DOI:
10.1039/C5RA09931E
(Paper)
RSC Adv., 2015,
5, 73184-73193
Ligand-conjugated pH-sensitive polymeric micelles for the targeted delivery of gefitinib in lung cancers
Received
26th May 2015
, Accepted 3rd August 2015
First published on 3rd August 2015
Abstract
The aim of the present study was to investigate the tumor targeting potential of a mannose-conjugated pH-sensitive nanosystem for the effective delivery of gefitinib (Gnb) to lung cancers. We have successfully demonstrated the potential of mannose-tagged nanomicelles as an efficient vector to transport the anticancer drug. The micelles had nanosized particles with excellent dispersity index. The nanomicelles exhibited a pH-responsive nature with enhanced drug release in acidic pH conditions. Fluorescence and flow cytometer analysis showed a superior cellular uptake for the mannose-tagged nanomicelles. Confocal laser scanning microscopy study revealed a receptor-mediated cellular internalization process. The M-NP-Gnb showed a superior anticancer effect in A549 cancer cells and remarkably inhibited cancer cell proliferation. Furthermore, M-NP-Gnb significantly increased the proportion of cells in the apoptosis and necrosis region. Importantly, the half-life of Gnb encapsulated in nanomicelles increased by about 5-fold compared to that of free Gnb. The augmented half-life clearly indicates the maximum residence time of the drug in the systemic circulation. Consistently, M-NP-Gnb showed a 7-fold higher accumulation of drug in tumor tissues compared to that of free Gnb. Overall, PLGA/His-based nanomicelles could be a promising delivery system to increase the therapeutic efficiency of Gnb in lung cancers.
Introduction
Lung cancer is one of the leading causes of cancer-related death worldwide. The death rate due to lung cancer far exceeds the combined death rate of prostate, colon, and breast cancers.1,2 The 5 year survival rate of lung cancer patients is below 15% despite the advancements in technology. Lung cancers can be divided into non-small cell lung cancer and small cell lung cancer.3 Non-small cell lung cancer comprises approximately 75–80% of all respiratory lung cancers. The incidence and mortality of lung cancer are increasing every year in China. At present, surgery, radiotherapy and chemotherapy constitute the main treatment modes for lung cancers.4,5
In this regard, tyrosine kinase inhibitors are shown to possess excellent antitumor activity against lung cancer cells. Gefitinib (Gnb), a tyrosine kinase inhibitor, has high affinity towards epithelial growth factor receptor (EGFR) which is normally expressed in lung cancers.6,7 Gnb can effectively kill lung cancer cells via promoting metastasis, angiogenesis, and apoptosis in tumor cells. The main mechanism behind the anticancer effect of Gnb is the blocking of signal transmission by competitive binding of Mg-ATP situated on the catalytic domain of EGFR-TK.8,9 This will inhibit the activation of mitogen-activated protein kinase and induce the apoptosis of cancer cells. Despite its potential clinical application, Gnb is widely distributed in the body, resulting in severe drug-related toxicity. Moreover, limited presence of the drug in cancer cells further complicates the chemotherapy treatment.10 Therefore, new strategies have to be developed to increase the chemotherapeutic efficacy of Gnb in lung cancers.
Nanotechnology has opened up new horizons in cancer drug delivery. Recent studies have highlighted the role of nanotechnology in cancer therapy.11 Drug-loaded nanocarriers have been shown to increase the blood circulation of anticancer small molecules, sustain drug release in physiological conditions, and increase drug accumulation in the tumor region.12 When antitumor drugs are loaded in nanoparticles with a size of 20–200 nm, their biodistribution in heart and lung is largely decreased.13 In this study, therefore, a novel pH-sensitive delivery system has been designed that will increase the drug concentration in cancer tissues while reducing its presence in normal tissues. PLGA is one of the most common polymers used as a carrier for drug delivery applications due to its inherent biodegradability and low toxicity. Therefore, PLGA-PEG was selected in order to form a stable hydrophobic core with PEG forming the outer shell which protects the carrier from immediate systemic clearance. Polyhistidine was synthesized and utilized in micelle formation in order to bring a pH-responsiveness to the carrier system.14–16 The nanocarrier thus formed is able to extravasate solid tumors into the tumor tissues via an enhanced permeability and retention effect. However, passive targeting of cancer cells is not found to be effective and warranted an alternative therapeutic approach.17,18 In this context, glycotargeting involves the interaction of lectin receptors with carbohydrate ligands. It has been reported that mannose–cytostatics complexes exhibit selective uptake in cancer cells.19 The preferential uptake was attributed to the receptor-mediated internalization by lectin receptors at the tumor surface. In the present study, therefore, a polysaccharide-mannose tagged PLGA-based nanocarrier system has been developed to target lung cancers.20
The main objective of the present study was to explore the prospects of polysaccharide-tagged pH-sensitive nanomicelles (PLGA/Histidine) for the selective delivery of Gnb in lung cancers. In this study, we firstly developed PLGA/pHistidine-based nanomicelles which were then conjugated with mannose as a cancer targeting moiety. The pH-sensitive nature of the nanocarrier was assessed by means of particle size analysis and drug release kinetics study. In vitro cellular uptake of targeted and non-targeted NP was analyzed by fluorescence and flow cytometry (fluorescence activated cell sorting (FACS)) and observed by confocal laser scanning microscopy (CLSM). The methylthiazoltetrazolium (MTT) assay was employed to examine the in vitro cytotoxicity of the free drug as well as drug-loaded micelles. Annexin V/propidium iodide (PI)-based apoptosis analysis was performed in A549 lung cancer cells. Finally, systemic performances of free drug and drug-loaded micelles were investigated in experimental rats.
