DOI:
10.1039/C5RA09617K
(Paper)
RSC Adv., 2015,
5, 59417-59427
Hydroxyl-containing non-viral lipidic gene vectors with macrocyclic polyamine headgroups†
Received
22nd May 2015
, Accepted 1st July 2015
First published on 1st July 2015
Abstract
Cationic lipids are the most widely used non-viral gene vectors, and it is important to develop novel lipids that have high transfection efficiency (TE) and good biocompatibility. A series of macrocyclic polyamine (cyclen and TACN)-based cationic lipids bearing different hydrophobic tails were synthesized with the ring-opening reaction. Several assays were used to study their interactions with plasmid DNA, and these lipoplexes could efficiently condense DNA into nanoparticles with proper sizes and zeta potentials. CCK-8-based cell viability assays showed relatively lower cytotoxicity of the lipoplexes compared with the commercially available Lipofectamine 2000. With transfection in vitro, lipid 6a had comparable or better TE than Lipofectamine 2000 in both 7402 and A549 cells. Interestingly, the TE of 6a was significantly increased in the presence of serum. The results not only demonstrate that lipid 6a can be a promising non-viral gene vector, they also provide clues for developing cationic gene vectors for future in vivo applications.
1. Introduction
In recent decades, scientists have developed numerous methods and drugs for treating diseases. However, there are still many untreatable diseases, including inherited and acquired disorders, such as cancer, AIDS and Parkinson's syndrome.1–3 The use of gene therapy to treat these diseases has gained considerable interest compared to traditional therapeutics.4 Gene therapy allows for the transfer of a specific exogenous gene (DNA) to unhealthy cells, curing them at the point of the disease's origin.5 Because the spontaneous entry of naked DNA into target cells is impossible, DNA transfer into cells primarily depends on gene transfection vectors. Viral and non-viral vectors were developed as the two major types of gene transfection vectors for in vitro and in vivo use. Compared to viral vectors, non-viral gene delivery vectors offer a safer and simpler method of delivering therapeutic genes. However, the clinical application of gene therapy is still difficult because gene transfection vectors are unsafe and inefficient.6–8 Therefore, it urgently needs to develop non-viral gene transfection vectors that have good transfection efficiency (TE) and biocompatibility.
Cationic lipids, as important non-viral gene transfection vectors, have been researched for many years because of their simple structure, ease of preparation, good repeatability and biocompatibility.9,10 Generally, cationic lipids consist of three parts, a cationic headgroup, hydrophobic domain, and linker. They can form micelles via the hydrophobic effect in solution and then bind anionic DNA molecules to form lipoplexes via electrostatic interactions.11 Thus far, many cationic lipids have been synthesized as candidates for non-viral gene delivery.5 Although some cationic lipids have shown good transfection performance in vitro (such as Lipofectamine 2000™), they are still far from adequate for in vivo gene therapy because of their potential cytotoxicity and poor biocompatibility.6,8 Consequently, it is important to develop novel nontoxic cationic structures with high TEs.
Some strategies have been developed to generate efficient and safe gene transfection vectors. These include the introduction of a zwitterion or electronegative groups to the non-viral gene transfection vectors,12–15 which can weaken the reaction capacity between the vectors and negatively charged serum components. These approaches often showed good biocompatibility and serum-tolerance ability. In this study, we used a ring-opening reaction to introduce a hydroxy group to the headgroup of the lipids, which was expected to improve their serum-tolerance ability and cytotoxicity. Many reports have noted that modifying hydroxy groups on the gene transfection vectors might help reduce their cytotoxicity as well as improve gene delivery efficiency and serum-tolerance ability.16–19 Furthermore, a macrocyclic polyamine such as 1,4,7,10-tetraazacyclododecane (cyclen) or 1,4,7-triazacyclononane (TACN) was used as the headgroup. We previously demonstrated that cyclen and TACN are excellent candidate cationic headgroups.20,21 Previous reports also claimed that oleyl alcohol, cholesterol and tocopherol are the optimal hydrophobic tails.22–24 However, these reported lipids either showed low TE or poor serum-tolerance ability. In this study, we connected the headgroups and tails via ring-opening reaction to form a new hydroxyl group, which was expected to enhance the biocompatibility of the lipids. Furthermore, the structure–activity relationship of these lipids in the DNA interaction and gene delivery are also discussed. We found that lipid 6a may serve as a non-viral gene delivery vector with low toxicity.
2. Experimental section
2.1. Materials and methods
All chemicals and reagents were obtained commercially and were used as received. Absolute chloroform (CHCl3) and dichloromethane (CH2Cl2) were distilled after being dried with calcium hydride (CaH2). Anhydrous acetonitrile (CH3CN) was distilled after being dried with P2O5. Trifluoroacetic acid (CF3COOH) was dried and purified under nitrogen by using standard methods. Column chromatography was performed using 200–300 mesh silica gel. All aqueous solutions were prepared from deionized or distilled water. 1,4,7-Tris(tert-butyloxycarbonyl)-1,4,7,10-tetraazacyclododecane, 1,7-bis(tert-butyloxycarbonyl)-1,4,7,10-tetraazacyclodocane were synthesized according to the literature.25,26 The 1H NMR and 13C NMR spectra were measured on a Bruker AM400 NMR spectrometer. Proton chemical shifts of NMR spectra were given in ppm relative to internals reference TMS (1H, 0.00 ppm). HRMS spectral data was recorded on a Bruker Daltonics Bio TOF mass spectrometer. MicroBCA protein assay kit was obtained from Pierce (Rockford, IL, USA). Luciferase assay kit was purchased from Promega (Madison, WI, USA). Endotoxin-free plasmid purification kit was purchased from TIANGEN (Beijing, China). The plasmids used in the study were pGL-3 (Promega, Madison, WI, USA) coding for luciferase DNA and pEGFP-N1 (Clontech, Palo Alto, CA, USA) coding for EGFP DNA. The Dulbecco's modified Eagle's medium (DMEM), and fetal bovine serum were purchased from Invitrogen Corp. BEL-7402 cells (human hepatocellular carcinoma cell), A549 lung cancer cell lines and HepG2 (liver hepatocellular carcinoma) were purchased from Shanghai Institute of Biochemistry and Cell Biology, Chinese Academy of Sciences.
2.2. Synthesis of cationic lipids
2.2.1. Preparation of compound 3. A mixture of R–OH (20 mmol), epichlorohydrin (5.6 g, 60.5 mmol), sodium hydroxide pellets (2.4 g, 60 mmol), water (1 mL, 56 mmol) and tetrabutylammonium bromide (322 mg, 1 mmol) were stirred for 24 h at 40 °C. If the R–OH was solid, suitable amount of methylene chloride should be added to dissolve R–OH. Then the reaction mixture was filtered off and the solid was washed with dichloromethane. The combined organic layer was dried with anhydrous magnesium sulfate. The solvent and excess epichlorohydrins were distilled off under reduced pressure and the obtained residue was purified by silica gel column chromatography (v/v 40
:
1, PE/EA) to give compound 3 as oil.
Compound 3a (yield 55%). 1H NMR (CDCl3, 400 MHz): δ = 0.68 (s, 3H), 0.87–2.02 (m, 38H), 2.24 (m, 1H), 2.39 (m, 1H), 2.64 (m, 1H), 2.82 (t, 1H), 3.16 (m, 1H), 3.24 (m, 1H), 3.47 (m, 1H), 3.74 (m, 1H), 5.37 (s, 1H).
