DOI:
10.1039/C5RA09708H
(Paper)
RSC Adv., 2015,
5, 53054-53062
Enhancement of dielectric permittivity by incorporating PDMS-PEG multiblock copolymers in silicone elastomers†
Received
23rd May 2015
, Accepted 10th June 2015
First published on 10th June 2015
Abstract
A silicone elastomer from PDMS-PEG multiblock copolymer has been prepared by use of silylation reactions for both copolymer preparation and crosslinking. The dielectric and mechanical properties of the silicone elastomers were carefully investigated, as well as the morphology of the elastomers was investigated by SEM. The developed silicone elastomers were too conductive to be utilized as dielectric elastomers but it was shown that when the above silicone elastomers were mixed with a commercial silicone elastomer, the resulting elastomer had very favourable properties for dielectric elastomers due to a significantly increased dielectric permittivity. The conductivity also remained low due to the resulting discontinuity in PEG within the silicone matrix.
Introduction
Dielectric elastomers (DEs) have been studied extensively with respect to finding both new and better elastomer candidates and novel applications.1–4 DEs are elastomers which exhibit a change in size or shape when stimulated by an external electric field. They are also known as “compliant capacitors”, with actuation occurring when electrostatic stress exceeds elastic stress.5 Such properties have enabled DEs to play a significant role in applications as actuators, sensors and generators.
Dielectric elastomers with high relative permittivity possess high electrical energy in the form of charge separation, due to polarisation. In an unactuated state, the elastomer can withstand a given electrical field, the so-called electrical “break-down strength”,6 but above this electrical field the DE will short-circuit. Another common failure associated with DEs is electromechanical instability (EMI), which arises during actuation when attractive forces between the two electrodes become dominant and locally exceed a certain threshold value that cannot be balanced by the material's resistance to compression.7,8 This phenomenon, which is also known as “electromechanical breakdown”, can usually be eliminated by prestretching the elastomer, since prestretching has a combined effect of hardening the silicone elastomer, decreasing film thickness and increasing electrical breakdown strength.9,10
Polydimethylsiloxanes (PDMS), as one promising type of dielectric elastomer, exhibit large ultimate extension.11–14 Despite its significant deformation, the drawback of PDMS is that it has low permittivity, in relation to the net dipole moment (μ), of 0.6–0.9 D.15 On the positive side, PDMS is known to have very low conductivity.16 In contrast, polyethyleneglycols (PEG) show high permittivity as a result of a dipole moment of 3.91 D,17 yet they are incapable of actuating, as they are highly conductive.18 Combining PDMS and PEG as a block copolymer presents the possibility of substantially improving properties such as high permittivity and non-conductivity, whereby PEG enhances permittivity and PDMS facilitates actuation through its non-conductive nature and inherent softness. The synthesis of the PDMS-PEG multiblock copolymer utilised herein is based on hydrosilylation, as shown in Fig. 1.
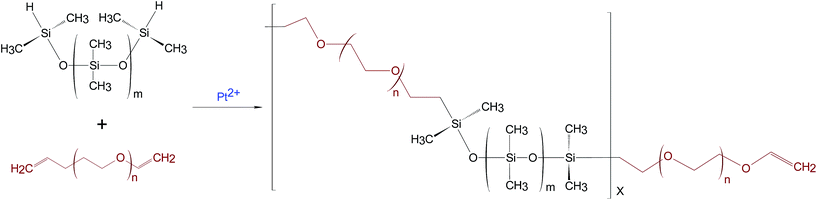 |
| Fig. 1 The hydrosilylation reaction utilized when preparing a PDMS-PEG multiblock copolymer, where m is the number of repeating dimethylsiloxane units in PDMS, and n = 4 is the constant number of repeating ethyleneglycol units in PEG. X is the number of repeating PDMS-PEG units in multiblock copolymers. | |
An astonishing feature of block copolymers is the variety of morphologies due to self-assembly in bulk or in solution.19,20 In principle, a diblock copolymer, which is the simplest block copolymer, assembles into different morphologies, such as sphere (S), cylinder (C), gyroid (G) and lamellar (L).19,21 These morphologies can be achieved when two immiscible, covalently-bonded polymers microphase separately.22 These morphologies can be changed by varying the volume fraction of one constituent in the diblock copolymer. For triblock copolymers, the morphologies are more complex, mainly due to the sequence order of three distinct polymers, e.g. ABC, ACB, BAC and BCA, which introduces further degrees of freedom and thus allows for the assembly of nearly 30 different morphologies.19 The similarity shared by the block copolymers is that they have four common equilibrium morphologies (S, C, G and L).21
Here, elastomers are prepared by means of phase separating PDMS-PEG multiblock copolymers, whereby the copolymers' blocks are expected to segregate to form well-defined structures, depending on the chain lengths of the two constituents. Subsequently the phase-separated copolymers are cross-linked via silylation into elastomers.
