DOI:
10.1039/C5RA09157H
(Paper)
RSC Adv., 2015,
5, 53280-53288
Superior As(III) removal performance of hydrous MnOOH nanorods from water†
Received
16th May 2015
, Accepted 10th June 2015
First published on 11th June 2015
Abstract
Hydrous manganite (MnOOH) nanorods were synthesized by a simple precipitation process in ethanol at room temperature, which eliminated high temperature calcination or a hydrothermal process in the creation of most manganese oxide-based adsorbents and resulted in low energy consumption and subsequently low production cost. These MnOOH nanorods had a high specific surface area at ∼165.9 m2 g−1 and their total pore volume was ∼0.561 cm3 g−1, which was beneficial to their arsenic removal performance. These MnOOH nanorods demonstrated a superior As(III) removal performance from an aqueous environment. At near neutral conditions (pH ∼ 7), their arsenic adsorption capacity was over 431.2 mg g−1, which was among the highest reported values in the literature. The superior As(III) removal performance of these MnOOH nanorods relied on the adsorption and subsequent oxidation of As(III) to less mobilized/toxic As(V), and its fixation on their surface to form inner-sphere arsenic surface complexes.
1. Introduction
Arsenic is one of the most toxic heavy metals with its name derived from the Greek word arsenikon. Most environmental arsenic contaminations are the result of its mobilization under natural conditions through natural weathering reactions, biological activities, geochemical reactions, volcanic emissions and so on.1,2 With the rapid development of modern industry, however, more and more of the arsenic contamination accidents which have occurred were caused by anthropogenic activities in recent years.3,4 Arsenic contamination in natural water and the subsequent arseniasis had been reported in many parts of the world, including USA, Canada, China, Argentina, Bangladesh, India and New Zealand,2,5–10 which poses a great threat to the health and everyday life quality of humans worldwide.11 To ensure the drinking water safety and reduce its health risk, the World Health Organization suggested in 1993 that the contaminant level (MCL) for arsenic in drinking water should be reduced from 0.05 mg L−1 to 0.01 mg L−1,12 which had been adopted in many countries and regions.13 Thus, the removal of excess arsenic from water bodies is critical to meet this requirement.
Various technologies had been developed for the removal of arsenic species from contaminated water sources,14 including oxidation/precipitation,15 coagulation,16 ion-exchange technique,17 membrane technique18 and adsorption. Among these technologies, the adsorption is a simple and cost-effective process, especially when the arsenic concentration is low as that in the natural water environment,1,19 and extensive research efforts had been made to develop efficient arsenic adsorbents.20–23 In natural water bodies, arsenic contaminations mainly exist as As(III) (arsenite) and As(V) (arsenate). As(III) is more mobile and difficult to be adsorbed by various adsorbents than As(V) due to its nonionic existence as H3AsO3 in natural water with pH value ranging from weakly acidic to weakly alkaline,15,24 while it is more toxic than As(V) in biological systems.8 Thus, it would be desirable to develop arsenic adsorbents with an oxidation capability to oxidize As(III) to As(V), which could enhance the arsenic adsorption performance to simplify the treatment process and lower the treatment cost while simultaneously reduce the toxicity of arsenic contaminations in water.
Manganese oxide-based materials had been widely utilized in various technical applications, including supercapacitors,25 oxidation catalysis,26,27 photocatalysis,28 cathode materials29 and magnetics.30 For water treatment purpose, manganese oxide-based adsorbents had been used to oxidize and remove toxic metal ions and organic pollutants in water, including copper, lead, aqueous phenol, and dye wastewater.31–33 Both experimental and theoretical studies had been conducted to investigate the oxidation and removal of As(III) from water by manganese oxide-based adsorbents, which demonstrated that they could effectively oxidize As(III) to As(V) and remove both As(III) and As(V) from water.34,35 Most of these studies were focused on the use of synthetic birnessite or binary oxides containing manganese oxide. However, their synthesis usually required high temperature calcination or hydrothermal process, which resulted in high energy consumption and production cost. Thus, it would be of interest to investigate the As(III) removal performance of other manganese oxides, which could be easily synthesized at room temperature with a low production cost.
In this work, hydrous manganite (MnOOH) nanorods were synthesized by a simple precipitation process in ethanol at room temperature. This process eliminated high temperature calcination or hydrothermal process, resulting in low energy consumption and subsequently low production cost. These MnOOH nanorods demonstrated a superior As(III) removal performance from aqueous environment. At near neutral condition (pH ∼ 7), their arsenic adsorption capacity was over 431.2 mg g−1, which was among the highest reported values in literatures for As(III).36–40 The removal of As(III) by these MnOOH nanorods relied on the adsorption and subsequent oxidation of As(III) to less mobilized/toxic As(V) and its fixation on their surface. These MnOOH nanorods may offer a simple one-step treatment option to treat water with high concentration of As(III).
2. Experimental section
2.1. Chemicals and materials
All the chemicals used in the synthesis process were of analytical reagent grade. Manganese(II) chloride tetrahydrate (MnCl2·4H2O, 99%, Sinopharm Chemical Reagent Co., Ltd., Shanghai, P. R. China) was used as the raw material. Ethanol (CH3CH2OH, 99.7%, Sinopharm Chemical Reagent Co., Ltd., Shanghai, P. R. China) was used as the solvent in the synthesis process. Sodium hydroxide (NaOH, 96%, Sinopharm Chemical Reagent Co., Ltd., Shanghai, P. R. China) was used as the precipitation agent in the preparation process. Sodium (meta)arsenite (NaAsO2, Shanghai Tian Ji Chemical Institute, Shanghai, P. R. China) was used to prepare As(III) stock solution. Concentrated hydrochloride acid (HCl, 32–38%, Tianda Chemical Reagents Factory, Tianjin, P. R. China) was used to stabilize the arsenic species after treatment. As(III) solutions used in the batch experiments were obtained by diluting the As(III) stock solution to desired concentration with deionized (DI) water.