Compound 3b (yield 89%). 1H NMR (CDCl3, 400 MHz): δ = 0.85 (s, 12H), 1.05–1.61 (m, 24H), 1.80 (m, 2H), 2.08 (s, 3H), 2.14 (s, 3H), 2.18 (s, 3H), 2.57 (t, J = 6.7 Hz, 2H), 2.71 (m, 1H), 2.88 (t, J = 4.6 Hz, 1H), 3.35 (m, 1H), 3.65 (m, 1H), 3.90 (m, 1H).
Compound 3c (yield 75%). 1H NMR (CDCl3, 400 MHz): δ = 0.90 (t, J = 6.8 Hz, 3H), 1.31 (m, 30H), 1.58 (m, 2H), 2.62 (m, 1H), 2.81 (m, 1H), 3.17 (m, 1H), 3.40 (m, 1H), 3.50 (m, 2H), 3.70 (m, 1H).
Compound 3d (yield 69%). 1H NMR (CDCl3, 400 MHz): δ = 0.87 (t, 3H), 1.28 (m, 22H), 1.57 (m, 2H), 2.01 (m, 4H), 2.61 (m, 1H), 2.81 (m, 1H), 3.16 (m, 1H), 3.39 (m, 1H), 3.50 (m, 2H), 3.72 (m, 1H), 5.36 (m, 2H).
2.2.2. Preparation of compound 4 and 5. In a typical procedure, compound 3 (1.27 mmol), cyclen or TACN (1.06 mmol) and 20 mL of anhydrous ethanol were mixed in a flask with magnetic stirring and refluxed for 60 h in an oil bath. After the reaction, the mixture was concentrated to give the crude product of 4 or 5, which were further purified by column chromatography on silica gel (PE/EtOH = 2
:
1).
Compound 4a (yield 62%). 1H NMR (CDCl3, 400 MHz): δ = 0.67 (s, H3, cholesterol-H); 0.85–1.40 (m, H26, cholesterol-H); 1.44–1.46 (d, H27, Boc-H); 1.62–2.40 (m, H14, cholesterol-H); 2.52–2.55 (d, H2, N–CH2); 2.71–2.98 (m, H4, cyclen-H); 3.13–3.72 (m, H16, cyclen-H, O–CH, O–CH2); 3.89 (s, H1, O–H); 5.34 (s, H1, cholesterol-H). 13C-NMR (CDCl3, 100 Hz): 11.8; 18.7; 19.3; 21.0; 22.5; 22.8; 23.8; 24.2; 27.9; 28.2; 28.3; 28.4; 28.5; 28.6; 31.8; 31.9; 35.7; 36.1; 36.8; 37.1; 39.0; 39.1; 39.5; 39.7; 42.3; 48.2; 50.1; 56.1; 56.7; 57.8; 67.9; 71.1; 79.3; 79.6; 121.7; 140.7; 155.5. HR-ESI-MS: 915.7150 ([M + H]+, C53H94N4O8; calcd 915.7150).
Compound 4b (yield 46%). 1H NMR (CDCl3, 400 MHz): δ = 0.83–0.87 (m, H12, CH3); 1.04–1.33 (m, H18, CH2); 1.36–1.38 (m, H3, CH3); 1.45–1.47 (d, H27, Boc-H); 1.50–1.53 (m, H3, CH); 1.72–1.84 (m, H2, CH2); 2.07 (s, H3, PH–CH3); 2.12 (s, H3, PH–CH3); 2.16 (s, H3, PH–CH3); 2.49–2.57 (m, H4, N–CH2, cyclen-H); 2.92 (s, H3, CH2, cyclen-H); 3.19–3.75 (m, H16, cyclen-H, O–CH2, O–CH, CH2); 4.11 (m, H1, O–H). 13C-NMR (CDCl3, 100 Hz): 11.7; 11.8; 12.7; 19.6; 19.7; 20.6; 21.0; 22.6; 22.7; 23.8; 24.4; 24.7; 27.9; 28.5; 28.6; 31.3; 32.7; 37.2; 37.4; 39.3; 40.0; 50.1; 58.2; 58.4; 74.8; 75.5; 79.3; 79.7; 117.5; 122.9; 125.6; 127.6; 147.6; 147.9. HR-ESI-MS: 959.7413 ([M + H]+, C55H98N4O9; calcd 959.7412).
Compound 4c (yield 54%). 1H NMR (CDCl3, 400 MHz): δ = 0.86–0.89 (t, H3, CH3); 1.25 (m, H30, CH2); 1.44–1.46 (d, H27, Boc-H); 1.54–1.57 (m, H2, CH2); 2.35–2.38 (d, H1, N–CH2); 2.51–2.55 (m, H2, cyclen-H); 2.71–2.85 (m, H3, N–CH2, cyclen-H); 3.00 (s, H1, O–CH2); 3.22–3.72 (m, H16, cyclen-H, O–CH, O–CH2); 3.92 (s, H1, O–H). 13C-NMR (CDCl3, 100 Hz): 14.0; 22.6; 28.6; 29.3; 29.4; 29.6; 31.9; 48.2; 49.9; 57.9; 67.7; 71.6; 73.8; 79.3; 79.6; 155.5. HR-ESI-MS: 799.6528 ([M + H]+, C44H86N4O8; calcd 799.6524).
Compound 4d (yield 66%). 1H NMR (CDCl3, 400 MHz): δ = 0.88–0.89 (t, H3, CH3); 1.25 (m, H22, CH2); 1.44–1.46 (d, H27, Boc-H); 1.55 (m, H2, CH2); 1.96–2.04 (m, H4, CH
CH–CH2); 2.35–2.38 (d, H1, N–CH2); 2.51–2.54 (d, H2, cyclen-H); 2.72–2.84 (m, H3, N–CH2, cyclen-H); 3.00 (s, H1, O–CH2); 3.22–3.71 (m, H16, cyclen-H, O–CH, O–CH2); 3.92 (s, H1, O–H); 5.33–5.35 (m, H2, CH
C–H). 13C-NMR (CDCl3, 100 Hz): 14.0; 22.6; 27.2; 28.6; 28.9; 29.1; 29.2; 29.3; 29.4; 29.5; 29.6; 29.7; 31.8; 32.5; 50.0; 57.9; 67.7; 71.6; 73.9; 79.3; 79.7; 129.8; 129.9; 155.5. HR-ESI-MS: 797.6361 ([M + H]+, C44H84N4O8; calcd 797.6367).
Compound 5a (yield 56%). 1H NMR (CDCl3, 400 MHz): δ = 0.69 (s, H3, cholesterol-H); 0.87–1.45 (m, H30, cholesterol-H); 1.49–1.50 (s, H18, Boc-H); 1.53–2.04 (m, H10, cholesterol-H); 2.17–2.23 (m, H1, N–CH2); 2.36–2.48 (m, H2, cyclen-H, N–CH2); 2.66–2.82 (m, H4, cyclen-H); 3.07–3.65 (m, H10, cyclen-H, O–CH, O–CH2); 3.73 (m, H1, O–CH); 4.28 (s, H1, O–H); 5.34 (s, H1, cholesterol-H). 13C-NMR (CDCl3, 100 Hz): 11.8; 18.7; 19.3; 21.0; 22.5; 22.8; 23.8; 24.2; 28.0; 28.2; 28.5; 31.9; 35.7; 36.1; 36.8; 37.2; 39.0; 39.5; 39.7; 42.3; 50.1; 50.4; 51.0; 51.7; 54.0; 54.3; 54.4; 54.6; 55.2; 56.1; 56.7; 63.1; 63.3; 67.8; 68.0; 70.7; 79.6; 79.9; 121.4; 140.9; 155.6; 155.8; 156.6. HR-ESI-MS: 772.6197 ([M + H]+, C46H81N3O6; calcd 772.6204).