Experimental
Materials and reagents
Hydride-terminated polydimethylsiloxanes (H-PDMS) used in the synthesis of the PDMS-PEG multiblock copolymer were DMS-H21, DMS-H11, DMS-H03 and SIH6117.0, each with an average molecular weight (Mn) of 6000, 1050, 550 and 208 g mol−1, respectively. They were purchased from Gelest Inc., while polyethyleneglycol divinyl ether (PEG-DE) was acquired from Sigma Aldrich. A commercial PDMS elastomer [MJK 4/13] was obtained from Wacker Chemie AG, and platinum-divinyl-tetramethyl disiloxane complex [SIP6830.3] was purchased from Gelest Inc. and contained 3.25% of platinum in xylene. A hydride-terminated methyl-hydrosiloxane-dimethylsiloxane copolymer [HMS-501] (Mn of 1050 g mol−1, 9-functional) cross-linker, along with tetravinyltetramethyl-cyclotetrasiloxane [SIT-7900] as an inhibitor, was purchased from Gelest Inc. Both methanol and toluene were purchased from Sigma Aldrich.
Synthesis of the PDMS-PEG prepolymer
The procedure used to synthesise PDMS-PEG multiblock copolymer was amended from that employed by Klasner et al.23 and Jukarainen et al.24 All glassware was thoroughly cleaned and dried at a temperature of 200 °C. The characterisations on Mn of DMS-H21, DMS-H11, DMS-H03, SIH6117.0 and PEG-DE were performed using 1H-NMR to obtain precise Mn for the stoichiometry calculations.
The theoretical PDMS-PEG repeating units in the multiblock copolymer were calculated from a target molecular weight of 30 kg mol−1, whereby the number of blocks for PDMS and PEG were X and (X + 1), respectively:
|
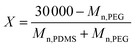 | (1) |
where
Mn,PDMS and
Mn,PEG are the molecular weight of PDMS and PEG, respectively.
The stoichiometric ratio for preparing multiblock copolymers (r1) was calculated as:
|
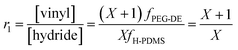 | (2) |
where
fPEG-DE and
fH-PDMS are the functionality of PEG-DE and H-PDMS, respectively.
25 Both polymers in this case were di-functional (
f = 2), and the telechelic vinyl groups of the resulting copolymer were targeted.
Dry toluene (prepared by molecular sieving) was added into the flask at 30 wt% of the total mass of H-PDMS and PEG-DE. The initial concentration of the platinum catalyst was 3120 parts per million (ppm). From this solution, the amount of catalyst solution was determined, in order to obtain a final concentration of 30 ppm in the reaction mixture, by assuming the density of the mixture was 1 g cm−3. The reaction occurred at 60 °C with mild stirring and in the presence of nitrogen gas to eliminate air inside the flask. The duration of the hydrosilylation reaction depended on the chain length of H-PDMS and ranged from 2 to 6 hours. The disappearance of a Si–H bond signal at 4.70 ppm was checked by H-NMR, to ensure that all hydrides in the PDMS had been fully consumed during the reaction; refer to ESI for NMR spectra in Fig. S1a–d.† The final solution was viscous and appeared light bronze in colour. Any remaining solvent (toluene) was removed with a rotary evaporator for a couple of hours. The product was purified by cold methanol precipitation, in order to remove excess PEG-DE, and washing was repeated at least five times. Methanol from the precipitation process was excluded by using a rotary evaporator for a few hours and then placing the mixture in a vacuum for a day.
Experimental setup for the PDMS-PEG block copolymer
To distinguish the PDMS-PEG multiblock copolymer samples from different PDMS volume fractions, they were named based on four different repeating unit numbers in the constituent polymer, as listed in Table 1. Asymmetrical morphologies in the PDMS-PEG multiblock copolymer were obtained by varying PDMS chain lengths (m = 3, 7, 14, 81) while sustaining the equivalent PEG chain length (n = 4), which in turn produced PDMS3-PEG, PDMS7-PEG, PDMS14-PEG and PDMS81-PEG, respectively. Hence PDMS81-PEG constituted the highest volume fraction of PDMS in the block copolymer (0.94), whereas the lowest volume fraction produced in this study was 0.45 (belonging to PDMS3-PEG).
Table 1 Sample details for PDMS-PEG multiblock copolymersa
PDMS-PEG block copolymer |
Number average molecular weight of H-PDMS (Mn,PDMS) [g mol−1] |
Number of repeating units in PDMS (m) |
Theoretical number of repeating units in (PDMS-PEG)X (X) |
Stoichiometric ratio (r1) |
Volume fraction of PDMS (fA) |
Note: Mn of PEG in PDMS-PEG block copolymer is 250 g mol−1. |
PDMS81-PEG |
6000.00 |
81 |
5 |
1.21 |
0.94 |
PDMS14-PEG |
1050.00 |
14 |
23 |
1.04 |
0.75 |
PDMS7-PEG |
550.00 |
7 |
37 |
1.03 |
0.62 |
PDMS3-PEG |
208.00 |
3 |
56 |
1.02 |
0.45 |
Binary polymer blends (BPBs)
PDMS-PEG multiblock copolymers were incorporated into PDMS elastomer (MJK) at 5, 10, 15 and 20 wt%. All mixtures were speedmixed at 3500 rpm for 2 minutes. After that, the blends were immediately cross-linked. The blends produced 16 samples in total.