2.2. Synthesis of manganite nanorods
Manganite (MnOOH) nanorods were synthesized by a simple process at room temperature. MnCl2·4H2O was first dissolved in 60 mL ethanol to form a 50 mM MnCl2 ethanol solution in a 200 mL beaker. Then, 60 mL of 100 mM NaOH ethanol solution was added drop-wise into it with magnetic stirring at room temperature. By mixing these two solutions, reddish brown precipitates immediately formed, and the color of these precipitates changed from reddish brown to brownish black after being stirred continuously in air for 1 h. The mixture solution was then aged for another 2 h, collected by the centrifugation, washed with DI water for several times until neutral pH and no Cl− ion could be detected by AgNO3 precipitation, and dried in air at 40 °C for 48 h to obtain manganite nanorods. For comparison purpose, part of the obtained MnOOH nanorods were heat treated at 150 °C for 2 h, which resulted in Mn3O4 nanoparticles. Another MnOOH sample without nanostructure was synthesized following a previous report by Pan et al.41 for the examination of nanostructure effect on As(III) removal.
2.3. Material characterization of manganite nanorods
The crystal structure of manganite nanorods was analyzed by the D/MAX-2004-X-ray powder diffractometer (Rigaku Corporation, Tokyo, Japan) with Ni-filtered Cu (0.15418 nm) radiation at 56 kV and 182 mA. BET surface area was measured by N2 adsorption–desorption isotherm with an Autosorb-1 Series Surface Area and Pore Size Analyzers (Quantachrome Instruments, Boynton Beach, FL, USA). Their morphology was observed by both field emission scanning electron microscopy (FESEM) and transmission electron microscopy (TEM). The SEM image was obtained with a SUPRA55 Field Emission Scanning Electron Microscope (ZEISS, Germany). SEM samples were prepared by dispersing MnOOH nanorods in ethanol, applying a drop of the dispersion on a conductive carbon tape, and drying in air. Prior to imaging, the sample was sputtered with gold for 20 s (Emitech K575 Sputter Coater, Emitech Ltd., Ashford Kent, UK). The TEM image was obtained with a JEOL 2010 transmission electron microscope (JEOL Ltd., Tokyo, Japan) operated at 200 kV, with point-to-point resolution of 0.28 nm. TEM samples were prepared by dispersing a thin film of MnOOH nanorods on Cu grid pre-coated with thin and flat carbon film. The semi-quantitative chemical composition and surface chemical states of samples were examined by X-ray Photoelectron Spectrometer (XPS) using an ESCALAB250 X-ray Photoelectron Spectrometer (Thermo Fisher Scientific Inc., Waltham, MA, U.S.A.) with an Al Kα anode (1486.6 eV photon energy, 0.05 eV photon energy resolution, 300 W). The XPS spectra were analyzed and fitted with the XPSPEAK software (version 4.1), and the C 1s peak was set at 284.6 eV as a reference. The electrophoretic spectroscopy was used to analyze the zeta potential of MnOOH nanorods before and after As(III) adsorption. The zeta-potential curves of these MnOOH nanorods dispersed in water at various pH values were measured with a JS84H electrophoretic spectroscope (Shanghai Zhongchen Digital Instrument Co., Ltd., Shanghai, P. R. China). The Fourier transform infrared (FTIR) spectra of MnOOH nanorods before and after As(III) adsorption were measured on a Nicolet 6700 FT-IR Spectrometer (Thermo Fisher Scientific Inc., Waltham, MA, U.S.A.).
2.4. Removal of As(III) from water samples
A series of stock As(III) solutions with high concentrations (ranging from 40 to 500 mg L−1) were prepared by dissolving proper amounts of NaAsO2 into DI water. As(III) solutions with lower concentrations (ranging from 0.1 to 20 mg L−1) were prepared by diluting the 100 mg L−1 stock As(III) solution with DI water. All the experiments of As(III) adsorption were carried out with 500 mL As(III) solutions at about 25 °C. The suspensions were sealed, magnetically stirred at 500 rpm, and their pH values were adjusted at 7 ± 0.3 by adding proper amounts of hydrochloric acid and sodium hydroxide. In the kinetic study of As(III) adsorption on MnOOH nanorods, two adsorbent dosages of 0.05 g L−1 and 0.1 g L−1 were used to examine the material loading effect on As(III) removal. To investigate the initial arsenic concentration effect, the stock solution was diluted to two initial concentrations at ∼1 mg L−1 and 0.1 mg L−1, respectively, which were in the middle to low range of arsenic concentrations found in natural water around the world. The pH value of 0.96 mg L−1 As(III) was measured at ∼7.2 and that of 0.097 mg L−1 As(III) was measured at ∼7.0. During the adsorption process, ∼10 mL suspension was collected at regular intervals for arsenic concentration analysis. Adsorption isotherm experiments were carried out at pH 7 by adding 0.1 g L−1 adsorbent into arsenic solutions with various initial concentrations from ∼1 mg L−1 to ∼500 mg L−1 in a series of reagent flasks, and the suspensions were magnetically stirred for 24 h before collecting samples for analysis. The adsorption capacity at different equilibrium arsenic concentrations could be calculated by the difference between the initial and equilibrium concentrations. After the adsorption experiment, collected suspensions were centrifuged at 12
000 rpm to recover clear top solutions for arsenic concentration analysis, and one drop of concentrated HCl was added into the clear top solution to preserve arsenic species. The samples were analyzed by an atomic fluorescence spectrophotometer (AFS-9800, Beijing KeChuangHaiGuang Instrument Inc., Beijing, P. R. China) to determine the remaining concentrations of As(III). The detection limit of As is 1 ppb, and the instrument was calibrated every day before the measurement with standard As solutions.