Compound 5b (yield 65%). 1H NMR (CDCl3, 400 MHz): δ = 0.85–0.89 (m, H12, CH3); 1.02–1.46 (m, H19, CH2); 1.50–1.51 (s, H18, Boc-H); 1.54–1.57 (m, H2, CH2); 1.64 (m, H3, CH3); 1.72–1.86 (m, H2, CH2); 2.09 (s, H3, PH–CH3); 2.14 (s, H3, PH–CH3); 2.18 (s, H3, PH–CH3); 2.56–2.80 (m, H6, N–CH2, cyclen-H); 3.04–3.77 (m, H12, cyclen-H, O–CH2, CH2); 3.98 (s, H1, O–CH); 4.46 (s, H1, O–H). 13C-NMR (CDCl3, 100 Hz): 11.7; 11.8; 12.7; 18.4; 19.6; 19.7; 20.6; 21.0; 22.6; 22.7; 23.8; 24.4; 24.7; 27.9; 28.4; 28.5; 31.3; 32.7; 37.2; 37.4; 39.3; 40.0; 51.0; 51.7; 54.0; 54.4; 54.7; 55.7; 58.4; 63.2; 63.5; 74.7; 74.9; 75.0; 79.9; 80.0; 117.5; 122.7; 125.7; 127.7; 147.7; 147.8; 147.9; 155.6; 155.7; 155.9; 156.6. HR-ESI-MS: 816.6461 ([M + H]+, C48H85N3O7; calcd 816.6466).
2.2.3. Preparation of lipid 6 and 7. Under ice bath, a solution of trifluoroacetic acid (1.5 mL) in anhydrous dichloromethane (5 mL) was added dropwise to a solution of compound 4 or 5 (0.5 mmol) in anhydrous dichloromethane (15 mL). The obtained mixture was then stirred at room temperature for 6 h. After the reaction solvent was evaporated, anhydrous diethyl ether (3 × 20 mL) was added into the residue and the mixture was stirred for another 20 min. Then the solution was evaporated to give the desired lipids 6 and 7.
Lipid 6a (yield 65%). 1H NMR (CDCl3, 400 MHz): δ = 0.67 (s, H2, cholesterol-H); 0.82–2.68 (m, H41, cholesterol-H); 2.94–3.52 (m, H18, cyclen-H, N–CH2); 3.70–3.75 (m, H2, O–CH, O–CH2); 3.87 (s, H2, O–CH, O–CH2); 5.32 (s, H1, cholesterol-H). 13C-NMR (CDCl3, 100 Hz): 11.8; 13.7; 18.0; 18.1; 18.2; 18.3; 18.7; 19.3; 19.7; 20.2; 21.0; 21.3; 22.5; 22.6; 22.7; 22.8; 22.9; 23.8; 24.2; 25.4; 25.5; 27.9; 28.0; 28.2; 31.4; 31.6; 31.8; 35.5; 35.7; 35.8; 36.1; 36.7; 37.0; 37.9; 39.0; 39.5; 42.3; 43.0; 44.5; 50.1; 50.9; 55.2; 55.6; 56.1; 56.7; 58.4; 64.3; 67.3; 69.9; 70.1; 75.6; 75.8; 79.8; 121.8; 140.4; 141.2. HR-ESI-MS: 615.5580 ([M + H]+, C38H70N4O2; calcd 615.5577).
Lipid 6b (yield 65%). 1H NMR (CDCl3, 400 MHz): δ = 0.84–0.87 (m, H12, CH3); 1.02–1.40 (m, H24, CH2, CH, CH3); 1.47–1.55 (m, H2, CH2); 1.71–1.80 (m, H2, PH–CH3); 2.05 (s, H5, PH–CH3); 2.08 (s, H2, PH–CH3); 2.52–2.55 (m, H2, N–CH2); 2.72–3.08 (m, H17, cyclen-H, CH2); 3.50–3.63 (m, H3, O–CH2, CH2); 3.71–3.76 (m, H1, O–CH); 4.13 (s, H1, O–H). 13C-NMR (CDCl3, 100 Hz): 11.5; 11.7; 12.4; 15.7; 19.6; 19.7; 20.5; 21.0; 22.6; 23.5; 24.4; 24.7; 27.9; 29.6; 31.2; 32.7; 37.2; 37.4; 37.5; 39.3; 40.3; 58.4; 64.3; 67.6; 74.2; 74.9; 117.4; 117.8; 123.1; 125.5; 127.9; 147.1; 148.2. HR-ESI-MS: 659.5837 ([M + H]+, C40H74N4O3; calcd 659.5839).
Lipid 6c (yield 65%). 1H NMR (CDCl3, 400 MHz): δ = 0.86–0.89 (t, H3, CH3); 1.24 (s, H30, CH2); 1.50 (m, H2, CH2); 2.63–2.65 (d, H2, N–CH2); 2.99–3.44 (m, H20, cyclen-H, O–CH2); 3.74–3.75 (m, H1, O–CH); 3.98 (s, H1, O–H). 13C-NMR (CDCl3, 100 Hz): 13.7; 14.0; 18.2; 22.6; 25.9; 29.3; 29.4; 29.6; 29.7; 31.9; 42.8; 44.6; 55.0; 58.4; 64.3; 66.9; 71.7; 72.6. HR-ESI-MS: 499.4948 ([M + H]+, C29H62N4O2; calcd 499.4951).
Lipid 6d (yield 65%). 1H NMR (CDCl3, 400 MHz): 0.85–0.89 (t, H3, CH3); 1.26 (s, H22, CH2); 1.51 (s, H2, CH2); 1.96–2.01 (m, H4, CH
CH–CH2); 2.63 (s, H2, N–CH2); 3.00–3.54 (m, H20, cyclen-H, O–CH2); 3.75–3.77 (m, H1, O–CH); 3.96 (s, H1, O–H); 5.33–5.38 (m, H2, CH
C–H). 13C-NMR (CDCl3, 100 Hz): 14.0; 22.6; 27.1; 29.2; 29.3; 29.4; 29.5; 29.6; 29.7; 31.8; 32.5; 33.6; 44.6; 54.8; 67.1; 71.8; 72.2; 129.7; 129.9; 130.2. HR-ESI-MS: 497.4796 ([M + H]+, C29H60N4O2; calcd 497.4795).
Lipid 7a (yield 65%). 1H NMR (CDCl3, 400 MHz): δ = 0.69 (s, H3, cholesterol-H); 0.84–2.20 (m, H42, N–CH2, cholesterol-H); 2.31–3.80 (m, H16, O–CH, O–CH2, cyclen-H); 3.99 (s, H1, O–H); 5.35 (s, H1, cholesterol-H). 13C-NMR ((D6)DMSO, 100 Hz): 12.1; 15.6; 19.0; 19.5; 21.0; 22.8; 23.0; 23.1; 23.6; 24.3; 27.8; 28.2; 28.4; 31.8; 35.6; 36.1; 37.1; 43.2; 44.5; 49.9; 50.0; 56.6; 59.0; 64.9; 65.3; 67.8; 69.3; 71.2; 121.5; 140.9. HR-ESI-MS: 572.5159 ([M + H]+, C36H65N3O2; calcd 572.5155).