Cross-linking
Four samples of PDMS-PEG multiblock copolymers and 16 samples of BPB were prepared. The stoichiometric ratio for the cross-linking (r2) was calculated as: |
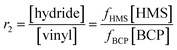 | (3) |
where fHMS and fBCP were the numbers of the HMS-501 (9-functional) functional group and the PDMS-PEG block copolymer (2-functional), respectively, while […] indicates the initial concentration.26,27
The values of r2 were calculated based on the mass of PDMS-PEG prepolymers added into the blends. The inhibitor (SIT7900) and the platinum catalyst were added to the blends at 1 wt% and 30 ppm, respectively. Those blends which consisted of PDMS-PEG prepolymer, namely MJK4/13, SIT7900 and 30 ppm platinum catalyst, were speed-mixed rigorously at 3000 rpm for 2 minutes. Cross-linker (HMS-501) was added, and the resulting mixture was additionally speed-mixed at 1500 rpm for 2 minutes. The cross-linked films were cured at a temperature of 60 °C overnight and then subsequently post-cured at 110 °C for 2 hours.
Characterisations
The NMR equipment utilised in this instance was the Bruker 300 MHz NMR. The number of scannings per sample was 128. The sample was prepared by diluting 50 mg of the sample in 0.5 mL of deuterated chloroform (CDCl3).
Static contact angles, created by using the “sessile drop-needle in” method, were taken at a room temperature of 23 °C using Dataphysics OCA20. The contact angle was measured by dropping 6 μL of deionised water onto the PDMS-PEG multiblock copolymer and BPB films. Measurements for each contact angle were taken for 65 seconds, and the contact angles were analysed every 5 seconds, in order to obtain contact angle versus time profiles.
Linear viscoelasticity (LVE) properties, i.e. storage and loss moduli, were characterised at room temperature using TA Instruments' ARES-G2. The geometry of the parallel plate was 25 mm. The axial force, strain and frequency ranges were 5 N, 2% and 100–0.01 Hz, respectively. The Young's modulus can be determined as Y = 2(1 + ν)G = 3G, since Poisson's ratio (ν) is close to 0.5, due to the incompressibility of silicones.
Dielectric permittivity, loss permittivity and conductivity were measured at a frequency of 106 to 10−1 using a broadband dielectric spectrometer from Novocontrol Technologies GmbH & Co. KG, Germany. The electrode diameter was 20 mm.
The breakdown tests were carried out on an in-house-built device based on international standards (IEC 60243-1 (1998) and IEC 60243-2 (2001)).28 Samples with a film thickness less than 100 μm were used, as breakdown strength depends greatly on sample thickness.10 The film was slid between the two spherical electrodes (radius of 20 mm), and breakdown was measured at the point of contact, with a stepwise increasing voltage applied (50 to 100 V per step) at a rate of 0.5–1 steps per s.29 Each sample was measured up to 12 times, and the average of these values was then taken as the breakdown strength.
The SEM model, FEI Inspect S, used to characterise nanoscale images, performed energy-dispersive X-ray and wavelength dispersive measurements. The accelerating voltage and resolution were 200 V to 30 kV and 50 nm at 30 kV, respectively, while the imaging modes used high and low vacuums.
The number average molecular weight (Mn) determinations for PDMS-PEG multiblock copolymers were performed on an SEC instrument consisting of a Viscotek GPCmax VE-2001 instrument equipped with a Viscotek TriSEC Model 302 triple detector using two PLgel mixed-D columns from Polymer Laboratories. Samples were run in tetrahydrofuran (THF) at 30 °C and at a rate of 1 mL min−1. Molar mass characteristics were calculated using polydimethylsiloxane standards.
Results and discussion
PDMS-PEG multiblock copolymer
The PDMS-PEG block copolymer samples with different PDMS chain-lengths were characterised by means of size-exclusion chromatography (SEC), while the cross-linked samples were analysed by means of dielectric spectroscopy and rheology. Results for the average number of molecular weights obtained from SEC, shown in Table 2, indicate that synthesised PDMS-PEG multiblock copolymers possess lower Mn than targeted.
Table 2 Average number of multiblock copolymers molecular weights
PDMS-PEG block copolymer |
Experimental Mn,T (103 g mol−1) |
PDMS81-PEG |
13 |
PDMS14-PEG |
2.5 |
PDMS7-PEG |
3.6 |
PDMS3-PEG |
1.2 |
The relative permittivity of the multiblock copolymers is shown in Fig. 2. Relative permittivity for the copolymer with the least PEG (PDMS81-PEG) is constant at all frequencies, with a slight increase at low frequencies. This behaviour is similar to that of the reference elastomer (MJK), but the PDMS81-PEG multiblock copolymer has three-fold higher relative permittivity. For samples with higher PEG content, significant relaxation takes place at low frequencies, leading to increased permittivity (as seen in Fig. 3), while dielectric loss also increases very abruptly when decreasing the frequency. This behaviour indicates conductive nature of the elastomers. In Fig. 4 the conductivity of the copolymers is shown. It is obvious that they are all conductive, due to the display of a plateau in conductivity at low frequencies. The block copolymers have conductivities of the order of 102 to 105 higher than those of the reference elastomer (MJK).