Results and discussion
Lung cancer is one of the leading causes of cancer-related death worldwide. In this regard, Gnb, a tyrosine kinase inhibitor, can effectively kill lung cancer cells via promoting metastasis, angiogenesis, and apoptosis in tumor cells. Therefore, in the present study, we have developed PLGA/pHistidine-based nanomicelles to target Gnb to lung cancer cells (Fig. 1).
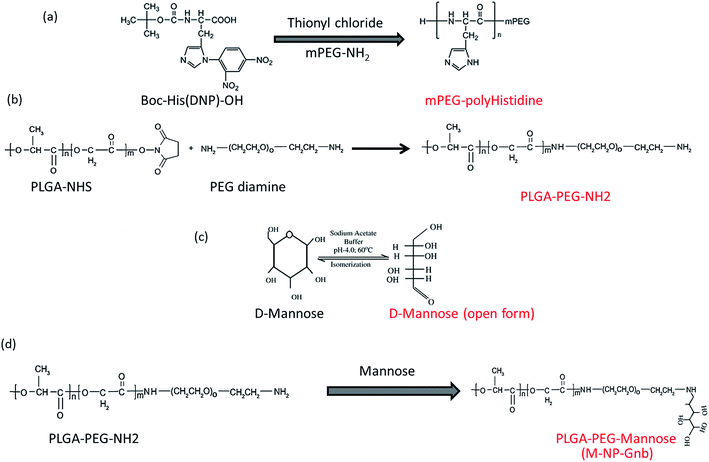 |
| Fig. 1 Synthesis of PLGA-PEG-NH2, PEG-polyhistidine, and PLGA-PEG-mannose. | |
Preparation of M-NP-Gnb nanomicelles
The pHis-PEG was synthesized by a ring opening polymerization process. Poly(L-histidine) was conjugated with PEG via an amide bond. In the case of PLGA-PEG-NH2, PEG-diamine was reacted with PLGA-NHS and the resulting product was reacted with mannose. The mannosylation was performed by ring opening of mannose and subsequent reaction of its aldehyde group with the free amine functional group of PLGA-PEG-NH2 of the nanoparticle surface. This led to the formation of a Schiff base (–N–CH–). The main objective of the present study was to explore the potential of the polysaccharide-tagged pH-sensitive nanomicelles (PLGA/Histidine) for the selective delivery of Gnb in lung cancers. PLGA-PEG and histidine-PEG were synthesized in order to formulate mixed micelles wherein PLGA forms the inner hydrophobic core and PEG forms the outer shell. The mannose was conjugated to PLGA-PEG polymer block and present in the outer nanoparticle surface. The presence of mannose on the outer nanoparticle surface will increase the targeting and accumulating efficiency of the chemotherapeutic drug.
Characterization of M-NP-Gnb
The particle size and zeta potential of all the nanoparticles were evaluated by the dynamic light scattering method. The average particle size of NP-Gnb was about 120 nm and the particle size increased after mannose conjugation (168 nm) (Fig. 2a). The increase in the size of nanoparticles was due to the occurrence of mannose conjugation to the outer surface. Nevertheless, a particle size of <200 nm would be very suitable for tumor targeting applications.21 The micelles were negatively charged with a potential of −18.5 mV. The negative surface charge of nanoparticles would increase their physiological stability in the blood circulation. During in vivo applications, such a negative surface charge would reduce the undesirable clearance by the reticuloendothelial system which would increase accumulation in tumor tissues. The particle morphology was observed using transmission electron microscopy (TEM) imaging. TEM showed clear spherical-shaped particles of M-NP-Gnb which are uniformly dispersed on a carbon-coated copper grid (Fig. 2b). The particle size observed from TEM imaging was consistent with the dynamic light scattering particle size.
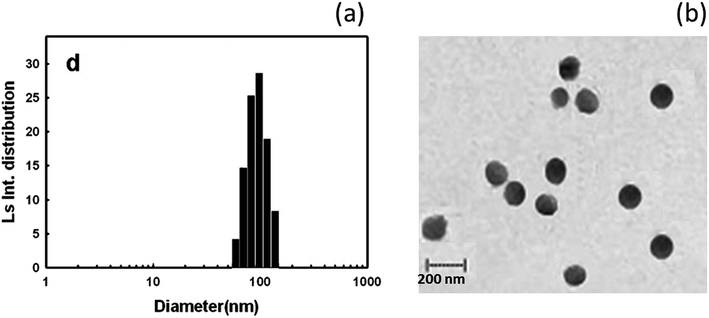 |
| Fig. 2 Characterization of drug-loaded nanoparticles. (a) Particle size distribution of M-NP-Gnb. (b) TEM image of M-NP-Gnb. | |
pH sensitivity of M-NP-Gnb micelles
The pH-sensitive nature of M-NP-Gnb was investigated by means of size, size distribution, and zeta potential analysis. The histidine present in the micellar structure is mainly responsible for the pH-responsiveness of the system. As shown in Table 1, zeta potential increased with a decrease in the pH of suspension medium indicating the effective protonation of the histidine residue. Especially, micelles were stable at pH 7.4; however, when the pH decreased to 5.5, abundant protonation of histidine resulted in a low zeta potential of −3 mV. Consistently, the particle size of the micelles increased due to the disassembly and aggregation of the multiple polymers.