Lipid 7b (yield 65%). 1H NMR (CDCl3, 400 MHz): δ = 0.85–0.89 (m, H12, CH3); 1.03–1.46 (m, H21, CH2); 1.51–1.57 (m, H3, CH3); 1.72–1.83 (m, H2, CH2); 2.07 (s, H3, PH–CH3); 2.08 (s, H3, PH–CH3); 2.12 (s, H3, PH–CH3); 2.54–2.55 (m, H2, N–CH2); 2.80–3.79 (m, H17, cyclen-H, O–CH2, CH2, O–CH); 4.20 (s, H1, O–H). 13C-NMR (CDCl3, 100 Hz): 11.7; 12.4; 18.1; 19.6; 19.7; 20.5; 21.0; 22.6; 22.7; 23.6; 24.4; 24.7; 27.9; 31.2; 32.7; 37.2; 37.4; 39.3; 40.3; 49.5; 58.5; 68.2; 74.5; 74.9; 117.8; 123.1; 125.5; 127.3; 147.0; 148.1. HR-ESI-MS: 616.5417 ([M + H]+, C38H69N3O3; calcd 616.5417).
2.3. Preparation of cationic liposomes
Individual cationic lipid (0.005 mmol) or its mixture with DOPE in the desired mole ratio was dissolved in anhydrous chloroform (2.5 mL) in autoclaved glass vials. Thin films were made by slowly rotary-evaporating the solvent at room temperature. Last trace of organic solvent was removed by keeping these films under vacuum above 8 h. The dried films and Tris–HCl buffer (10 mM, pH 7.4) were preheated to 70 °C, and then the buffer was added to the films resulting in the final lipid concentration of 2.0 mM. The mixtures were vortexed vigorously until the films were completely resuspended. Sonication of these suspensions for 20 min in a bath sonicator at 60 °C afforded the corresponding cationic liposomes that were stored at 4 °C.
2.4. Amplification and purification of plasmid DNA
pGL-3 and pEGFP-N1 plasmids were used. The former one was used as the luciferase reporter gene, which was transformed in JM109 Escherichia coli, and the latter one was used as the enhanced green fluorescent protein reporter gene, which was transformed in E. coli DH5α. Both plasmids were amplified in E. coli grown in LB medium at 37 °C and 220 rpm overnight. The plasmids were purified by an EndoFree Tiangen™ Plasmid Kit. Then, the purified plasmids were dissolved in TE buffer solution and stored at −80 °C. The integrity of plasmids was confirmed by agarose gel electrophoresis. The purity and concentration of plasmids were determined by the ratio of ultraviolet (UV) absorbances at 260 nm to 280 nm.
2.5. Preparation of lipid/DOPE/DNA complexes (lipoplexes)
To prepare the liposome/pDNA complexes (lipoplexes), various amounts of cationic lipids were mixed with a constant amount of DNA by pipetting thoroughly at various N/P ratio, and the mixture was incubated for 30 min at room temperature. The theoretical N/P ratio represents the charge ratio of cationic lipid to nucleotide base (in mole) and was calculated by considering the average nucleotide mass of 350.
2.6. Gel retardation assay
To determine the formation of liposome/pDNA complex (lipoplexes), lipoplexes of various N/P ratios ranging from 0 to 8 were prepared as described above. Constant amount of 0.125 μg DNA was used here; 15 μL of each lipoplexes solution was electrophoresed on the 1% (WV-1) agarose gel containing Gelred and Tris–acetate (TAE) running buffer at 110 V for 30 min. DNA was visualized with a UV lamp using a BioRad Universal Hood II.
2.7. Dynamic light scattering (DLS)
Particle size and zeta potential of liposomes or lipoplexes at various N/P ratios were measured by a dynamic light scattering system (Zetasizer Nano ZS, Malvern Instruments Led) at 25 °C. Lipoplexes were prepared by following the instructions used for transfection and measured with 1 μg DNA per mL sample.
2.8. Ethidium bromide replacement assay
The ability of lipid 6 and 7 to condense DNA was studied using ethidium bromide (EB) exclusion assays. Fluorescence spectra were measured at room temperature in air by a Horiba Jobin Yvon Fluoromax-4 spectrofluorometer and corrected for the system response. EB (5 μL, 1.0 mg mL−1) was put into a quartz cuvette containing 2.5 mL of 10 mM 4-(2-hydroxyethyl)-1-piperazineethanesulfonic acid (HEPES) solution (pH 7.4). After shaking, the fluorescence intensity of EB was measured. Then, CT DNA (10 μL, 1.0 mg mL−1) was added to the solution with mixing. The measured fluorescence intensity is the result of the interaction between DNA and EB. Subsequently, the solutions of lipid 8 (1.0 mM, 2 μL for each addition) were added to the above solution for further measurement. All the samples were excited at 520 nm, and the emission was measured at 600 nm.
2.9. Cell culture
HepG2 cell lines were incubated in Dulbecco's modified Eagle's medium (DMEM), A549 lung cancer cell lines and BEL-7402 cells (human hepatocellular carcinoma cell) were incubated in 1640 containing 10% fetal bovine serum (FBS) and 1‰ antibiotics (penicillin–streptomycin, 10
000 U mL−1) at 37 °C in a humidified atmosphere containing 5% CO2.
2.10. Cellular uptake of plasmid DNA
The cellular uptake of the polymer/fluorescein labeled-DNA complexes was analyzed by flow cytometry. The Label IT Cy5 Labeling Kit was used to label pDNA with Cy5 according to the manufacturer's protocol. Briefly, 7402 cells were seeded in 12-well plates (2.4 × 105 cells per well) and allowed to attach and grow for 24 h. For transfection in the absence of serum, the medium was exchange with serum-free medium. As for transfection in the presence of serum, the medium was exchanged with serum-containing medium. Cells were incubated with Cy5 labeled DNA complexes (2 μg DNA per well, optimal N/P ratio of each sample) in media for 4 h at 37 °C. Subsequently, the cells were washed with 1× PBS and harvested with 0.25% Trypsin/EDTA and resuspended in 1× PBS. Mean fluorescence intensity was analyzed using FACSCalibur flow cytometer (Becton Dickinson and Company). Cy5-labeled plasmid DNA uptake was measured in the FL4 channel using the red diode laser (633 nm). Data from 10
000 events were gated using forward and side scatter parameters to exclude cell debris. The flow cytometer was calibrated for each run to obtain a background level of w1% for control samples (i.e., untreated cells).
2.11. Cytotoxicity assays
Toxicity of lipoplexes toward Bel-7402, A549 and HepG2 cells was determined by using a Cell Counting Kit-8 (CCK-8; Dojindo Laboratories, Kumamoto, Japan). About 10
000 cells per well were seeded into 96-well plates. After 24 h, the medium was replaced with fresh medium containing 10% serum. Optimized lipid/DOPE formulations were complexed with 0.2 μg of DNA at various N/P ratios for 30 min and then added to the cells. After 24 h of incubation, 10 μL CCK-8 was added to each well and the plates were incubated at 37 °C for another 1 h. Then, the absorbance of each sample was measured using an ELISA plate reader (model 680, BioRad) at a wavelength of 450 nm. The cell viability (%) was obtained according to the manufacturer's instruction. Lipoplex prepared from Lipofectamine 2000 was used as control.