3. Results and discussion
3.1. Material characterization results of manganite nanorods
Fig. 1a shows the X-ray diffraction (XRD) pattern of obtained precipitates from the interaction between MnCl2·4H2O and NaOH in ethanol solvent at room temperature. It demonstrated that main XRD peaks belonged to the monoclinic MnOOH (JCPDS card number 41-1379).42 The broadening of these XRD peaks could be mainly attributed to the poor crystallization of these MnOOH nanorods due to their synthesis process under room temperature. During the synthesis process, it was observed that the color of precipitates changed from reddish brown to brownish black within 1 h. It had been reported that the color variation of manganese oxides was correlated with the chemical valence of manganese and deeper color corresponded to higher oxidation state.42,43 The precipitate color change clearly demonstrated that the oxidation state of manganese element changed from Mn2+ to a higher oxidation state in the reaction suspension in air due to its reaction with oxygen, which was in accordance with the XRD analysis result that the final product was MnOOH with manganese element existed as Mn3+.
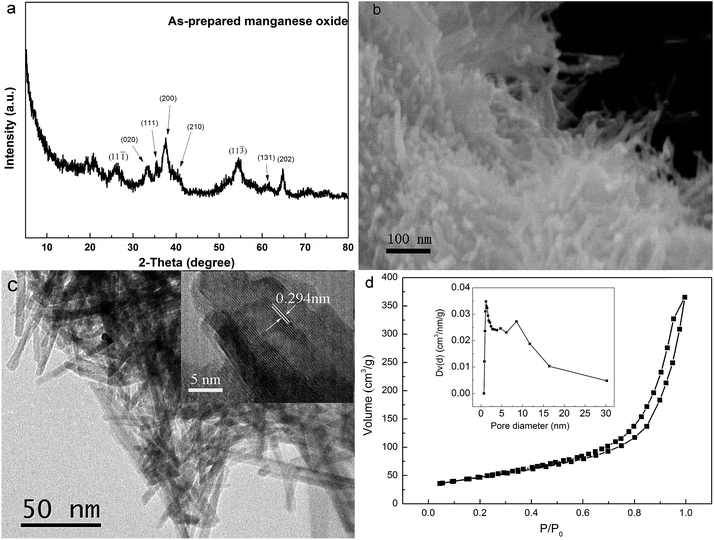 |
| Fig. 1 (a) X-ray diffraction pattern, (b) FESEM image, (c) HRTEM image, and (d) nitrogen adsorption–desorption isotherms of MnOOH nanorods (note: insert plot in (c) shows a higher magnification HRTEM image on several MnOOH nanorods; inset plot in (d) illustrates the corresponding Barrett–Joyner–Halenda pore size distribution curve). | |
The morphology of obtained MnOOH precipitates was examined by both FESEM and HRTEM observations. Fig. 1b shows the FESEM image, which demonstrated clearly that obtained MnOOH precipitates were consisted of aggregations of nanorods. The HRTEM image of MnOOH confirmed their nanorod morphology. It demonstrated clearly that these MnOOH nanorods had an average diameter of ∼5 nm and the length of several tens of nanometers. The insert image in Fig. 1c shows a higher magnification HRTEM image on several MnOOH nanorods, which demonstrated clearly their crystalline structures by the clear crystal lattices. One set of lattice planes could be easily identified with the d-spacing at 0.294 nm, which corresponded to the (11
) plane of the monoclinic structure of MnOOH. Fig. 1d shows the BET measurement curve of MnOOH nanorods. The BET surface specific area of these MnOOH nanorods was determined to be ∼165.9 m2 g−1, which was much higher than their counterparts without nanostructure (∼40 m2 g−1). Higher specific surface area from their nanostructure could be beneficial for their As(III) removal performance. The pore size distribution of these MnOOH nanorods was demonstrated in the insert image in Fig. 1d, which was calculated from the desorption data with the Barrett–Joyner–Halenda (BJH) model. Their total pore volume was ∼0.561 cm3 g−1 and the average pore diameter was ∼6.7 nm. The pore size distribution ranged ∼1.1 nm up to over 30 nm, and most pores were mesopores. Pores with smaller size should come from pores on individual MnOOH nanorods, while pores with larger size should come from pores between individual MnOOH nanorods in their aggregation.