Table 1 pH-sensitive nature of M-NP-Gnb by dynamic light scattering analysis
pH |
Size (nm) |
PDI |
Zeta potential (mV) |
8.5 |
146.8 ± 2.16 |
0.118 ± 0.018 |
−24.5 ± 1.65 |
7.4 |
165.7 ± 1.68 |
0.157 ± 0.025 |
−18.2 ± 1.12 |
6.5 |
210.2 ± 2.61 |
0.265 ± 0.034 |
−8.32 ± 1.89 |
5.5 |
325.5 ± 4.65 |
0.398 ± 0.042 |
−3.26 ± 1.46 |
Drug loading and in vitro drug release study
Knowledge of drug entrapment efficiency is very important for drug delivery applications. The M-NP-Gnb exhibited a high entrapment efficiency of >95% with a high drug loading of ∼24.7%. The pH-sensitive nature of NP-Gnb and M-NP-Gnb was evaluated from release kinetics. It is expected that the presence of histidine in the mixed micelles would provide the nanocarrier system with a pH-responsive nature. The pH-responsiveness would increase the swelling and dissociation of the micellar system resulting in the release of drug. It can be seen from Fig. 3 that both NP-Gnb and M-NP-Gnb exhibited a typical pH-sensitive release pattern. Approximately 60% of drug was released in pH 5.5 conditions compared to 35% of drug released in pH 7.4 conditions. The release trend continued up to 48 h where almost 95% of drug was released in acidic pH and 50% of drug was released in pH 7.4 conditions. It should be noted that no initial burst release phenomenon was observed suggesting that all of the drug was tightly packed in the hydrophobic core of micelles. Although the rate of drug release from NP-Gnb and M-NP-Gnb was very similar, a slight decrease in the release rate from M-NP-Gnb was ascribed to the presence of the protective coat of mannose on the micelle surface, which impedes drug release.22 Overall, enhanced release rate at lower pH would be beneficial for anticancer application, since tumor tissue is characterized by an acidic microenvironment.
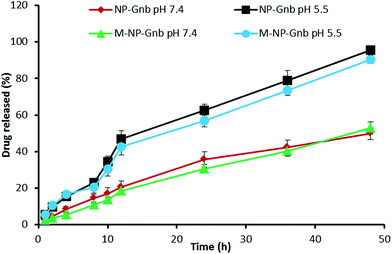 |
| Fig. 3 Release profile of NP-Gnb and M-NP-Gnb in phosphate buffered saline (pH 7.4) and acetate buffered saline (pH 5.5). The release study was performed at 37 °C (containing 0.1% Tween 80). | |
Cellular uptake efficiency
The cellular uptake efficiency as a function of targeting agent has been studied with CHO cells (control) and A549 lung cancer cells. For this purpose, rhodamine-b was loaded in the nanomicellar formulations to observe the fluorescence intensity or conduct fluorescence measurements. The cellular uptake of targeted and non-targeted nanoparticles was studied in these two cell lines by means of flow cytometry (Fig. 4a). In the case of CHO cells (which do not express lectin receptors), cellular uptake of NPs and M-NP-Gnb was not markedly different. In the case of A549 cells, however, a marked difference was observed between targeted and non-targeted nanoparticles. It could be clearly seen that M-NP-Gnb had significantly higher cellular uptake than NP after 2 h of incubation. The mannose-tagged micelles could enter the cells more quickly than the non-targeted NPs. Mannosylated NPs could not be taken up by CHO cells via receptor-mediated endocytosis because mannose receptors are not expressed on their membrane. Having shown the specificity of mannosylated NPs towards lectin receptor expression cancer cells, we carried out all our experiments with A549 cells.
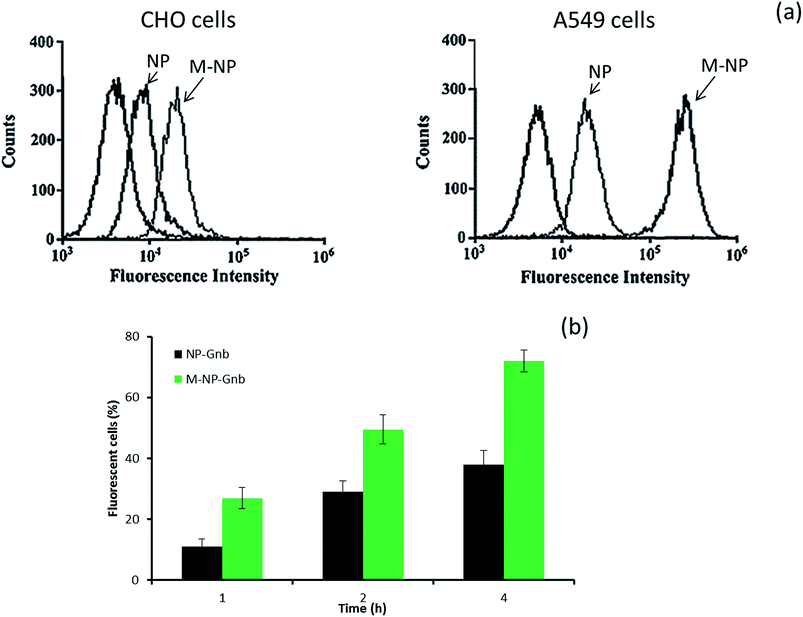 |
| Fig. 4 Cellular uptake study of A549 cells. (a) Fluorescence analysis of NP-Gnb and M-NP-Gnb in cancer cells. The cellular uptake analysis was performed at various time points. (b) Flow cytometer analysis of NP-Gnb and M-NP-Gnb. | |
The cellular uptake was further studied by fluorescence experiments. The cancer cells were exposed to NP-Gnb and M-NP-Gnb and incubated for different time periods up to 4 h (Fig. 4b). The results clearly revealed a time-dependent cellular uptake. Importantly, mannose-tagged formulations showed a significant increase in the cellular uptake compared to the non-targeted nanosystem. For example, at the end of 4 h, M-NPs showed ∼70% fluorescence intensity compared to ∼38% fluorescence intensity of non-targeted NPs. The high fluorescence intensity associated with the M-NP system was mainly attributed to the lectin receptor-mediated internalization of nanomicelles. It was noticed that non-targeted NPs also showed some uptake which is possibly due to the endocytosis-mediated non-specific internalization.23 Such high uptake of the mannose-targeted system could increase the intracellular concentration as well as the cytotoxic effect of anticancer drugs.