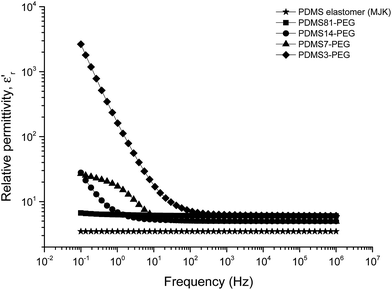 |
| Fig. 2 Relative permittivity of cross-linked PDMS-PEG multiblock copolymers at 23 °C. | |
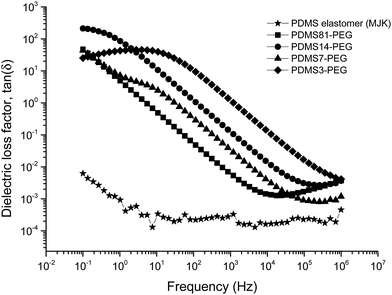 |
| Fig. 3 Dielectric loss factor for cross-linked PDMS-PEG multiblock copolymers at 23 °C. | |
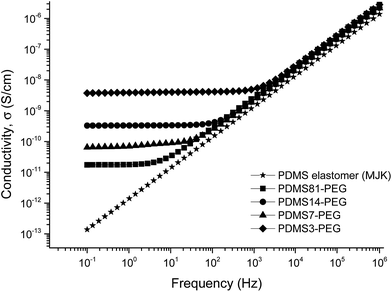 |
| Fig. 4 Conductivity of PDMS-PEG multiblock copolymers at 23 °C. | |
The rheological properties of the cross-linked copolymers are shown in Fig. 5. The PDMS14-PEG and PDMS81-PEG samples show the behaviour of very soft networks with low storage moduli compared to silicone elastomers, and they also demonstrate significant relaxation at low frequencies, which further indicates the inherent softness. In contrast, the PDMS3-PEG and PDMS7-PEG samples possess PEG-like properties with high storage moduli and low losses. Furthermore, their shear modulus is higher than that of the reinforced commercial silicone elastomer. Therefore, it is clear that an increase of PEG constituents in a PDMS-PEG multiblock copolymer reinforces the network comparable with the effect of silica fillers. It is noteworthy that PDMS81-PEG and PDMS14-PEG closely resemble each other despite PDMS81-PEG being significantly shorter than PDMS14-PEG (see Table 2), and thus PDMS81-PEG should provide significantly higher cross-link density and thus higher G. However, this effect cannot be seen simply because the increased content of PEG in PDMS14-PEG has an identical cross-linking effect.
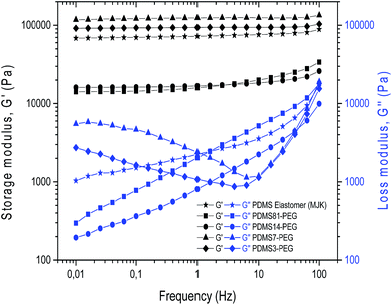 |
| Fig. 5 Comparison between the storage and loss modulus PDMS-PEG multiblock copolymers at 23 °C. | |
Binary polymer block copolymer and silicone elastomer blends
Due to the conductivity of PDMS-PEG multiblock copolymers, they were further blended and cross-linked into a commercial PDMS elastomer (MJK). Incorporating the block copolymers into a silicone network as a binary polymer blend (BPB) can facilitate the creation of PEG spheres, as illustrated in Fig. 6. The blends consist of PDMS-PEG multiblock copolymers at loadings of 5, 10, 15 and 20 wt% and are denoted as MJK/PDMSi, where i = 81, 14, 7, 3. When increasing PEG fractions, unfavourable and discontinuous morphologies may be formed.
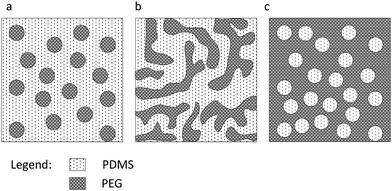 |
| Fig. 6 Illustration of morphologies for BPB of PDMS-PEG block copolymer and silicone elastomer: (a) continuous phase in PDMS (b). Co-continuous phase in PDMS (c). Discontinuous phase in PDMS. | |
Dielectric properties of the binary polymer blends
The relative dielectric permittivity and loss permittivity of the polymer blends are shown in Fig. 7 and 8. Relative permittivities are significantly improved compared to the reference elastomer (MJK), and loss permittivities are substantially lower than those of the pure copolymers – as hypothesised. Refer to ESI Fig. S2–4† for data for all samples.
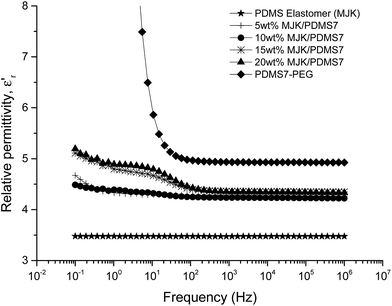 |
| Fig. 7 The relative permittivity of MJK/PDMS7 (5–20 wt% of PDMS7-PEG) at 23 °C. | |
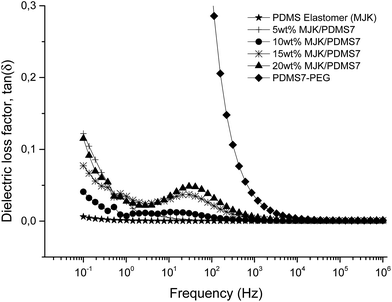 |
| Fig. 8 The dielectric loss factor of MJK/PDMS7 (5–20 wt% of PDMS7-PEG) at 23 °C. | |
In general, the storage permittivity of MJK/PDMS7 increases as the wt% of the PDMS7-PEG multiblock copolymer increases in line with loadings from 5 to 20 wt%. Incorporating 20 wt% of PDMS7-PEG in a PDMS network yields the highest relative permittivity (5.2), which is an increase of 60% compared to the relative permittivity of MJK (3.5). The small increase in relative permittivity at low frequencies for MJK/PDMS7, with 5 and 10 wt%, is due to electrode polarisation effects occurring during the measurement process. However, this can be corrected by applying silicone grease between the sample and the electrode.30 The dynamic dipole orientation of polymer molecules resulting from polarisation are observed for MJK/PDMS7 at 15 and 20 wt%, as Debye-relaxation peaks occur at frequencies of 100 to 103 Hz.