These MnOOH nanorods were synthesized under room temperature in ethanol solvent, which eliminated the use of high temperature treatment adopted in the synthesis of most manganese oxide-based arsenic adsorbents. When a further thermal treatment was conducted, obtained MnOOH nanorods were transferred to Mn3O4. High resolution XPS scans on O 1s spectral region were conducted on both MnOOH and Mn3O4 samples. As demonstrated in Fig. 2, the O 1s spectra of both samples could be best fitted with three overlapped O 1s peaks of oxide oxygen (O2−), hydroxyl group (–OH), and adsorbed water (H2O). The –OH percentage in the total surface oxygen in MnOOH was ∼46.4%, while that of Mn3O4 was only ∼23.3%. The oxygen percentage of MnOOH was ∼75.1%, and that of Mn3O4 was ∼66.2%. Thus, more surface –OH groups could be preserved in MnOOH by our synthesis approach. The elimination of high temperature treatment in the synthesis process also led to the constraint on the grain growth of these MnOOH nanorods, which resulted in their fine size, large surface area, and high pore volume. All of these features were beneficial to their arsenic adsorption.
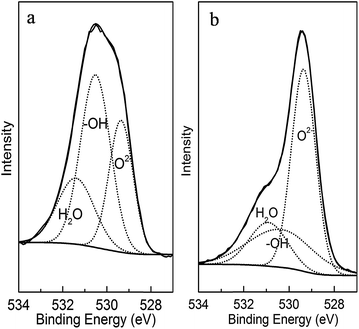 |
| Fig. 2 The surface O 1s high resolution X-ray photoelectron spectra of (a) MnOOH nanorods and (b) Mn3O4. | |
3.2. Kinetic studies on As(III) adsorption on MnOOH nanorods
It had been demonstrated that manganese oxide-based adsorbents could effectively oxidize As(III) to As(V) and remove both As(III) and As(V) from water,34,35 so As(V) could also be found in treated water samples due to the adsorption–desorption equilibrium. Thus, an reduction treatment was conducted on treated water samples to reduce As(V) to As(III) before their arsenic concentration analysis to obtain the total residual arsenic concentrations in these samples. Fig. 3 demonstrates the decrease of total arsenic concentration in lab-prepared water samples with the increase of the treatment time. With the increase of the loading of MnOOH nanorods, the arsenic removal rate increased and the residual arsenic concentration in the water sample decreased. Two initial As(III) concentrations of ∼0.1 mg L−1 and ∼1 mg L−1, respectively, were used in this study, which could represent the low/middle and the high ranges of the arsenic species concentrations in natural water bodies.4 Fig. 3a summarizes the arsenic concentration changes when the initial As(III) concentration was 0.097 mg L−1 with two different loading concentrations at 0.01 g L−1 and 0.03 g L−1, respectively. The results demonstrated that arsenic species could be readily adsorbed onto MnOOH nanorods. For example, when the MnOOH loading concentration was just 0.03 g L−1, the equilibrium arsenic concentration could drop to 0.0028 mg L−1 after the treatment. Thus, MnOOH nanorods were quite effective to remove arsenic species when the As(III) concentration in water samples was relatively low. For a relatively higher arsenic concentration (∼0.96 mg L−1), MnOOH nanorods also demonstrated a good removal effect (see Fig. 3b). With the loading concentration of MnOOH nanorods increased from 0.05 g L−1 to 0.10 g L−1, the equilibrium As concentration decreased from 0.39 mg L−1 to 0.13 mg L−1. When the MnOOH loading concentration was just 0.1 g L−1, which represented a mass ratio of adsorbent to treated water at 1
:
104, ∼87% arsenic species in the solution was removed at the equilibrium. Fig. S1 in the ESI† compares the arsenic concentration changes when the initial As(III) concentration was ∼1.0 mg L−1 and adsorbent loading at 0.10 g L−1 by MnOOH nanorods and their counterparts without nanostructure, respectively. It showed that the As(III) removal performance of MnOOH nanorods was much higher than that of their counterparts without nanostructure under similar experimental conditions, which clearly demonstrated the nanostructure effect and subsequent higher specific surface area on their As(III) removal performance.44
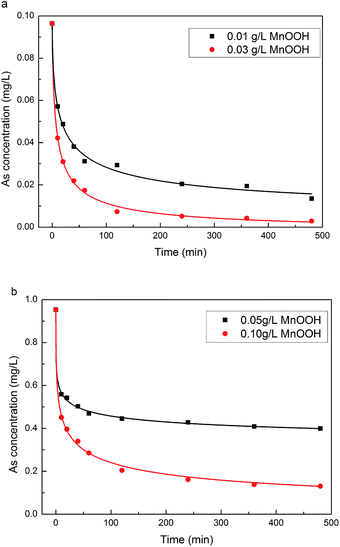 |
| Fig. 3 Adsorption kinetics of As(III) on MnOOH nanorods with initial As(III) concentration at (a) 0.097 mg L−1, and (b) 0.96 mg L−1. | |
The adsorption kinetics study was beneficial for the understanding of the arsenic adsorption mechanism on these MnOOH nanorods. As demonstrated in Fig. 4, the experimental data could be best fitted into a pseudo-second-order kinetic model, which is based on the hypothesis that the rate-limiting step may be chemisorption involving valency forces through sharing or exchange of electrons between sorbent and sorbate. Thus, the adsorption of arsenic species onto MnOOH nanorods should involve chemisorption, which was discussed in details in the FTIR study section. The pseudo-second-order kinetic model could be expressed by eqn (1) and its linear form was given in eqn (2):
|
dqt/dt = Kad(qe − qt)2
| (1) |
|
t/qt = 1/(Kadqe2) + t/qe
| (2) |
where
qe and
qt are the amount (mg g
−1) of arsenic adsorbed at equilibrium and at time
t, respectively, and
Kad is the rate constant of adsorption (g (mg
−1 min
−1)). The kinetics parameters obtained in fitting the experimental data were summarized in
Table 1. The applicability of the pseudo-second-order rate model was quantified by the square of the correlation coefficient
R (
R2), and the closeness of
R2 to 1 indicated that the model fitted the experimental data accurately. With the increase of the loading concentration, the ratio of the arsenic amount adsorbed at equilibrium to the initial As(
III) amount increased, and the rate constant (
Kad) also increased, indicating a faster adsorption rate.