2.12. Transfection procedure
Gene transfection of a series of complexes was investigated in Bel-7402, A549 and HepG2 cells. Cells were seeded in 24-well plates (12 × 105 cells per well) and grown to reach 70–80% cell confluence at 37 °C for 24 h in 5% CO2. Before transfection, the medium was replaced with a serum-free or a 10% serum-containing culture medium containing liposomes/pDNA (0.8 μg) complexes at various N/P ratios. After 4 h under standard incubator conditions, the medium was replaced with fresh medium containing serum and incubated for another 20 h.
For fluorescent microscopy assays, cells were transfected by complexes containing pEGFP-N1. After 24 h incubation, GFP-expressed cells were observed with an inverted fluorescence microscope (Nikon Eclipse TE 2000E) equipped with a cold Nikon camera. Control transfection was performed in each case using a commercially available transfection reagent Lipofectamine 2000 based on the standard conditions specified by the manufacture.
For luciferase assays, cells were transfected by complexes containing pGL-3. For a typical assay in a 24-well plate, 24 h post transfection as described above, cells were washed with cold PBS and lysed with 100 mL 1× lysis reporter buffer (Promega). The luciferase activity was measured by microplate reader (Model 550, BioRad, USA). Protein content of the lysed cell was determined by BCA protein assay. Gene transfection efficiency was expressed as the relative fluorescence intensity per mg protein (RLU per mg protein). All the experiments were done in triplicates.
3. Results and discussion
3.1. Synthesis of the cyclen derived lipids
Target lipids 6a, 6b and 7a, 7b were synthesized as shown in Scheme 1. First, the epoxidation derivatives 3a–3d were prepared by reacting R–OH with epichlorohydrin in the presence of sodium hydroxide and water; tetrabutylammonium bromide (TBAB) was used as a phase transfer catalyst. A little dichloromethane should be added to dissolve the solid reactants cholesterol and octadecanol. To evaluate the influence of the number of amines on transfection, the Boc-protected cyclen and TACN were used. The ring-opening reaction was performed between the Boc-protected macrocyclic polyamine (1 and 2) and 3, in the presence of methanol, under reflux. The obtained 4a–d and 5a, b have a hydroxyl group, which has the potential to improve the serum-tolerance ability and cytotoxicity. After the final deprotection with trifluoroacetic acid, the target lipids 6 and 7 were obtained. All novel compounds were characterized using NMR and HRMS.
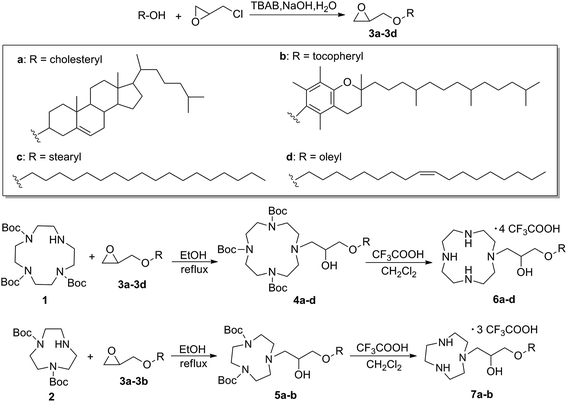 |
| Scheme 1 Synthetic route of the target lipids. | |
3.2. Interactions between the liposomes and plasmid DNA
Cationic liposomes can be formed from either individual cationic lipids or in combination with co-lipids, such as 1,2-dioleoyl-sn-glycero-3-phosphoethanolamine (DOPE), which could improve the endosomal release of lipoplexes and significantly increase the TE.27,28 Herein the liposomes were prepared by mixing title lipid and DOPE in the mole ratio of 1
:
3. As shown in Fig. 1A, all liposomes could completely inhibit the electrophoretic mobility of plasmid DNA at an N/P ratio of 2, except for 6a, which interfered with DNA at the N/P ratio of 4, indicating its weaker DNA binding ability. A gel retardation assay was also performed to examine the DNA binding ability of the liposomes in the presence of serum. These liposomes could effectively retard DNA in the presence of 10% serum (Fig. S1†). Except for 6d, the N/P at which the liposomes could completely inhibit the electrophoretic mobility of plasmid DNA was not changed, indicating that the serum could not induce the dissociation of DNA from the complexes. In summary, serum did not influence the DNA binding capacities. Furthermore, an ethidium bromide (EB) intercalation assay was also used to investigate the interaction between the liposomes and DNA (Fig. S2†). In this assay, EB reveals high fluorescence after intercalating into DNA base pairs, and the fluorescence decreases with the addition of another binding agent that expels EB from its intercalation site.21 All of the liposomes could significantly decrease the fluorescent intensities along with the increase in the N/P ratio (0–10) until they plateaued. Liposomes 6b, 6c and 6d sharply decreased the fluorescent intensities, and from an N/P ratio of 6, only approximately 20% of the original fluorescent was found for 6b, 6c and 6d, indicating they had stronger DNA binding capacity than the other three liposomes. Their strong DNA binding ability may hinder the release of DNA in the cell, reducing their TEs. Conversely, for liposomes 7a and 7b, at N/P of 10, up to ∼49% of the original fluorescent was observed, suggesting their weaker DNA binding ability. This weaker binding suggests that the charge density of the cationic lipid headgroup plays an important role in the DNA binding capacity. Similarly, the weaker DNA binding ability of liposomes 7a and 7b could also reduce the TEs because they could not effectively protect the DNA.
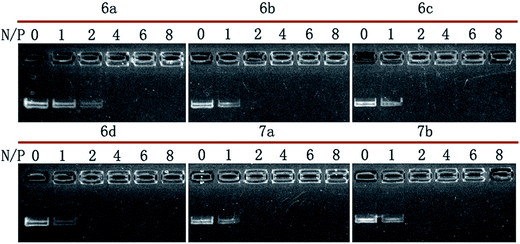 |
| Fig. 1 Electrophoretic gel retardation assays of pDNA in the presence of liposomes at different N/P ratios. “N/P = 0” means that the DNA lacked liposomes. The molar ratio of lipid/DOPE was 1 : 3. | |
Proper sizes and zeta potentials are important factors for the liposome/DNA complexes (lipoplexes) used as gene vectors.29 Dynamic light scattering (DLS) was applied to characterize these properties of the liposomes and lipoplexes. All liposomes had particle sizes in a range of 120–150 nm, indicating that all lipids could form nanoparticles with the neutral liposome DOPE (Fig. S3A†). The morphology of liposomes formed from 6a and 7a was also characterized by transmission electron microscopy (TEM) at N/P ratio of 6 in deionized water (Fig. S4†). It was shown that both of them could form spherical-like nanoparticles with a diameter of 30–70 nm. The particle sizes obtained by TEM were smaller than those measured by DLS, which might be attributed to the fact that the particle sizes measured by DLS were obtained in the hydrated state in deionized water, while those obtained by TEM had been dried after being dropped onto carbon-coated copper meshes.30 The liposomes have positive surface charges in a range of 50–60 mV due to the protonated cyclen and TACN ring, facilitating their electrostatic interaction with the negatively charged DNA (Fig. S3B†). Moreover, the stabilities of the liposomes were also evaluated, and liposomes 6a and 7a had good stability: the particle sizes and zeta-potentials after being stored for 2 weeks were not significantly different compared to the freshly prepared liposomes (Table S1†).