Cellular internalization
The result from the cellular uptake analysis indicates that the mannose-tagged nanomicelles could be more effectively internalized by the cancer cells than the non-targeted nanomicelles. We further investigated the mechanism of cellular uptake in A549 cancer cells. CLSM was used to evaluate the cellular uptake. For this purpose, we stained the lysosome with Lysotracker Green (green color) and the nucleus was stained with DAPI (blue color). As mentioned above, nanomicelles were loaded with rhodamine-b to enable them to fluoresce. As seen (Fig. 5), M-NP-treated cells showed bright red fluorescence compared to the NP-treated cell group indicating an enhanced cellular internalization in the case of ligand-tagged formulations. The M-NP was very well colocalized in the lysosomal region indicating a typical lectin receptor-mediated endocytosis uptake. In contrast, NP did not colocalize appreciably due to a simple gradient-mediated uptake mechanism. It could be expected that the nanomicelles are triggered in the acidic endosomal/lysosomal compartments and leave endosomal/lysosomal compartments via simple diffusion to the nuclear region. Earlier, it has been reported that micellar formulations were internalized in a caveolae-dependent manner wherein nanoparticles rapidly colocalize in the acidic compartments and the released drug will travel to the nuclear region.
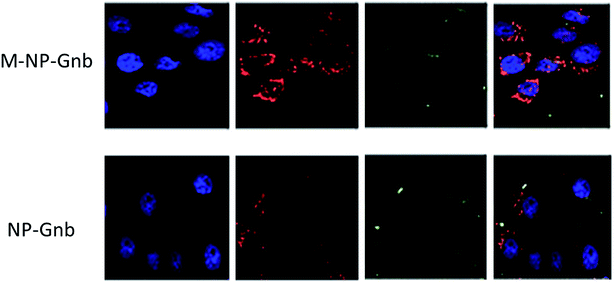 |
| Fig. 5 Cellular internalization of NP-Gnb and M-NP-Gnb in A549 cancer cells. The cells were stained with Lysotracker Green (green color) and DAPI (blue color). | |
Cytotoxicity assay
The cytotoxic potentials of free Gnb and Gnb-loaded nanomicelles were evaluated by means of MTT assay. The results clearly showed a time-dependent and concentration-dependent cytotoxic action of Gnb (free and encapsulated form) in A549 lung cancer cells. The cytotoxic potential of different formulations was consistent with the cell uptake assays (Fig. 6a and b). The IC50 value (the concentration that inhibited cell growth by 50%) was calculated to quantitate the cytotoxic action of individual formulations. The IC50 value of M-NP-Gnb was 0.85 μg ml−1 compared to 2.35 μg ml−1 for NP-Gnb and 4.12 μg ml−1 for free Gnb after 24 h incubation. The superior cytotoxic action of M-NP-Gnb was mainly attributed to the ligand–receptor interactions. The enhanced internalization, high accumulation of nanomicelles due to lectin–mannose interaction, and sustained release characteristics of formulations within the cells could be responsible for the augmented cytotoxic effect.24
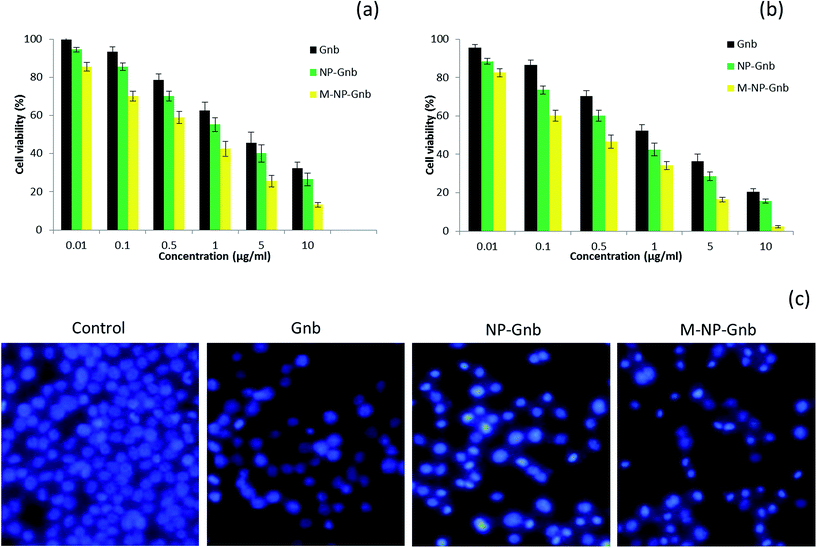 |
| Fig. 6 (a) Cytotoxic effect of free Gnb, NP-Gnb and M-NP-Gnb in A549 cancer cells. The cells were incubated for 24 h (a) and 48 h (b) at 37 °C. (c) The cytotoxic effect of individual formulations was evaluated by means of MTT assay. | |
Hoechst staining was carried out to observe the cellular morphology. As seen, control cells were intact with clear morphology (Fig. 6c). The drug treatment, however, induced remarkable changes in cell morphology. Especially, M-NP-Gnb led to marked changes in cellular and nuclear morphology, including chromatin condensation, membrane blebbing, nuclear breakdown, and the appearance of membrane-associated apoptotic bodies.