One essential finding from the dielectric characterisation is that none of the polymer blends is conductive. To further analyse the optimum polymer blend, selection based on the sample which gives the lowest dielectric loss factor is carried out. Polymer blends of MJK/PDMS3, MJK/PDMS14 and MJK/PDMS81 possess electrical loss factors in the ranges of 0.5–0.9, 0.25–0.75 and 0.06–1.25, respectively, in the investigated frequency regime. MJK/PDMS7 is the most promising blend, due to a low dielectric loss factor of 0.05–0.125 (Fig. 8).
The behaviour of MJK/PDMS7 non-conductivity with different copolymer loadings is very promising, since no plateau regions are observed at low frequencies (Fig. 9). This implies that a blending method applied properly causes the successful formation of a discontinuous phase for PEG that creates non-conductive behaviour of the developed polymer in the PDMS elastomer and PDMS7-PEG blends at loadings of 5, 10, 15 and 20 wt%. The conductivity of MJK/PDMS7 is consistent with respect to the MJK elastomer, which is non-conductive, as shown in Fig. 9.
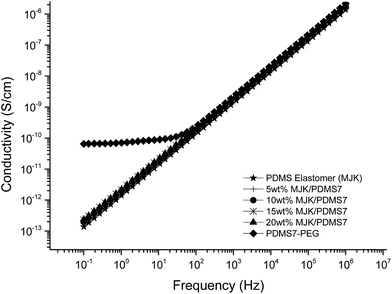 |
| Fig. 9 The conductivity of MJK/PDMS7 (5–20 wt% of PDMS7-PEG) at 23 °C. | |
The low dielectric loss factor and non-conductivity of MJK/PDMS7 for all investigated copolymer loadings indicates that the composites consist of PEG in discontinuous phases.
Rheological properties of BPB
To evaluate the effect of blending on mechanical properties, elastomers from MJK/PDMS7 with a 5–20 wt% copolymer were rheologically characterised, as shown in Fig. 10. The storage modulus of MJK/PDMS7 with 20 wt% is relatively close to the storage modulus of silicone elastomer (MJK). In contrast, MJK/PDMS7 with 5 and 10 wt% is softer than the PDMS elastomer, with storage moduli being one-fold and three-fold lower than the storage modulus of MJK (7 × 105 Pa). The blend of MJK/PDMS7 with 15 wt% is the stiffest, with G′ = 8 × 105 Pa. Another important feature observed from Fig. 10 is the appearance of small relaxation peaks in the loss moduli for 15 and 20 wt%. This is due to the transient nature of the PEG semi-crystalline phases acting as reinforcing domains.
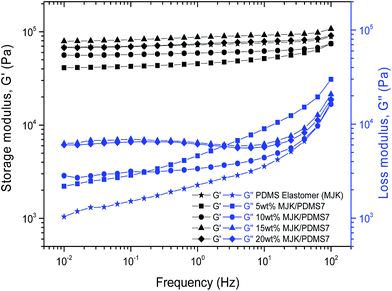 |
| Fig. 10 The storage and loss moduli of MJK/PDMS7 (5–20 wt% of PDMS7-PEG) at 23 °C. | |
All elastomers, however, do show to be well cross-linked and appear very elastic, and therefore they are suitable as soft dielectric elastomers.
Dielectric breakdown (EBD) strength
Electrical breakdown and the influence of different PDMS7-PEG block copolymer loadings in MJK/PDMS7 on the Weibull parameters were investigated. The Weibull fits can be seen in Fig. 11. The Weibull β-parameter (slope of the dashed line in Fig. 11) decreases in line with an increasing MJK/PDMS7 wt%, and it even increases at 20 wt%. The y-axis (Fig. 11) was determined from the formula below: |
ln[−ln(1 − F)] = β ln(EBD) − β ln(η)
| (4) |
where F and EBD were the Weibull cumulative distribution function and electrical breakdown, respectively. The value of the Weibull location parameter η was determined from ln[−ln(1 − F)] = 63.2.