Fig. 2A depicts the mean particle sizes of the 6a–6d and 7a–7b/DNA lipoplexes at different N/P ratios. The average diameters were observed in the range of 120–470 nm, which strongly depended on the N/P ratio. From an N/P ratio of 2, the average diameters gradually decreased with the increase in the N/P; then, they plateaued at approximately 120–170 nm for an N/P ratio of 4, indicating that full DNA condensation was achieved. The surface potentials of the lipoplex nanoparticles were also measured with DLS, and the results are shown in Fig. 2B. The zeta potentials of the six lipoplexes increased from ∼−20 mV to ∼40 mV along with the increase in the N/P ratio (1–10), and they plateaued around ∼20 mV to ∼35 mV for an N/P ratio of 4. This result validated that full DNA condensation was achieved at an N/P of 4.
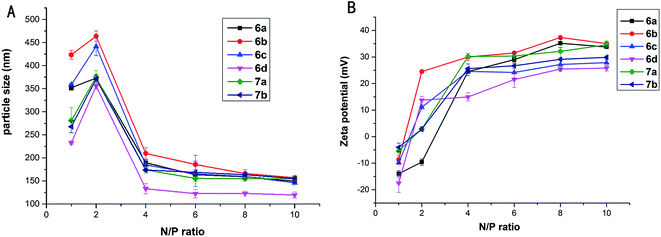 |
| Fig. 2 Mean particle size (A) and zeta-potential (B) of the lipoplexes formed from the six lipids under various N/P ratios (DLS at room temperature). The molar ratio of lipid/DOPE was 1 : 3. Data represent mean ± SD (n = 3). | |
As serum proteins can bind to lipid membranes and increase the liposome size, we also investigated the particle size of the liposomes and lipoplexes in the presence of 10% serum (Table S2†). There was a significant increase in the particle size with serum, indicating that serum components interact with the liposomes and lipoplexes and then, finally, bind to them. Marchini and co-workers found that serum binding to lipoplexes not only increases the particle size, which could change the entry pathway of complexes into the cells, it induced liposome phase transformation from lamellar to inverted hexagonal phases, which could facilitate the intracellular release of DNA and result in a high TE.31 In another word, the influence of serum on TE is not always negative.
3.3. Cytotoxicity
The low cytotoxicity of a synthetic gene delivery carrier is another key factor that enhances its potential application in clinical gene therapy. To investigate the cytotoxicity of the cationic lipoplexes, CCK-8-based cell viability assays were performed in several cell lines with Lipofectamine 2000 as a control. The N/P ratio (2, 4, 6, 8, 10 and 12) and concentration of DNA used in this study were in accordance with those used in the transfection experiments. As shown in Fig. 3, only lipids 6c and 6d showed higher cytotoxicity than Lipofectamine 2000 at the high N/P ratios. Lipids 6a, 6b, 7a and 7b all had much lower cytotoxicity than Lipofectamine 2000 in three cell lines. In HepG2 cells especially, these four lipids showed almost no cytotoxicity, indicating their good biocompatibility. The low cytotoxicity of these lipids might be from the introduction of a hydroxy group to the lipids. In addition, considering the structural difference among these six lipids, we speculated that the cholesterol and tocopherol moieties might be more biocompatible than the alkyl chain moiety in this type of cationic lipid.
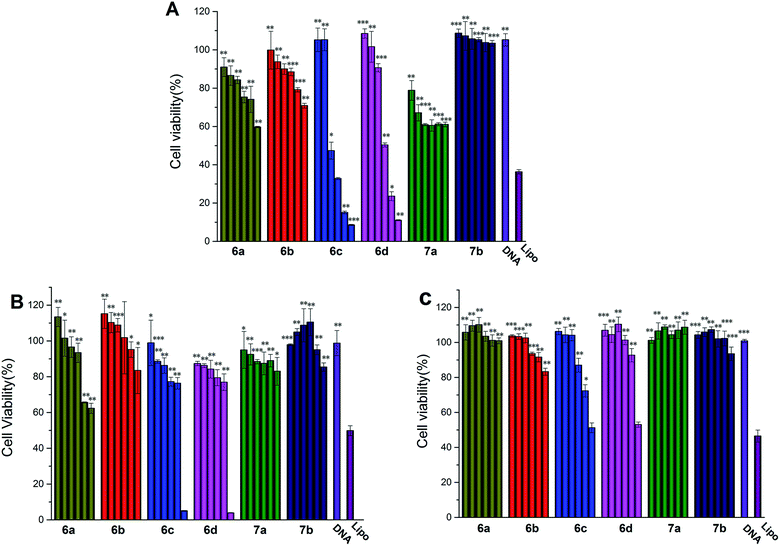 |
| Fig. 3 Cytotoxicity of the lipoplexes at various N/P (2, 4, 6, 8, 10 and 12) ratios in 7402 (A), A549 (B) and HepG2 (C) cells. Data represent the mean ± SD (n = 3). The molar ratio of lipid/DOPE was 1 : 3. *P < 0.05; **P < 0.01; ***P < 0.001 vs. Lipo. Data represent mean ± SD (n = 3). | |
3.4. In vitro gene transfection
In addition to the N/P ratio, the mole ratio of lipid/DOPE in the liposome also affects the TE. To determine the optimized lipid/DOPE ratio, we first performed a fluorescence microscopy assay using pEGFP-N1 plasmid DNA as the reporter gene in 7402 cells. Cationic lipid 6a was used to be mixed with DOPE in various molar ratios (1
:
1, 1
:
2, and 1
:
3) to determine the optimal combination, and the resulting images are shown in Fig. 4. The lipoplexes had evident fluorescent expression, except for the version with a lipid/DOPE mole ratio of 1
:
1. With the increase of the mole ratio, the fluorescent expression became stronger. Based on preliminary results, the lipid/DOPE mole ratio of 1
:
3 was the best combination in the liposomes for efficient transfection. To obtain more accurate results, quantitative luciferase assays were also performed to further optimize the lipid/DOPE ratio in 7402 cells. Lipoplexes were prepared at five mole ratios of lipid/DOPE (1, 2, 3, 4 and 5) for lipid 6a, and the results (at N/P of 8) are shown in Fig. 5. The lipid/DOPE mole ratio of 1
:
3 was the best choice for achieving a high TE, which is consistent with the fluorescence microscopy assay. This result was also consistent with our recent findings.32 Therefore, this mole ratio was used in the subsequent experiments.