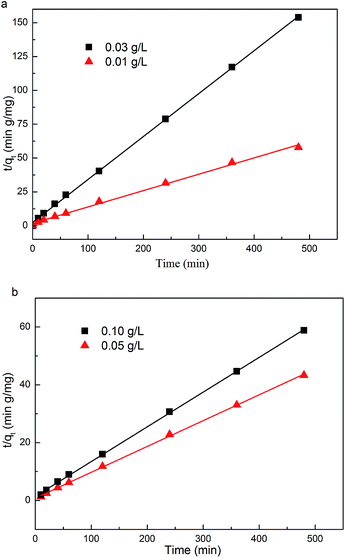 |
| Fig. 4 The pseudo-second-order rate kinetic model fitting of the adsorption kinetics of As(III) on MnOOH nanorods with initial As(III) concentration at (a) 0.097 mg L−1, and (b) 0.96 mg L−1. | |
Table 1 Pseudo-second-order kinetics model fitting parameters for As(III) sorption by MnOOH nanorods
Initial As(III) concentration (mg L−1) |
0.097 |
0.96 |
MnOOH loading (g L−1) |
0.01 |
0.03 |
0.05 |
0.10 |
qe (mg g−1) |
8.28 |
3.15 |
11.2 |
8.33 |
Kad (g mg−1 min−1) |
0.00808 |
0.0409 |
0.00986 |
0.0101 |
R2 |
0.996 |
0.999 |
0.999 |
0.999 |
3.3. Equilibrium adsorption isotherm study of As(III) adsorption on MnOOH nanorods
The adsorption capacity of these MnOOH nanorods on As(III) at near neutral condition (pH ∼ 7) was investigated by the equilibrium adsorption isotherm study with the equilibrium As(III) concentration up to ∼470 mg L−1. As demonstrated in Fig. 5, these MnOOH nanorods demonstrated a superior arsenic adsorption capacity of over ∼430 mg g−1, which was the highest value in literatures.45–49 For example, Zhang et al.49 reported that the sorption capacity of As(III) on iron(III) oxide-loaded melted slag was only 30 mg g−1 in the range of 20–300 ppm concentration, while the adsorbent had a very large SSA of 196 m2 g−1. Manju et al.45 evaluated the As(III) removal performance of coconut husk carbon for industrial wastewater, which showed that the maximum sorption capacity is 146 mg g−1 in the highest As(III) concentration of 600 ppm. In the groundwater As(III) removal research of Guo and Chen,50 the beads cellulose loaded with iron oxyhydroxide were used to remove As(III) and the uptake capacity was ∼99.6 mg g−1 between 0 and 100 mmol L−1.
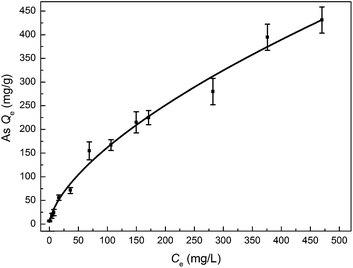 |
| Fig. 5 The equilibrium adsorption isotherm of As(III) on MnOOH nanorods. | |
With the increase of the equilibrium arsenic concentration in a relatively wide range, the amount of arsenic species adsorbed increased steadily. When the equilibrium arsenic concentration increased from ∼0.3 mg L−1 to ∼68.8 mg L−1, the adsorbed arsenic amount by these MnOOH nanorods sharply increased from ∼6.6 mg g−1 to ∼154.8 mg g−1. When the equilibrium As(III) concentration increased from ∼68.8 mg L−1 to ∼470 mg L−1, the adsorbed As amount by these MnOOH nanorods increased from ∼154.8 mg g−1 to ∼431.2 mg g−1 with a relatively slower increase rate. The equilibrium adsorption data could be best fitted with the Freundlich isotherm as follows:
where
qe is the amount (mg g
−1) of arsenic species adsorbed at equilibrium,
Ce is the equilibrium arsenic concentration (mg L
−1) in water samples, and
KF and
n are the Freundlich constants. The parameters obtained in fitting the experimental data were summarized in
Table 2. The closeness of the square of the correlation coefficient
R2 to 1 indicates that the Freundlich isotherm fitted the experimental data well. With the further increase of the equilibrium arsenic concentration, the arsenic amount adsorbed by these MnOOH nanorods could further increased to over 431.2 mg g
−1.