Apoptosis assay
The apoptosis effect of individual formulations was studied by FACS analysis using annexin V/PI staining (Fig. 7). The results clearly showed that numbers of apoptotic and necrotic cells increased upon treatment with the formulation. The free Gnb slightly induced cell apoptosis with a marginal amount of cells in the necrotic region. The NP-Gnb, however, showed a higher percentage of cells in the apoptosis and necrotic region. Notably, M-NP-Gnb showed significantly higher proportions of cells in the apoptosis and necrotic stage indicating the superior anticancer effect of mannose-tagged formulations. The enhanced apoptosis of cancer cells was consistent with the higher cellular internalization and higher cell killing effect.
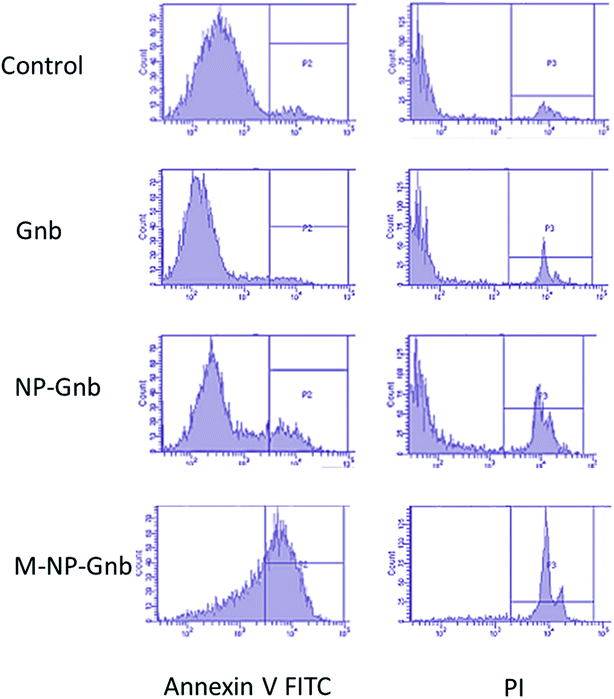 |
| Fig. 7 Annexin V/PI-based apoptosis assay via flow cytometer (FACS). Annexin V was used for apoptosis analysis and PI was used for necrosis analysis. | |
Pharmacokinetic and biodistribution analysis
A pharmacokinetic study has been performed to investigate the systemic performance of free drug as well the drug-loaded nanomicelles. The in vivo study clearly revealed that pharmacokinetics of free drug significantly altered after encapsulation in the nanomicelles. As seen (Fig. 8a), free Gnb was immediately cleared from the systemic circulation within 4–6 h. The NP-Gnb and M-NP-Gnb significantly prolonged the residence time of Gnb up to 24 h. The half-life of Gnb encapsulated in nanomicelles increased about 5-fold compared to that of free Gnb. The augmented half-life clearly indicates the maximum residence time of drug in the systemic circulation. The AUC0–24 and AUC0–∞ values of free Gnb (18.45 μg h ml−1), NP-Gnb (65.92 μg h ml−1) and M-NP-Gnb (68.12 μg h ml−1) further demonstrated the long-time circulation property of nanomicelles. The long-time circulation property of NP-Gnb and M-NP-Gnb was attributed to the presence of PEG on the outer surface of the nanocarrier. The PEG will effectively evade macrophage uptake and augment the half-life of drug in the nanocarrier system. The prolonged blood circulation profile of mannose-conjugated nanomicelles would be crucial for tumor targeting.25 The M-NP-Gnb would be preferentially accumulated in tumor tissues via receptor-mediated endocytosis in mannose receptor overexpressed A549 tumor tissues.
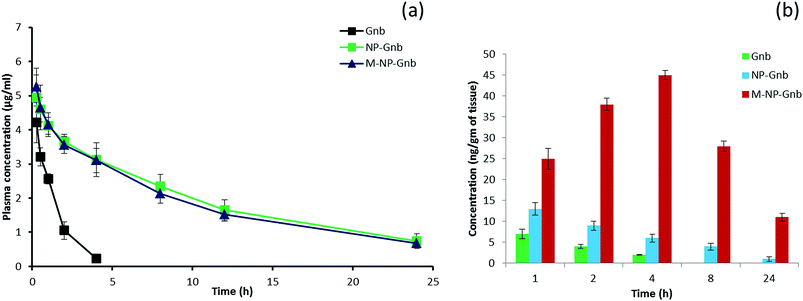 |
| Fig. 8 (a) Plasma concentration–time profile of free Gnb, NP-Gnb and M-NP-Gnb. The study was carried out up to 24 h. Gnb was administered at a fixed dose of 5 mg kg−1 via tail vein. (b) Biodistribution of free Gnb, NP-Gnb and M-NP-Gnb in tumors at various time intervals up to 24 h. | |
A biodistribution study was conducted to further investigate the targeting ability of the mannose-targeted nanosystem. As shown in Fig. 8b, significantly higher concentration of Gnb was accumulated in tumor tissues from the M-NP-Gnb therapeutic system at all time points. Approximately 7-fold higher accumulation of drug was observed in tumor tissues compared to free drug. At the end of 24 h, ∼10 ng g−1 of tissue was observed for the M-NP-Gnb treated mice group whereas none was observed for free drug treated group. The ability of M-NP-Gnb to localize preferentially in tumor tissue indicates its reduced entry to other vital organs. Moreover, it should be noted that the drug level in the M-NP-Gnb treated group kept increasing until 4 h. This may be explained on the basis of the presence of lectin receptors on tumor tissues that favored selective entry of M-NP-Gnb in tumors. The presence of lectin receptors on the tumor surface presents an interesting platform and facilitates targeting. This strategy will help to augment the therapeutic effectiveness by lowering the dose of the therapeutic moiety.