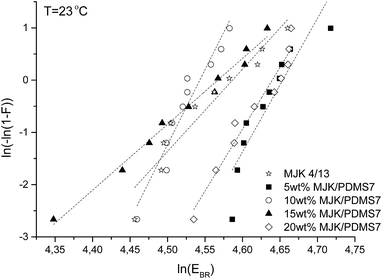 |
| Fig. 11 Cumulative probability of failure of the PDMS elastomer (MJK) and MJK/PDMS7 with 5–20 wt% of the PDMS7-PEG multiblock copolymer (T = 23 °C). The dashed lines represent the linear fit line to the data. | |
Averaged and fitted electrical breakdown data for all the samples are presented in Table 3. MJK/PDMS7 with 5 wt% bears the highest dielectric breakdown strength (103 V μm−1) with a standard deviation of ±4 V μm−1 when averaging over the 12 samples. All samples have an almost identical Weibull η parameter and respective breakdown strengths.
Table 3 Dielectric breakdown strength, Weibull parameters η and β, and R2 of linear fit for the pure silicone elastomer (MJK) and MJK/PDMS7 with 5–20 wt% of the PDMS7-PEG multiblock copolymer
MJK/PDMS7 |
Dielectric breakdown EBD (V μm−1) |
Weibull η-parameter |
Weibull β-parameter |
R2 of linear fit |
MJK |
93 ± 7 |
98 |
17 |
0.92 |
5 wt% |
103 ± 4 |
105 |
31 |
0.84 |
10 wt% |
92 ± 3 |
94 |
31 |
0.93 |
15 wt% |
93 ± 8 |
96 |
13 |
0.99 |
20 wt% |
101 ± 5 |
103 |
25 |
0.95 |
Adding conductive particles usually destabilises the elastomer in respect to electrical breakdown,31 but in the composites investigated herein the conductive PEG clearly stabilises the elastomers, as the β parameters for the composites are significantly larger – and thus the materials will be more electrically stable. This may be due to the charge-trapping effects of PEG.10 The trapping effect probably decreases in line with increased loadings, and thus there is an optimum in the composition at which the electrical stabilisation is highest. The softest sample (5 wt%) is furthermore very steep, and therefore the effect cannot be attributed to increased Young's moduli, as shown in Vudayagiri et al.28
Figure of merit (FOM)
One method which can be used to evaluate the actuation performance of the elastomer is by means of a figure of merit for dielectric elastomer actuators, FOM(DEA), derived by Sommer-Larsen and Larsen:32 |
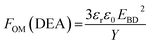 | (5) |
where EBD is electrical breakdown, ε0 is vacuum permittivity (8.85 × 10−12 F m−1), εr is relative permittivity and Y is the Young's modulus.
The FOM(DEA) for the MJK/PDMS7 samples was determined relative to the absolute value of the FOM(DEA) of Elastosil RT625 (1.86 × 10−24%), as reported by Vudayagiri et al.28 The normalised FOM(DEA) was calculated as:
|
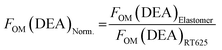 | (6) |
The calculated figures of merit are shown in Table 4. The composite with 5 wt% has the highest normalised FOM(DEA) value at 17, i.e. 17 times greater actuation than the reference elastomer. This composition is the best-performing elastomer amongst those investigated, due to the combination of high electrical breakdown strength, a low Young's modulus and relatively high dielectric permittivity.
Table 4 Normalised FOM(DEA) and Young's modulus (Y) for MJK/PDMS7 with 5–20 wt% of PDMS7-PEG multiblock copolymer
MJK/PDMS7 |
Young's modulus, Ya (kPa) |
Normalised FOM(DEA) |
Young's modulus calculated from Y = 3G′. |
0 wt% (MJK) |
205 |
6.1 |
5 wt% |
123 |
17.2 |
10 wt% |
169 |
9.6 |
15 wt% |
238 |
8.0 |
20 wt% |
203 |
11.2 |
Contact angles of BPB
The wettability of MJK/PDMS7 polymer blends was evaluated by static contact angle measurements. The nature of the PDMS-PEG multiblock copolymer is known as one of the amphiphilic dynamic polymer chains. Similar to MJK/PDMS7, which consists of PDMS7-PEG block copolymers in the PDMS matrix, the trend on wettability leans toward amphiphilic behaviour. In Fig. 12, the contact angles of MJK/PDMS7 for different wt% (5, 10, 15 and 20) decline steeply for the first 20 s and are followed by a slight decrease until they are almost stable at the end of the time period. This indicates that the block copolymer in the polymer blends orients its polymer chains in order to achieve the lowest possible surface energy, since the copolymer comprises blocks of both hydrophobic PDMS and hydrophilic PEG. When the developed elastomer is exposed to air, the surface is controlled by the hydrophobic PDMS from the block copolymer and the matrix, but upon contact with water the chains re-orient and the PDMS blocks migrate back into the bulk material and are replaced by the more hydrophilic PEG blocks at the surface.23 This behaviour is confirmed by the contact angle measurement, where the rearrangement of the polymer chains accounts for the change in contact angle over time when a droplet of deionised water is dropped onto the top surface of the sample. Thus, classing the wettability of MJK/PDMS7 as amphiphilic is the result of incorporating the PDMS7-PEG multiblock copolymer in the network, since PEGs are well-known for their hydrophilic properties.
