0.30 mmol g−1 |
FAAS |
Diphenylcarbazide-MCF |
Cadmium |
0.04 |
75 |
190 mg g−1 |
This work |
Copper |
0.1 |
75 |
160 mg g−1 |
References
- G. Somer, A. N. Unlu, S. Kalayci and F. Sahin, Turk. J. Chem., 2006, 30, 419–427 CAS.
- A. Bagheri, M. Taghizadeh, M. Behbahani, A. A. Asgharinezhad, M. Salarian, A. Dehghani, H. Ebrahimzadeh and M. M. Amini, Talanta, 2012, 99, 132–139 CrossRef CAS PubMed.
- A. Bagheri, M. Behbahani, M. M. Amini, O. Sadeghi, M. Taghizade, L. Baghayi and M. Salarian, Talanta, 2012, 89, 455–461 CrossRef CAS PubMed.
- N. Jalbani and M. Soylak, Talanta, 2015, 131, 361–365 CrossRef CAS PubMed.
- M. Behbahani, Y. Bide, M. Salarian, M. Niknezhad, S. Bagheri, A. Bagheri and M. R. Nabid, Food Chem., 2014, 158, 14–19 CrossRef CAS PubMed.
- F. Aydin, E. Yilmaz and M. Soylak, RSC Adv., 2015, 5, 40422–40428 RSC.
- Z. Wei, S. Sandron, A. T. Townsend, P. N. Nesterenko and B. Paull, Talanta, 2015, 135, 155–162 CrossRef CAS PubMed.
- Z. Wang, P. Mun Lee and E. Liu, Thin Solid Films, 2013, 544, 341–347 CrossRef CAS PubMed.
- M. Behbahani, P. Ghareh Hassanlou, M. M. Amini, F. Omidi, A. Esrafili, M. Farzadkia and A. Bagheri, Food Chem., 2015, 187, 82–88 CrossRef CAS PubMed.
- E. Yilmaz and M. Soylak, Talanta, 2014, 126, 191–195 CrossRef CAS PubMed.
- M. R. Nabid, R. Sedghi, M. Behbahani, B. Arvan, M. M. Heravi and H. Abdi Oskooie, J. Mol. Recognit., 2014, 27, 421–428 CrossRef CAS PubMed.
- C. Cui, M. He, B. Chen and B. Hu, Talanta, 2013, 116, 1040–1046 CrossRef CAS PubMed.
- S. Su, B. Chen, M. He and B. Hu, Talanta, 2014, 123, 1–9 CrossRef CAS PubMed.
- C. Kulek de Andrade, V. Egéa dos Anjos, M. Lurdes Felsner, Y. Reyes Torres and S. Pércio Quináia, Food Chem., 2014, 146, 166–173 CrossRef PubMed.
- M. Kalate Bojdi, M. H. Mashhadizadeh, M. Behbahani, A. Farahani, S. S. Hosseini Davarani and A. Bagheri, Electrochim. Acta, 2014, 136, 59–65 CrossRef PubMed.
- A. Bahrami, A. Besharati-Seidani, A. Abbaspour and M. Shamsipur, Electrochim. Acta, 2014, 118, 92–99 CrossRef CAS PubMed.
- M. Hossien-poor-Zaryabi, M. Chamsaz, T. Heidari, M. H. Arbab Zavar, M. Behbahani and M. Salarian, Food Analytical Methods, 2014, 7, 352–359 CrossRef.
- M. H. Karbasi, B. Jahanparast, M. Shamsipur and J. Hassan, J. Hazard. Mater., 2009, 170, 151–155 CrossRef CAS PubMed.
- Z. A. Alothman, E. Yilmaz, M. Habila and M. Soylak, Ecotoxicol. Environ. Saf., 2015, 112, 74–79 CrossRef CAS PubMed.
- H. R. Fouladian and M. Behbahani, Food Analytical Methods, 2015, 8, 982–993 CrossRef.
- B. Feist and B. Mikula, Food Chem., 2014, 147, 225–229 CrossRef CAS PubMed.
- S. Aslam Arain, T. G. Kazi, H. I. Afridi, A. R. Abbasi, A. H. Panhwar, Naeemullah, B. Shanker and M. B. Arain, Spectrochim. Acta, Part A, 2014, 133, 651–656 CrossRef PubMed.
- G. Hellé, C. Mariet and G. Cote, Talanta, 2015, 139, 123–131 CrossRef PubMed.
- C. F. Poole, A. D. Gunatilleka and R. Sethuraman, J. Chromatogr. A, 2000, 885, 17–39 CrossRef CAS.
- Y. Han, S. Seong Lee and J. Y. Ying, J. Chromatogr. A, 2010, 1217, 4337–4343 CrossRef CAS PubMed.
- Q. Li, Z. Wu, D. Feng, B. Tu and D. Zhao, J. Phys. Chem. C, 2010, 114, 5012–5019 CAS.
- P. Schmidt-Winkel, W. W. Lukens, J. Dongyuan Zhao, P. Yang, B. F. Chmelka and G. D. Stucky, J. Am. Chem. Soc., 1999, 121, 254–255 CrossRef CAS.
- M. Behbahani, M. Salarian, M. M. Amini, O. Sadeghi, A. Bagheri and S. Bagheri, Food Analytical Methods, 2013, 6, 1320–1329 CrossRef.
- J. S. Lettow, Y. J. Han, P. Schmidt-Winkel, P. Yang, D. Zhao, G. D. Stucky and J. Y. Ying, Langmuir, 2000, 16, 8291–8295 CrossRef CAS.
- W. W. Lukens Jr, P. Yang and G. D. Stucky, Chem. Mater., 2001, 13, 28–34 CrossRef.
- P. Innocenzi, J. Non-Cryst. Solids, 2003, 316, 309–319 CrossRef CAS.
- M. Behbahani, A. Ali Akbari, M. M. Amini and A. Bagheri, Anal. Methods, 2014, 6, 8785–8792 RSC.
- K. Barnes, J. Liang, S. D. Worley, J. Lee, R. M. Broughton and T. S. Huang, J. Appl. Polym. Sci., 2007, 105, 2306–2613 CrossRef CAS PubMed.
- Y. Han, S. Seong Lee and J. Y. Ying, Chem. Mater., 2007, 19, 2292–2298 CrossRef CAS.
- E. Durduran, H. Altundag, M. Imamoglu, S. Zeki Yıldız and M. Tuzen, J. Ind. Eng. Chem., 2015, 27, 245–250 CrossRef CAS PubMed.
- N. Jalbani and M. Soylak, Food Chem., 2015, 167, 433–437 CrossRef CAS PubMed.
- M. Khajeh, Biol. Trace Elem. Res., 2010, 135, 355–363 CrossRef CAS PubMed.
- I. Narin, Y. Surme, E. Bercin and M. Soylak, J. Hazard. Mater., 2007, 145, 113–119 CrossRef CAS PubMed.
- W. Ngeontae, W. Aeungmaitrepirom and T. Tuntulani, Talanta, 2007, 71, 1075–1082 CrossRef CAS PubMed.
Footnote |
† Electronic supplementary information (ESI) available. See DOI: 10.1039/c5ra10240e |
|
This journal is © The Royal Society of Chemistry 2015 |