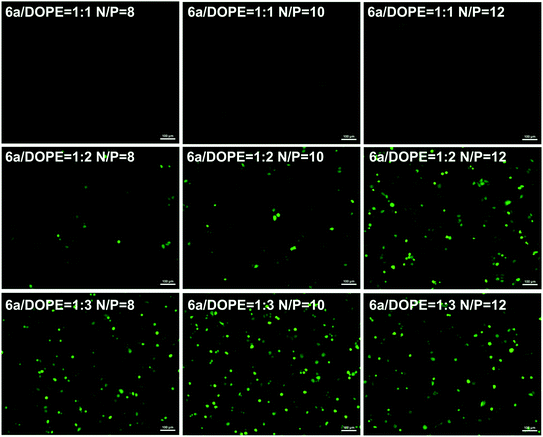 |
| Fig. 4 Fluorescent microscope images of 7402 cells transfected by the lipoplexes formed from 6a at the L/DOPE mole ratio of 1 : 1, 1 : 2, and 1 : 3. The cells were observed by fluorescence microscopy 24 h after transfection. | |
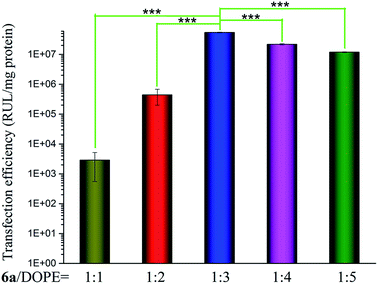 |
| Fig. 5 TEs of 6a derived lipoplexes at various lipid/DOPE ratios with an N/P ratio of 8 in 7402 cells by luciferase transfection assays. ***P < 0.001. Data represent mean ± SD (n = 3). | |
Furthermore, the TE of all title lipids was also investigated under similar transfection conditions, and the results are shown in Fig. 6. In the absence of serum (Fig. 6A), 6a had the best TE at an N/P of 6, and the TE was approximately 1.3 times higher than that of Lipofectamine 2000, indicating its excellent gene transfer ability. In the presence of serum, the best TE of 6a was obtained at an N/P of 8, and it was approximately 2.7 times higher than that of Lipofectamine 2000. Compared with transfection performed in the absence of serum, the TE of 6a was obviously increased. This is unusual because the presence of serum usually decreases the TE.31,33,34 Bhavani also reported that the TEs of some benzothiazole-based cationic lipids, with a hydroxyl group, are unaffected in the presence of 10% serum.19 These results suggested that the modification of a hydroxy group might improve its serum-tolerance ability. In contrast, other lipids had lower TEs compared with Lipofectamine 2000 (Fig. 6B). These results indicated that both the head group and the hydrophobic tail could largely affect the TE of the cationic lipids. The superiority of 6a for gene delivery was also demonstrated by the transfection of EGFP reporter gene. As shown in Fig. S5,† the green fluorescence density for 6a-mediated transfection was much higher than others. Previous reports also found that cationic lipids using cholesterol as hydrophobic tail may improve the TE, because the cholesterol group would help to form rigid and stable bilayer structure, and its endogenous biodegradability and fusion activity also facilitate the transfection.23 For the cationic headgroup, cyclen shows better performance than TACN, this is consistent with literature report11 and our previous studies.
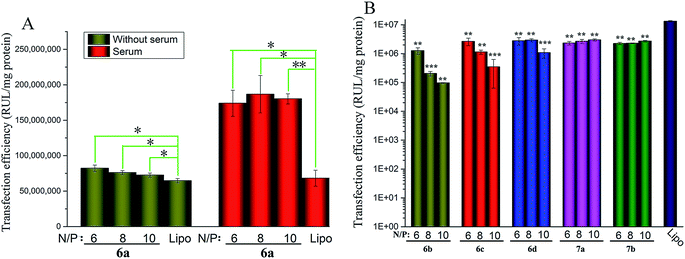 |
| Fig. 6 Luciferase transfection assays (compared to Lipofectamine 2000). (A) TE of 6a derived lipoplexes in the absence of serum and in the presence of serum in 7402 cells. (B) TE of various lipoplexes except 6a at different N/P ratios in the absence of serum in 7402 cells. *P < 0.05; **P < 0.01; ***P < 0.001 vs. Lipo. The molar ratio of lipid/DOPE was 1 : 3. Data represent mean ± SD (n = 3). | |
According to the results obtained in 7402 cells, the most efficient lipid 6a was achieved with the transfection of other cell lines to further investigate its gene delivery efficacy. As shown in Fig. 7A, in A549 cells without serum, 6a had the best TE at an N/P of 10, which was only comparable to Lipofectamine 2000. However, to our delight, in the presence of serum, 6a had a much higher TE than Lipofectamine 2000 at its optimal N/P ratio. Similar to the results in 7402 cells, 6a also showed good serum tolerance. In Fig. 7B, although the TE of 6a was lower than Lipofectamine 2000, the relative TE was also improved in the presence of serum. As a result, lipid 6a might be a promising candidate for future systemic in vivo gene transfection experiments, and it could provide us with clues for the design of serum-compatible non-viral gene delivery vectors.
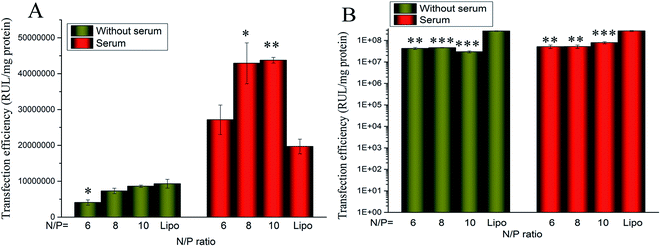 |
| Fig. 7 Luciferase transfection assays (compared Lipofectamine 2000). (A) TE of 6a-derived lipoplexes in A549 cells. (B) TE of 6a derived lipoplexes in HepG2 cells. *P < 0.05; **P < 0.01; ***P < 0.001 vs. Lipo. The molar ratio of lipid/DOPE was 1 : 3. Data represent mean ± SD (n = 3). | |
3.5. Cellular uptake
As one of the barriers in the gene delivery process, the internalization of the nucleic acid complexes largely influences the TE. Flow cytometry was performed to analyze the cellular uptake of DNA. After incubation of the lipoplexes (at the optimal N/P ratio) with 7402 cells for 4 h, the percentage of cells that were positive for Cy5-labeled pDNA was calculated. The cellular uptake of 6a and Lipofectamine 2000 was approximately the same in the absence of serum (Fig. 8A and S6†), but the higher mean fluorescence intensity (Fig. 8B) could contribute to the higher TE of 6a. In addition, lipid 6a had modest DNA binding ability, which might be beneficial to DNA release in cells. Although 6d has the highest cellular uptake, its lower mean fluorescence intensity and stronger DNA binding ability (Fig. 1B) likely cause its lower TE. Similarly, the reason for the low TE of 7a might be caused by its lower mean fluorescence intensity and weaker DNA binding ability (Fig. 2). Serum has obviously negative effect on both cellular uptake and mean fluorescence intensity, especially for 6a. However, 6a had a much higher TE than Lipofectamine 2000 with serum. Marchini and co-workers found that serum could induce the phase transformation of liposomes from the lamellar to inverted hexagonal phases, which could facilitate the intracellular release of DNA.31 Since cellular uptake is only one of several barriers in the gene delivery process,35 the higher TE of 6a in the presence of serum may come from its better intracellular delivery. In-depth transfection mechanism studies of these lipids are underway.
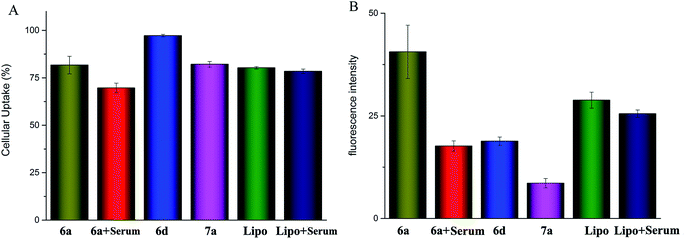 |
| Fig. 8 Cellular uptake (A) and mean fluorescence intensity (B) of lipoplexes at the optimal N/P (N/P = 6 for 6a, N/P = 8 for 6a with serum, N/P = 8 for 6d and N/P = 10 for 7a) ratio in 7402 cells quantified by flow cytometry analysis. The molar ratio of lipid/DOPE was 1 : 3. Data represent mean ± SD (n = 3). | |
4. Conclusion
A series of macrocyclic polyamine (cyclen and TACN)-based cationic lipids, bearing different hydrophobic tails, were synthesized using the ring-opening reaction. All of the liposomes formed from lipids with DOPE could efficiently condense DNA into nanoparticles with a proper size and zeta potential. Furthermore, the cytotoxicity of these lipids was much lower than Lipofectamine 2000. Both the headgroup and hydrophobic tail could affect their TE. Among these lipids, 6a, which had cyclen as a headgroup and cholesterol as a hydrophobic tail, had higher TE than Lipofectamine 2000 in the absence of serum in 7402 cells. As expected, 6a exhibited good serum tolerance, which might be ascribed to the introduction of hydroxyl group. Results indicate the high potential of 6a as a promising non-viral cationic gene vector for future in vivo application.