Materials and methods
Materials
PLGA ([η] = 0.32–0.44 dl g−1; 50
:
50) and D-mannose were purchased from Sigma-Aldrich (China). PEG-diamine was purchased from Laysan Bio Inc. (Arab, AL). 1-Ethyl-3-(3-dimethylaminopropyl) carbodiimide (EDC) was purchased from Sigma-Aldrich, China. N-(t-butyloxycarboryl)-1-(2,4-dinitrophenyl)-L-histidine [Boc-His(DNP)-OH] was purchased from Sigma-Aldrich (St Louis, USA). All other chemicals were of reagent grade and used as such without further modification.
Preparation of gefitinib-loaded nanomicelles
The PLGA-PEG polymer block was fabricated using carbodiimide chemistry. In brief, PLGA (10 g) was dissolved in DMSO, and to this solution EDC/NHS was added and the reaction was allowed to continue for 24 h. The so-formed PLGA-NHS was collected by the addition of cold ether and filtered. The PLGA-NHS was reacted with PEG-diamine at a ratio of 6
:
1 in DMSO and further stirred for 20 h at room temperature. The final product, PLGA-PEG-NH2, was precipitated with excess acetonitrile and washed with diethyl ether. The unreacted PEG-diamine was separated by dialysis. The final product, folate–PEG–PLA, was obtained by freeze-drying and was stored in a refrigerator (4 °C).26
mPEG-polyhistidine was synthesized from the precursor Boc-His(DNP)-OH. In brief, 10 g of Boc-His(DNP)-OH was dissolved in dioxane and dichlorosulfoxide was added and stirred for 1 h. The so-formed DNP-NCA-HCl was obtained after washing with dioxane and dried in vacuum. A specific amount of DNP-NCA-HCl was reacted with sodium carbonate in DMF solution for 1 h. The mPEG-NH2 (in DMF) was then added drop-wise to the above mixture and stirred for 48 h at room temperature. The so-formed mPEG-poly(Nim-DNP-histidine) was obtained after filtration and drying in vacuum. Then, mPEG-poly(Nim-DNP-histidine) (0.5 g) was dissolved in 8 ml of DMSO and reacted with 2-mercaptoethanol for 12 h at room temperature. The final product, mPEG-polyhistidine, was obtained after filtration and washing with ethanol and ether. The isolated product was dialyzed against distilled water (molecular weight cut-off = 3500 Da), and then freeze-dried to give the final product.27
The precipitate was redissolved in DMF. The drug-loaded nanomicelles were prepared by a dialysis method. Briefly, Gnb (5 mg), PLGA-PEG-NH2 (20 mg), and pHis-PEG (10 mg) were dissolved in 4 ml of DMSO and stirred for 20 h. The solution was then dialyzed in a large quantity of water (dialysis membrane Mw ∼ 3500) for 48 h. The drug-loaded nanomicelles were collected and subsequently conjugated with D-mannose. D-Mannose (10 μM) was dissolved in sodium acetate buffer (pH 4.0) and stirred for 24 h. The mannosylated nanomicelles were further dialyzed for 48 h to remove the unconjugated mannose. The final product was collected and purified as mentioned above and stored at cold temperatures.
Particle size and zeta potential analysis
The particle size, size distribution, and zeta potential surface charge of the nanomicelles were evaluated by the photon correlation spectroscopy method. A Zetasizer (Malvern Instruments, England) was used to measure the particle size and zeta potential. Samples were suitably diluted and analyzed at a fixed angle of 90°. For zeta potential measurement, the samples were diluted with phosphate buffered saline (PBS; pH 7.4).
In vitro release study
The drug release profile of Gnb from M-NP-Gnb and NP-Gnb was evaluated by means of a dialysis method. For this purpose, 1 mg equivalent of Gnb in formulations were loaded in a dialysis tube (Mw ∼ 3500 Da), sealed at both ends and suspended in a Falcon tube containing 20 ml of release media with 1% Tween 80 to increase the solubility of drug in the medium. The medium was continuously stirred at 100 rpm and the whole assembly was kept at 37 °C. At specific time points, samples were withdrawn from the medium and replaced with equal amount of fresh release medium. The samples were analyzed using HPLC. A Shimadzu HPLC system with a C-18 column was used. 0.02 M dipotassium hydrogen orthophosphate and methanol at a fixed ratio of 10
:
90 was used as a mobile phase. The mobile phase was run at 1 ml min−1 and quantified at 246 nm.