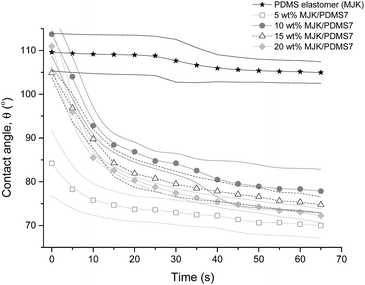 |
| Fig. 12 MJK/PDMS7 contact angles (5–20 wt% of PDMS7-PEG) at 23 °C. | |
SEM analysis
In order to verify the hypothesised structure of the composites, the prepared films were investigated by SEM; the microscope pictures are shown in Fig. 13. For MJK/PDMS7 with 5 wt% copolymer loading, a rough surface is obtained. There are no visible PEG domains observed, and the composite appears homogeneous on the microscale. When the loading of the block copolymer increases from 10 to 20 wt%, the microspherical domains become visible and the number of microspheres increases in line with an increased concentration of PEG. The domains were analysed using Image Processing and Analysis software (ImageJ). The domain sizes of visible spherical domains for MJK/PDMS7 at 10, 15 and 20 wt% are 1.3 ± 0.2 μm, 1.3 ± 0.2 μm and 1.6 ± 0.2 μm, respectively. The observation of spherical domains is coherent with the samples from Liu et al.,33 who observed pores on composite samples of PDMS and PEG etched with ethanol.33 The obtained morphologies indicate that the methodology of blending polymers creates the good dispersion of multiblock copolymers in a silicone network where the spherical domain size seems independent on concentration, as the chain length of the PEG was not a variable in this study. Since the composite with the lowest concentration of PEG possesses different morphology, and at the same time possesses the best overall properties for actuation and lifetime, it may be argued that the introduction of additional surfaces into the system is unfavourable, especially as these surfaces may increase permittivity but they also destabilise the elastomer.
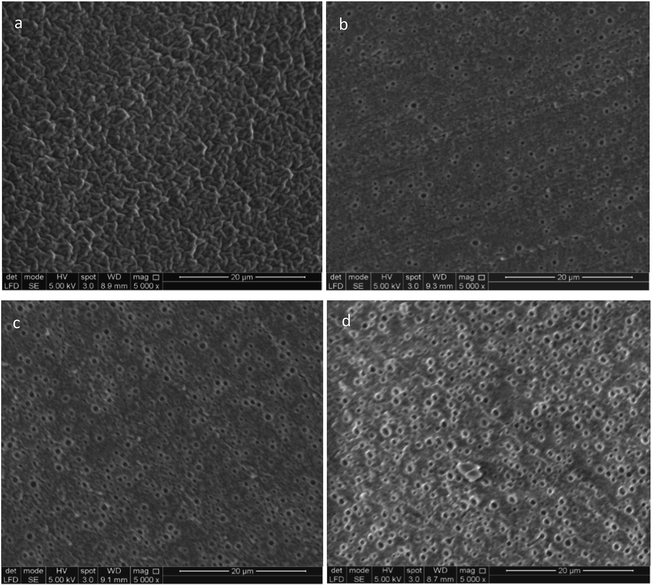 |
| Fig. 13 SEM images of MJK/PDMS7 at: (a) 5 wt% (b). 10 wt% (c). 15 wt% (d). 20 wt%. | |
Conclusion
A new composite elastomer, which has high relative and low permittivity, was successfully created from a binary system of polymer blends consisting of conducting PDMS7-PEG multiblock copolymer and non-conducting PDMS elastomer (MJK). The desired morphology (discontinuous phase of the block copolymer and continuous phase of PDMS) was successfully created in the blends, thereby indicating the development of non-conductive behaviour in the elastomer. Low copolymer loading is favourable, since it creates a homogeneous elastomer on the micro-scale which in turn facilitates a more electrically stable elastomer. Even though the PDMS7-PEG multiblock copolymer is conductive and has high loss permittivity, a good composite elastomer can be developed by incorporating the block copolymer into a silicone network at different wt% and by employing a proper mixing technique. The dielectric breakdown strengths for cross-linked MJK/PDMS7 polymer blends were relatively high, with values in the order of 100 V μm−1. Finally, by integrating all the characterised parameters, i.e. Young's modulus, breakdown strength and relative permittivity, figures of merit for the dielectric elastomer actuation of the various MJK/PDMS7s were determined, and it was concluded that by incorporating low concentrations of PEG, actuation could be improved 17-fold along with the extension to the lifetime of the dielectric elastomer.
Acknowledgements
The Malaysian Ministry of Education (MoE) and Universiti Tun Hussein Onn Malaysia (UTHM), as well as Innovationsfonden Danmark, are gratefully acknowledged for their funding.