Acknowledgements
This work was financially supported by the National Program on Key Basic Research Project of China (973 Program, 2012CB720603), National Science Foundation of China (No. 21472131, 21232005), and Specialized Research Fund for the Doctoral Program of Higher Education (20120181130006).
References
- M. Morille, C. Passirani, A. Vonarbourg, A. Clavreul and J.-P. Benoit, Biomaterials, 2008, 29, 3477–3496 CrossRef CAS PubMed.
- H. Jin, A. Kanthasamy, A. Ghosh, V. Anantharam, B. Kalyanaraman and A. G. Kanthasamy, Biochim. Biophys. Acta, Mol. Basis Dis., 2014, 1842, 1282–1294 CrossRef CAS PubMed.
- O. Sankoh, S. Arthur, B. Nyide and M. Weston, HIV & AIDS Review, 2015, 14, 1–8 Search PubMed.
- M. A. Mintzer and E. E. Simanek, Chem. Rev., 2008, 109, 259–302 CrossRef PubMed.
- S. Bhattacharya and A. Bajaj, Chem. Commun., 2009, 4632–4656 RSC.
- C. H. Jones, C.-K. Chen, A. Ravikrishnan, S. Rane and B. A. Pfeifer, Mol. Pharm., 2013, 10, 4082–4098 CrossRef CAS PubMed.
- R. Srinivas, S. Samanta and A. Chaudhuri, Chem. Soc. Rev., 2009, 38, 3326–3338 RSC.
- X.-X. Zhang, T. J. McIntosh and M. W. Grinstaff, Biochimie, 2012, 94, 42–58 CrossRef CAS PubMed.
- M. Tsukamoto, T. Ochiya, S. Yoshida, T. Sugimura and M. Terada, Nat. Genet., 1995, 9, 243–248 CrossRef CAS PubMed.
- Y. Aoshima, R. Hokama, K. Sou, S. R. Sarker, K. Iida, H. Nakamura, T. Inoue and S. Takeoka, ACS Chem. Neurosci., 2013, 4, 1514–1519 CrossRef CAS PubMed.
- D. Zhi, S. Zhang, S. Cui, Y. Zhao, Y. Wang and D. Zhao, Bioconjugate Chem., 2013, 24, 487–519 CrossRef CAS PubMed.
- L. Cheng, Y. Li, X. Zhai, B. Xu, Z. Cao and W. Liu, ACS Appl. Mater. Interfaces, 2014, 6, 20487–20497 CAS.
- X. Guo and L. Huang, Acc. Chem. Res., 2011, 45, 971–979 CrossRef PubMed.
- F. Dai and W. Liu, Biomaterials, 2011, 32, 628–638 CrossRef CAS PubMed.
- K.-M. Xiu, N.-N. Zhao, W.-T. Yang and F.-J. Xu, Acta Biomater., 2013, 9, 7439–7448 CrossRef CAS PubMed.
- X.-h. Luo, F.-w. Huang, S.-y. Qin, H.-f. Wang, J. Feng, X.-z. Zhang and R.-x. Zhuo, Biomaterials, 2011, 32, 9925–9939 CrossRef CAS PubMed.
- Q.-F. Zhang, W.-J. Yi, B. Wang, J. Zhang, L. Ren, Q.-M. Chen, L. Guo and X.-Q. Yu, Biomaterials, 2013, 34, 5391–5401 CrossRef CAS PubMed.
- X. Dong, L. Lin, J. Chen, Z. Guo, H. Tian, Y. Li, Y. Wei and X. Chen, Macromol. Biosci., 2013, 13, 512–522 CrossRef CAS PubMed.
- B. Kedika and S. V. Patri, Eur. J. Med. Chem., 2014, 74, 703–716 CrossRef CAS PubMed.
- H.-J. Wang, Y.-H. Liu, J. Zhang, Y. Zhang, Y. Xia and X.-Q. Yu, Biomater. Sci., 2014, 2, 1460–1470 RSC.
- B. Wang, W.-J. Yi, J. Zhang, Q.-F. Zhang, M.-M. Xun and X.-Q. Yu, Bioorg. Med. Chem. Lett., 2014, 24, 1771–1775 CrossRef CAS PubMed.
- B. Kedika and S. V. Patri, Mol. Pharm., 2012, 9, 1146–1162 CAS.
- D. Zhi, S. Zhang, B. Wang, Y. Zhao, B. Yang and S. Yu, Bioconjugate Chem., 2010, 21, 563–577 CrossRef CAS PubMed.
- B.-K. Kim, K.-O. Doh, J. H. Nam, H. Kang, J.-G. Park, I.-J. Moon and Y.-B. Seu, Bioorg. Med. Chem. Lett., 2009, 19, 2986–2989 CrossRef CAS PubMed.
- E. Kimura, S. Aoki, T. Koike and M. Shiro, J. Am. Chem. Soc., 1997, 119, 3068–3076 CrossRef CAS.
- L. M. De León-Rodríguez, Z. Kovacs, A. C. Esqueda-Oliva and A. D. Miranda-Olvera, Tetrahedron Lett., 2006, 47, 6937–6940 CrossRef PubMed.
- K. Koiwai, K. Tokuhisa, R. Karinaga, Y. Kudo, S. Kusuki, Y. Takeda and K. Sakurai, Bioconjugate Chem., 2005, 16, 1349–1351 CrossRef CAS PubMed.
- S. Gorle, M. Ariatti and M. Singh, Eur. J. Pharm. Sci., 2014, 59, 83–93 CrossRef CAS PubMed.
- S. E. A. Gratton, P. A. Ropp, P. D. Pohlhaus, J. C. Luft, V. J. Madden, M. E. Napier and J. M. DeSimone, Proc. Natl. Acad. Sci. U. S. A., 2008, 105, 11613–11618 CrossRef CAS PubMed.
- M. Ma, F. Li, Z.-f. Yuan and R.-x. Zhuo, Acta Biomater., 2010, 6, 2658–2665 CrossRef CAS PubMed.
- C. Marchini, M. Montani, A. Amici, H. Amenitsch, C. Marianecci, D. Pozzi and G. Caracciolo, Langmuir, 2009, 25, 3013–3021 CrossRef CAS PubMed.
- Z. Huang, Y.-H. Liu, Y.-M. Zhang, J. Zhang, Q. Liu and X.-Q. Yu, Org. Biomol. Chem., 2015, 13, 620–630 CAS.
- J. Turek, C. Dubertret, G. Jaslin, K. Antonakis, D. Scherman and B. Pitard, J. Gene Med., 2000, 2, 32–40 CrossRef CAS.
- J. G. Lewis, K. Y. Lin, A. Kothavale, W. M. Flanagan, M. D. Matteucci, R. B. DePrince, R. A. Mook, R. W. Hendren and R. W. Wagner, Proc. Natl. Acad. Sci. U. S. A., 1996, 93, 3176–3181 CrossRef CAS.
- N. P. Gabrielson and D. W. Pack, Biomacromolecules, 2006, 7, 2427–2435 CrossRef CAS PubMed.
Footnote |
† Electronic supplementary information (ESI) available. See DOI: 10.1039/c5ra09617k |
|
This journal is © The Royal Society of Chemistry 2015 |