Cell viability assay
A549 lung epithelial cancer cell line was cultured in DMEM supplemented with 10% heat-inactivated fetal bovine serum, 1% streptomycin, and 3 mM glutamine in a 37 °C humidified incubator and 5% CO2 atmosphere. The cell viability of A549 cancer cells was investigated using 3-(4,5-dimethylthiazol-2-yl)-2,5-diphenyltetrazolium bromide (MTT) assay. The cells were seeded into a 96-well plate at a density of 5 × 104 cells per well and allowed to attach for 24 h at 37 °C. The cells were then treated with free Gnb, NP-Gnb and M-NP-Gnb and incubated for 24 h and 48 h, separately. The medium was removed and washed twice with PBS buffer. Cells were treated with MTT solution (10 mg ml−1) and incubated for 4 h at 37 °C, allowing MTT to be reduced by viable cells with the formation of purple formazan crystals. DMSO was added to each well to dissolve the formazan crystals and the absorbance of individual wells was noted at 570 nm using an ELISA plate reader.
Cellular uptake analysis
The cellular uptake of targeted and non-targeted nanomicelles was determined by fluorescence microscopy. Briefly, cells were seeded in a 96-well plate, allowed to attach, and exposed to rhodamine-b-loaded NP and M-NP. The formulations were incubated in a time-dependent manner. The cells were washed twice with PBS to remove the nanocarriers adhering on the cell surface. The uptake was calculated from the absorbance of individual wells which was noted at 570 nm via an ELISA plate reader.
The cellular uptake was further confirmed by a FACS instrument (BD Biosciences FACS Aria, Germany). Briefly, cells were seeded in a 96-well plate, allowed to attach, and exposed to rhodamine-b-loaded NP and M-NP. The formulations were incubated in a time-dependent manner. The cells were washed and trypsinized (0.1% w/v). The cells were pelleted by centrifuging at 1200 rpm for 5 min. The pellets were again re-dispersed in PBS buffer and cell-associated fluorescence was measured by the FACS instrument.
Cell apoptosis analysis
The apoptosis effect of individual formulations was evaluated by annexin-V/PI double staining assay using a flow cytometer. The A549 cells were seeded in a 12-well plate and incubated overnight. The following day, cells were exposed to free Gnb, NP-Gnb and M-NP-Gnb formulations and incubated for 18 h. Untreated cells were considered as a control. Next day, cells were washed with PBS twice, extracted, and re-suspended with binding buffer. Cells were then treated with 5 μl of annexin V-FITC and 5 μl of PI for 5 minutes in the dark at room temperature, and apoptosis was then detected by flow cytometry (BD Biosciences FACS Aria, Germany).
Qualitative apoptosis analysis
The A549 cells were seeded in a 12-well plate (2 × 105 cells per well) and treated with free Gnb, NP-Gnb and M-NP-Gnb formulations and incubated for 24 h. The cells were fixed and exposed to Hoechst 33382 nuclear staining dye. The cellular and nuclear morphology was observed using a fluorescence inversion microscope.
Cellular internalization
The cellular internalization of targeted and non-targeted nanomicelles was studied by means of confocal laser scanning microscopy (CLSM). The cells were seeded in a 6-well plate and allowed to attach for 24 h. The cells were then exposed to rhodamine-b-loaded NP and M-NP and incubated for 1 h. After washing with PBS, cells were stained with Lysotracker Green for lysosomes. The cells were fixed with 4% paraformaldehyde and washed. The cells were again stained with DAPI as a nuclear staining dye. The cellular internalization process was investigated using a confocal microscope (Nikon, Japan).
Pharmacokinetic and biodistribution study
Male Balb/c mice, 4–5 weeks old, weighing 10–20 g, were selected to perform the pharmacokinetic analysis. The animal study protocol was approved (ref no. 2015ANZQ268) by the Institutional Animals Ethical Committee (IAEC), Shandong Cancer Hospital and Institute, China. The entire study was carried out in accordance with the standard institutional guiding principles of IAEC. As per the reported protocol, A549 tumors were generated on the right flank of mice. The mice were divided into 3 groups with 6 mice in each group. Free Gnb, NP-Gnb, and M-NP-Gnb were administered at a fixed dose of 5 mg kg−1 via tail vein. For the biodistribution study, animals were sacrificed at specific time intervals and each tumor was extracted and stored at −80 °C until further analysis. For pharmacokinetic assay, blood samples were collected and centrifuged at 5000 rpm for 10 min and plasma was separated. To 100 μl of plasma, 100 μl of acetonitrile was added and protein was precipitated. The drug was extracted from the organic mixture using centrifugation. The supernatant was collected, filtered through a 0.45 μm filter and injected into an HPLC column. The amount of drug present in the blood as well as in the individual organ was determined using HPLC.
Statistical analysis
Statistical analysis of the data was performed using SPSS 16.0 (SPSS Corp., USA) with one-way ANOVA followed by Tukey's post hoc test. P < 0.05 was considered statistically significant. The data are presented as mean ± SD.
Conclusion
In this study, we have successfully prepared a mannose-conjugated pH-sensitive nanosystem for the effective delivery of Gnb to lung cancer cells. We have successfully demonstrated the potential of mannose-tagged nanomicelles as an efficient vector to transport the anticancer drug. The micelles had nanosized particles with excellent dispersity index. The nanomicelles exhibited a pH-responsive nature with enhanced drug release in acidic conditions. The fluorescence and flow cytometer analysis showed a superior cellular uptake for mannose-tagged nanomicelles. The CLSM study revealed a receptor-mediated cellular internalization process. M-NP-Gnb showed a superior anticancer effect in A549 cancer cells and remarkably inhibited cancer cell proliferation. Furthermore, M-NP-Gnb significantly increased the proportion of cells in the apoptosis and necrosis region. Importantly, the half-life of Gnb encapsulated in nanomicelles increased about 5-fold compared to that of free Gnb. The augmented half-life clearly indicates the maximum residence time of drug in the systemic circulation. Consistently, M-NP-Gnb showed a 7-fold higher accumulation of drug in tumor tissues compared to that of free Gnb. Overall, PLGA/His-based nanomicelles could be a promising delivery system to increase the therapeutic efficiency of Gnb in lung cancers.