Notes and references
- S. Bauer, S. Bauer-Gogonea, I. Graz, M. Kaltenbrunner, C. Keplinger and R. Schwödiauer, Adv. Mater., 2014, 26, 149–162 CrossRef CAS PubMed
. - O. A. Araromi, I. Gavrilovich, J. Shintake, S. Rosset, M. Richard, V. Gass and H. R. Shea, IEEE ASME Trans. Mechatron., 2014, 20, 438–446 CrossRef
. - I. A. Anderson, I. A. Ieropoulos, T. McKay, B. O'Brien and C. Melhuish, IEEE ASME Trans. Mechatron., 2011, 16, 107–111 CrossRef
. - T. G. McKay, B. M. O'Brien, E. P. Calius and I. A. Anderson, Appl. Phys. Lett., 2011, 98, 1–3 CrossRef PubMed
. - R. Pelrine, R. Kornbluh, J. Joseph, R. Heydt, Q. Pei and S. Chiba, Mater. Sci. Eng., C, 2000, 11, 89–100 CrossRef
. - J. L. Bigue, P. Chouinard, S. Proulx, G. Miron and J. S. Plante, Cansmart Work, 2014, 303–314 Search PubMed
. - X. Zhao and Z. Suo, Appl. Phys. Lett., 2007, 91, 24–27 Search PubMed
. - J. Plante and S. Dubowsky, Proc. SPIE, 2006, 6168, 61681J Search PubMed
. - R. Kornbluh, R. Pelrine, Q. Pei, R. Heydt, S. Stanford, S. Oh and J. Eckerle, Proc. SPIE, 2002, 4698, 254–270 CAS
. - S. Zakaria, P. H. F. Morshuis, M. Y. Benslimane, L. Yu and A. L. Skov, Smart Mater. Struct., 2015, 24, 1–10 CrossRef CAS
. - F. B. Madsen, A. E. Daugaard, C. Fleury, S. Hvilsted and A. L. Skov, RSC Adv., 2014, 4, 6939–6945 RSC
. - P. Brochu and Q. Pei, Macromol. Rapid Commun., 2010, 31, 10–36 CrossRef CAS PubMed
. - F. B. Madsen, L. Yu, A. E. Daugaard, S. Hvilsted and A. L. Skov, RSC Adv., 2015, 5, 10254–10259 RSC
. - B. Kussmaul, S. Risse, M. Wegener, M. Bluemke, J. Krause, J. Wagner, T. Feller, K. Clauberg, J. Hitzbleck, R. Gerhard and H. Krueger, Proc. SPIE, 2013, 8687, 86872S Search PubMed
. - J. N. Lee, C. Park and G. M. Whitesides, Anal. Chem., 2003, 75, 6544–6554 CrossRef CAS PubMed
. - Y. Poojari and S. J. Clarson, J. Inorg. Organomet. Polym. Mater., 2009, 20, 46–52 CrossRef PubMed
. - R. J. Sengwa, K. Kaur and R. Chaudhary, Polym. Int., 2000, 608, 599–608 CrossRef
. - W. Tiejun, Q. I. Yingqun, X. U. Jingkun, H. U. Xiujie and C. Ping, Chin. Sci. Bull., 2003, 48, 2444–2445 Search PubMed
. - Y. Mai and A. Eisenberg, Chem. Soc. Rev., 2012, 41, 5969–5985 RSC
. - A. L. Larsen and E. M. Terentjev, Macromolecules, 2006, 39, 9497–9507 CrossRef CAS
. - F. S. Bates and G. H. Fredrickson, Phys. Today, 1999, 52, 32–38 CrossRef CAS PubMed
. - O. Gazit, R. Khalfin, Y. Cohen and R. Tannenbaum, J. Phys. Chem. C, 2009, 113, 576–583 CAS
. - S. A. Klasner, E. C. Metto, G. T. Roman and C. T. Culbertson, Langmuir, 2009, 25, 10390–10396 CrossRef CAS PubMed
. - H. Jukarainen, S. Clarson, J. Seppala and L. Oy, Am. Chem. Soc., 2000, 729, 353–357 CAS
. - M. P. Stevens, Polymer Chemistry (An Introduction), Oxford University Press, New York, 3rd edn, 1999 Search PubMed
. - A. L. Larsen, K. Hansen, P. Sommer-Larsen, O. Hassager, A. Bach, S. Ndoni and M. Jørgensen, Macromolecules, 2003, 36, 10063–10070 CrossRef CAS
. - S. M. G. Frankær, M. K. Jensen, A. G. Bejenariu and A. L. Skov, Rheol. Acta, 2012, 51, 559–567 CrossRef
. - S. Vudayagiri, S. Zakaria, L. Yu, S. S. Hassouneh, M. Benslimane and A. L. Skov, Smart Mater. Struct., 2014, 23, 1–15 CrossRef
. - M. Y. Benslimane, H. Kiil and M. J. Tryson, Polym. Int., 2010, 59, 415–421 CrossRef CAS PubMed
. - P. Ben Ishai, M. S. Talary, A. Caduff, E. Levy and Y. Feldman, Meas. Sci. Technol., 2013, 24, 1–21 Search PubMed
. - K. Goswami, A. E. Daugaard and A. L. Skov, RSC Adv., 2015, 5, 12792–12799 RSC
. - P. Sommer-Larsen and A. L. Larsen, Proc. SPIE, 2004, 5385, 68–77 CAS
. - H. Liu, L. Zhang, D. Yang, N. Ning, Y. Yu, L. Yao, B. Yan and M. Tian, J. Phys. D: Appl. Phys., 2012, 45, 1–9 Search PubMed
.
Footnote |
† Electronic supplementary information (ESI) available: 1H-NMR spectra and contact angles of the synthesised PDMS-PEG multiblock copolymers (PDMS81-PEG, PDMS14-PEG, PDMS7-PEG and PDMS3-PEG). Results of dielectric and rheology for binary polymer blends by incorporating PDMS-PEG multiblock copolymers at different wt% (5, 10, 15 and 20) into a PDMS network (MJK). See DOI: 10.1039/c5ra09708h |
|
This journal is © The Royal Society of Chemistry 2015 |