Table 2 Equilibrium adsorption isotherm fitting parameters for As(III) onto MnOOH nanorods
Freundlich |
KF |
9.77 |
n |
1.58 |
R2 |
0.988 |
3.4. Oxidation of As(III) on MnOOH nanorods
To investigate the removal process and mechanism of As(III) on MnOOH nanorods, the surface chemical status of MnOOH nanorod sample before and after As(III) adsorption in water was examined by X-ray photoelectron spectroscopy (XPS). Fig. 6 shows the high resolution XPS scan over As 3d spectral region on MnOOH nanorods after the As(III) adsorption treatment. The As 3d spectrum could be best fitted by the combination of both As(III) 3d peak (44.5 eV) and As(V) 3d peak (45.5 eV). In the initial water solution, only As(III) existed. After the treatment process, As(III) only accounted for 26.6% of the total As on the surface of MnOOH nanorods, while As(V) accounted for 73.4%. This observation demonstrated clearly that the removal of As(III) by these MnOOH nanorods was not only an adsorption process. After As(III) was adsorbed on the surface of these MnOOH nanorods, it was oxidized by MnOOH to As(V). The oxidation reaction could strongly fix arsenic species on the surface of MnOOH nanorods for their removal, and the oxidation of As(III) to As(V) could largely reduce the toxicity of arsenic contamination. We also examined the concentration of As(V) in the solution after the treatment. When the initial As(III) was 10 mg L−1, the adsorbent dosage was 0.1 g L−1, and the adsorption lasted for 8 h to reach the equilibrium, the As(V) concentration in treated solution was 0.41 mg L−1, while the As(III) concentration was 6.2 mg L−1. This observation also clearly indicated that As(III) was oxidized to As(V) during the removal process.
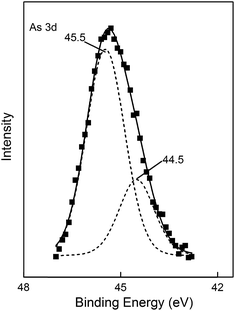 |
| Fig. 6 The high resolution XPS scan over As 3d spectral region on MnOOH nanorods after the As(III) adsorption treatment. | |
3.5. Competing ion effect
Due to the complexity of substances in natural water, competition from other species might exist to deteriorate the arsenic removal performance. Thus, the competing effects of major cations (Na+, Ca2+, Mg2+, and Al3+) and anions (H2PO4−, CO32−, F−, Cl−, and NO3−) in water were examined on the As(III) removal performance of our MnOOH nanorods. The initial As(III) concentration was 0.094 mg L−1, the material dosage was 0.02 g L−1, the solution pH was ∼7.0, and the molar ratio of co-existing ions to As(III) was set at 500
:
1. As demonstrated in Fig. 7, the presence of most co-existing ions in water showed no or just a slight deterioration effect on the As(III) removal performance of our MnOOH nanorods even when their concentrations were much higher than that of As(III) (500 times). Only H2PO4− demonstrated a strong hindrance effect. This observation suggested that these MnOOH nanorods were able to remove As(III) even with exceptionally high concentrations of competing ions used in this study except for H2PO4−.
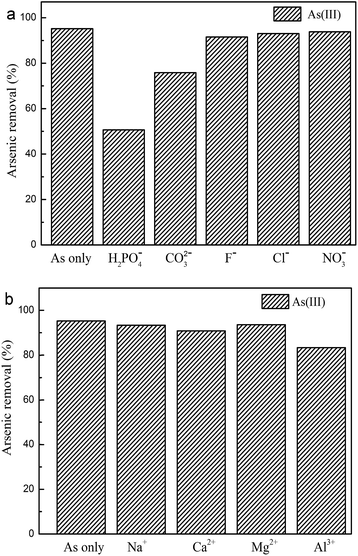 |
| Fig. 7 The competing ion effects on the As(III) removal performance of MnOOH nanorods: (a) common co-existing anions, and (b) common co-existing cations. | |
3.6. The formation of inner-sphere complexes on MnOOH nanorods
Fig. 8a shows the zeta potential curves of the as-prepared MnOOH nanorods and MnOOH nanorods after the adsorption of As(III) (initial concentration of ∼100 mg L−1), respectively. The isoelectric point (IEP) of the as-prepared MnOOH nanorods was determined at pH 4.3. Thus, they were negatively charged at the near neutral environment, indicating the existence of the surface hydroxyl groups. After the adsorption of As(III), the IEP of these MnOOH nanorods decreased to pH 3.3. The IEP of metal oxides is determined by protonation and deprotonation of surface hydroxyl groups. Only the formation of inner-sphere arsenic anionic charged surface complexes could change the surface charge and subsequently cause the shift of IEP to a lower value, while the formation of outer-sphere complexes could not shift the IEP because there is no chemical reaction in this process that could change the surface charge between the adsorbate and adsorbent surface.13,42,51 Thus, the shifting of IEP to a lower value clearly demonstrated the formation of inner-sphere arsenic anionic charged surface complexes on the surface of these MnOOH nanorods.
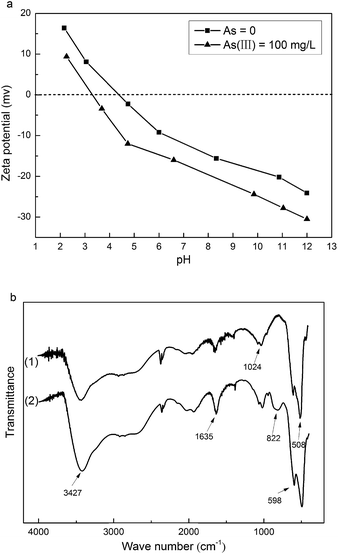 |
| Fig. 8 (a) The zeta potential curves of the as-prepared MnOOH nanorods and MnOOH nanorods after the adsorption of As(III) (initial concentration of ∼100 mg L−1), respectively. (b) The FTIR spectra of the as-prepared MnOOH nanorods and MnOOH nanorods after the adsorption of As(III) (initial concentration of ∼160 mg L−1), respectively. | |
The As(III) oxidation and the formation of inner-sphere arsenic surface complexes on the surface of these MnOOH nanorods could be further demonstrated by the microscopic technique of FTIR spectroscopy. To ensure quantitative analysis, the samples were mixed with KBr at the fixed ratio of 1%. Fig. 8b shows the FTIR spectra of the as-prepared MnOOH nanorods and MnOOH nanorods after the adsorption of As(III) (initial concentration of ∼160 mg L−1), respectively. After the adsorption of As(III), a new peak at ∼822 cm−1 occurred in the FTIR spectrum of MnOOH nanorods, which could be attributed to the stretching vibration of uncomplexed As(V)–O bond.20 The uncomplexed As(V)–O bond of dissolved arsenate species was determined at ∼858 cm−1. The red shift of As(V)–O bond observed here was caused by the decrease of the strength of the uncomplexed As–O bond because of the formation of Mn–O–As bond.52 Thus, FTIR study result demonstrated that the As(III) adsorbed on the surface of MnOOH nanorods was oxidized to As(V), and the formation of the As(V)–O–Mn bond further verified the formation of inner-sphere arsenic anionic charged surface complexes on the surface of these MnOOH nanorods.