Acknowledgements
This work was supported by the traditional Chinese medicine science and technology development plan of Shandong Province (no. 2013-208).
References
- R. Siegel, J. Ma, Z. Zou and A. Jemal, Ca-Cancer J. Clin., 2014, 64, 9–29 CrossRef PubMed.
- C. G. Azzoli, S. Baker and S. Temin, et al., J. Clin. Oncol., 2009, 27, 6251–6266 CrossRef CAS PubMed.
- A. Jemal, F. Bray, M. M. Center, J. Ferlay, E. Ward and D. Forman, Ca-Cancer J. Clin., 2011, 61, 69 CrossRef PubMed.
- D. M. Parkin, F. Bray, J. Ferlay and P. Pisani, Ca-Cancer J. Clin., 2005, 55, 74–108 CrossRef.
- H. Wao, R. Mhaskar, A. Kumar, B. Miladinovic and B. Djulbegovic, Syst. Rev., 2013, 2, 10 CrossRef PubMed.
- S. M. Thomas and J. R. Grandis, Cancer Treat. Rev., 2004, 30, 255–268 CrossRef CAS PubMed.
- T. Kosaka, Y. Yatabe, H. Endoh, K. Yoshida, T. Hida, M. Tsuboi, H. Tada, H. Kuwano and T. Mitsudomi, Clin. Cancer Res., 2006, 12, 5764–5769 CrossRef CAS PubMed.
- J. Hyung Park, S. Kwon, M. Lee, H. Chung, J. H. Kim, Y. S. Kim, R. W. Park, I. S. Kim, S. Bong Seo, I. C. Kwon and S. Young Jeong, Biomaterials, 2006, 27, 119–126 CrossRef PubMed.
- A. Morabito, R. Costanzo, A. M. Rachiglio, R. Pasquale, C. Sandomenico, R. Franco, A. Montanino, E. De Lutio, G. Rocco and N. Normanno, J. Thorac. Oncol., 2013, 8, e59–e60 CrossRef CAS PubMed.
- J. Ozao-Choy, G. Ma, J. Kao, G. X. Wang, M. Meseck, M. Sung, M. Schwartz, C. M. Divino, P. Y. Pan and S. H. Chen, Cancer Res., 2009, 69, 2514–2522 CrossRef CAS PubMed.
- H. Chen, Y. Zhao, H. Wang, G. Nie and K. Nan, Curr. Drug Metab., 2012, 13, 1087–1096 CrossRef CAS.
- F. Danhier, O. Feron and V. Préat, J. Controlled Release, 2010, 148, 135–146 CrossRef CAS PubMed.
- V. P. Torchilin, Adv. Drug Delivery Rev., 2012, 64, 302–315 CrossRef PubMed.
- D. Kim, E. S. Lee, K. T. Oh, Z. G. Gao and Y. H. Bae, Small, 2008a, 4, 2043–2050 Search PubMed.
- D. Kim, E. S. Lee, K. Park, I. C. Kwon and Y. H. Bae, Pharm. Res., 2008, 25, 2074–2082 CrossRef CAS PubMed.
- L. Zhang, F. X. Gu, J. M. Chan, A. Z. Wang, R. S. Langer and O. C. Farokhzad, Clin. Pharmacol. Ther., 2008, 83, 761–769 CrossRef CAS PubMed.
- D. Peer, J. M. Karp, S. Hong, O. C. Farokhzad, R. Margalit and R. Langer, Nat. Nanotechnol., 2007, 2, 751–760 CrossRef CAS PubMed.
- M. E. Davis, Z. Chen and D. M. Shin, Nat. Rev. Drug Discovery, 2008, 7, 771–782 CrossRef CAS PubMed.
- S. Jain and S. P. Vyas, J. Liposome Res., 2006, 16, 331–334 CrossRef CAS PubMed.
- A. Nag and R. C. Ghosh, J. Drug Targeting, 1999, 6, 427–438 CrossRef CAS PubMed.
- K. Osada and K. Kataoka, Adv. Polym. Sci., 2006, 202, 113–153 CrossRef CAS.
- P. Agrawal, U. Gupta and N. K. Jain, Biomaterials, 2007, 28, 3349–3359 CrossRef CAS PubMed.
- M. D. Chavanpatil, A. Khdair and J. Panyam, Pharm. Res., 2007, 24, 803–810 CrossRef CAS PubMed.
- A. Miglietta, R. Cavalli, C. Bocca, L. Gabriel and M. R. Gasco, Int. J. Pharm., 2000, 210, 61–67 CrossRef CAS.
- H. B. Ruttala and Y. T. Ko, Pharm. Res., 2015, 32, 1002–1016 CrossRef CAS PubMed.
- M. Nahar and N. K. Jain, Pharm. Res., 2015, 26, 2588–2598 CrossRef PubMed.
- E. S. Lee, K. Na and Y. H. Bae, J. Controlled Release, 2003, 91, 103–113 CrossRef CAS.
|
This journal is © The Royal Society of Chemistry 2015 |