4. Conclusions
In summary, a simple and low-cost precipitation process was developed to synthesize hydrous MnOOH nanorods in ethanol at room temperature. These MnOOH nanorods demonstrated a superior As(III) removal performance from aqueous environment. At near neutral condition (pH ∼ 7), their arsenic adsorption capacity was over 431.2 mg g−1, which was among the highest reported values in literature. XPS analysis result demonstrated that most adsorbed As(III) was oxidized to less mobilized/toxic As(V) by these MnOOH nanorods, and zeta potential and FTIR analysis results demonstrated that inner-sphere arsenic surface complexes were formed on their surface for the fixation of arsenic species. With further development, it may offer a simple and one-step treatment option to effectively remove As(III) contamination from water, especially at high As(III) concentration.
Acknowledgements
This study was supported by the Knowledge Innovation Program of Institute of Metal Research, Chinese Academy of Sciences (Grant no. Y0N5A111A1), the Youth Innovation Promotion Association, Chinese Academy of Sciences (Grant no. Y2N5711171), and the Scientific Research Foundation for the Returned Overseas Chinese Scholars, State Education Ministry, P. R. China.
References
- D. Mohan and C. U. Pittman Jr, J. Hazard. Mater., 2007, 142, 1–53 CrossRef CAS PubMed.
- D. K. Nordstrom, Science, 2002, 296, 2143–2145 CrossRef CAS PubMed.
- A. H. Welch, D. B. Westjohn, D. R. Helsel and R. B. Wanty, Ground Water, 2000, 38, 589–604 CrossRef CAS PubMed.
- P. L. Smedley and D. G. Kinniburgh, Appl. Geochem., 2002, 17, 517–568 CrossRef CAS.
- M. Amini, K. C. Abbaspour, M. Berg, L. Winkel, S. J. Hug, E. Hoehn, H. Yang and C. A. Johnson, Environ. Sci. Technol., 2008, 42, 3669–3675 CrossRef CAS.
- T. S. Y. Choong, T. G. Chuah, Y. Robiah, F. L. G. Koay and I. Azni, Desalination, 2007, 217, 139–166 CrossRef CAS PubMed.
- M. M. Karim, Water Res., 2000, 34, 304–310 CrossRef CAS.
- C. K. Jain and I. Ali, Water Res., 2000, 34, 4304–4312 CrossRef CAS.
- S. J. McLaren and N. D. Kim, Environ. Pollut., 1995, 90, 67–73 CrossRef CAS.
- D. Chakraborti, M. M. Rahman, A. Mukherjee, M. Alauddin, M. Hassan, R. N. Dutta, S. Pati, S. C. Mukherjee, S. Roy, Q. Quamruzzman, M. Rahman, S. Morshed, T. Islam, S. Sorif, M. Selim, M. R. Islam and M. M. Hossain, J. Trace Elem. Med. Biol., 2015, 31, 237–248 CAS.
- S. J. Marlborough and V. L. Wilson, Sci. Total Environ., 2015, 520, 253–259 CrossRef CAS PubMed.
- World Health Organization, Guidelines for drinking-water quality, 3rd edn, 2008 Search PubMed.
- A. H. Smith, P. A. Lopipero, M. N. Bates and C. M. Steinmaus, Science, 2002, 296, 2145–2146 CrossRef CAS PubMed.
- R. Singh, S. Singh, P. Parihar, V. P. Singh and S. M. Prasad, Ecotoxicol. Environ. Saf., 2015, 112, 247–270 CrossRef CAS PubMed.
- M. Borho and P. Wilderer, Water Sci. Technol., 1996, 34, 25–31 CrossRef CAS.
- H. K. Hansen, P. Nunez and R. Grandon, Miner. Eng., 2006, 19, 521–524 CrossRef CAS PubMed.
- R. Baciocchi, A. Chiavola and R. Gavasci, in Leading-Edge Technology 2005-Water Treatment, ed. J. Clement and S. Parsons, 2005, vol. 5, pp. 67–74 Search PubMed.
- M. D. Ballinas, E. R. De San Miguel, M. T. D. Rodriguez, O. Silva, M. Munoz and J. De Gyves, Environ. Sci. Technol., 2004, 38, 886–891 CrossRef.
- G. S. Zhang, J. H. Qu, H. J. Liu, R. P. Liu and G. T. Li, Environ. Sci. Technol., 2007, 41, 4613–4619 CrossRef CAS.
- M. Pena, X. Meng, G. P. Korfiatis and C. Jing, Environ. Sci. Technol., 2006, 40, 1257–1262 CrossRef CAS.
- B. K. Biswas, J. Inoue, K. Inoue, K. N. Ghimire, H. Harada, K. Ohto and H. Kawakita, J. Hazard. Mater., 2008, 154, 1066–1074 CrossRef CAS PubMed.
- B. Saha, R. Bains and F. Greenwood, Sep. Sci. Technol., 2005, 40, 2909–2932 CrossRef CAS PubMed.
- Y. H. Kim, C. M. Kim, I. H. Choi, S. Rengaraj and J. H. Yi, Environ.
Sci. Technol., 2004, 38, 924–931 CrossRef CAS.
- H. Lee and W. Choi, Environ. Sci. Technol., 2002, 36, 3872–3878 CrossRef CAS.
- W. Yao, J. Wang, H. Li and Y. Lu, J. Power Sources, 2014, 247, 824–830 CrossRef CAS PubMed.
- J. Zhang, J. Du, H. Wang, J. Wang, Z. Qu and L. Jia, Mater. Lett., 2011, 65, 2565–2567 CrossRef CAS PubMed.
- S. Dharmarathna, C. K. King'ondu, L. Pahalagedara, C. H. Kuo, Y. S. Zhang and S. L. Suib, Appl. Catal., B, 2014, 147, 124–131 CrossRef CAS PubMed.
- M. A. Lemus, T. Lopez, S. Recillas, D. M. Frias, M. Montes, J. J. Delgado, M. A. Centeno and J. A. Odriozola, J. Mol. Catal. A: Chem., 2008, 281, 107–112 CrossRef PubMed.
- S. Dou, J. Solid State Electrochem., 2013, 17, 911–926 CrossRef CAS.
- X. Yang, Z. Zhiguo, W. Li, T. Caizhi, Y. Hong and Y. Shiping, Mater. Res. Bull., 2014, 57, 97–102 CrossRef CAS PubMed.
- R. Liu, H. Liu, X. Zhao, J. Qu and R. Zhang, J. Hazard. Mater., 2010, 176, 926–931 CrossRef CAS PubMed.
- B. Hu, C.-H. Chen, S. J. Frueh, L. Jin, R. Joesten and S. L. Suib, 2010, 9835–9844.
- R. Han, W. Zou, H. Li, Y. Li and J. Shi, J. Hazard. Mater., 2006, 137, 934–942 CrossRef CAS PubMed.
- B. A. Manning, S. E. Fendorf and B. Bostick, Environ. Sci. Technol., 2002, 36, 976–981 CrossRef CAS.
- J. N. Moore, J. R. Walker and T. H. Hayes, Clays Clay Miner., 1990, 38, 549–555 CAS.
- J. Pattanayak, K. Mondal, S. Mathew and S. B. Lalvani, Carbon, 2000, 38, 589–596 CrossRef CAS.
- S. Bang, M. Patel, L. Lippincott and X. Meng, Chemosphere, 2005, 60, 389–397 CrossRef CAS PubMed.
- H. Matsunaga, T. Yokoyama, R. J. Eldridge and B. A. Bolto, React. Funct. Polym., 1996, 29, 167–174 CrossRef CAS.
- X. Guo and F. Chen, Environ. Sci. Technol., 2005, 39, 6808–6818 CrossRef CAS.
- K. N. Ghimire, K. Inoue, K. Makino and T. Miyajima, Sep. Sci. Technol., 2002, 37, 2785–2799 CrossRef CAS.
- G. Pan, Y. W. Qin and X. L. Li, Chin. J. Environ. Sci., 2003, 24, 1–7 Search PubMed.
- C. C. Hu, Y. T. Wu and K. H. Chang, 2008, 2890–2894.
- M. Chigane, M. Ishikawa and M. Izaki, J. Electrochem. Soc., 2001, 148, D96 CrossRef CAS PubMed.
- M. Habuda-Stanic and M. Nujic, Environ. Sci. Pollut. Res. Int., 2015, 22, 8094–8123 CrossRef CAS PubMed.
- G. N. Manju, C. Raji and T. S. Anirudhan, Water Res., 1998, 32, 3062–3070 CrossRef CAS.
- C. Tournassat, L. Charlet, D. Bosbach and A. Manceau, Environ. Sci. Technol., 2002, 36, 493–500 CrossRef CAS.
- R. Say, N. Yilmaz and A. Denizli, Sep. Sci. Technol., 2003, 38, 2039–2053 CrossRef CAS.
- C. T. Kamala, K. H. Chu, N. S. Chary, P. K. Pandey, S. L. Ramesh, A. R. K. Sastry and K. C. Sekhar, Water Res., 2005, 39, 2815–2826 CrossRef CAS PubMed.
- F. Zhang and H. Itoh, Chemosphere, 2005, 60, 319–325 CrossRef CAS PubMed.
- X. Guo and F. Chen, Environ. Sci. Technol., 2005, 39, 6808–6818 CrossRef CAS.
- M. Chigane, M. Ishikawa and M. Izaki, J. Electrochem. Soc., 2001, 148, D96 CrossRef CAS PubMed.
- X. H. Guan, J. M. Wang and C. C. Chusuei, J. Hazard. Mater., 2008, 156, 178–185 CrossRef CAS PubMed.
Footnote |
† Electronic supplementary information (ESI) available. See DOI: 10.1039/c5ra09157h |
|
This journal is © The Royal Society of Chemistry 2015 |
Click here to see how this site uses Cookies. View our privacy policy here.