DOI:
10.1039/C5RA08550K
(Review Article)
RSC Adv., 2015,
5, 81188-81202
An insight into cochleates, a potential drug delivery system
Received
8th May 2015
, Accepted 7th September 2015
First published on 16th September 2015
Abstract
Cochleates, a type of lipid based drug delivery system, are solid particulates made up of large continuous lipid bilayer sheets rolled up in a spiral structure with little or no internal aqueous phase. These nano-sized or sub-micron sized structures are generated on fusion of negatively charged liposomes with metal cations. They are efficient in encapsulating drug molecules that are hydrophobic and hydrophilic; positively charged as well as negatively charged. The interior of a cochleate structure remains substantially intact irrespective of outer harsh environmental conditions or enzymes. Cochleate technology is applicable for administration through parenteral, topical as well as oral routes and can be formulated in liquid or powder form. Cochleates have been reported to improve the oral bioavailability; improve the safety of the drugs by decreasing side effects and increasing drug efficacy; all of which lead to enhanced patient compliance. This review article highlights the important aspects of cochleates such as their structure, properties, methods of preparation, stability, advantages, applications and current status. The information provided herein should help formulators in judiciously selecting cochleate technology for delivery of drugs.
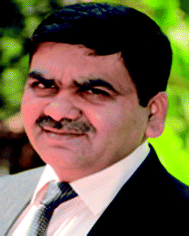 Atmaram Pawar | Atmaram Pawar is currently employed as a Professor of Pharmaceutics and Vice-Principal for the Department of Pharm D at Bharati Vidyapeeth University, Pune, Maharashtra, India. He acquired graduate, post graduate and a PhD degrees from Bharati Vidyapeeth University, Pune, Maharashtra, India. With an academic teaching experience of 25 years, he is also the author of approximately 70 peer-reviewed publications, twelve textbooks and three Indian patents. He is a referee for more than 25 pharmaceutical international journals including Langmuir, JCR, DDIP, IJP, AAPS, J. Microcap., J. Pharm. And Pharmacology, Journal of Macromolecular Science, Chem. Engg. And Communication, etc. |
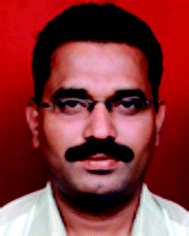 C. Bothiraja | C. Bothiraja is an Associate Professor of Pharmaceutics at Bharati Vidyapeeth University, Maharashtra, India. He acquired a PhD from Bharati Vidyapeeth University, Maharashtra, India. He has a rich experience of 15 years academic teaching. He has published 31 papers in international peer reviewed journals. He is a referee for more than fifteen pharmaceutical international journals including RSC Advances, Exp. Opi. Drug. Deliv., Colloids Surface B Interface., Food and Chemical Toxicology., J. Microencap., Pharm. Dev. Technol., Int. J. Nanomedicine and etc. He is actively involved in the development of novel drug delivery systems, specifically nanoparticles, nanocomposites, microsponges, liposomes, micelles, nanocochleates and phytopharmaceuticals. |
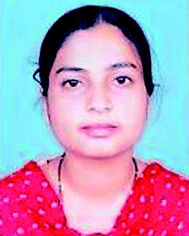 Karimunnisa Shaikh | Karimunnisa Shaikh is currently employed as an Associate Professor of Pharmaceutics at the Modern College of Pharmacy, University of Pune, Maharashtra, India. She acquired a PhD degree from Bharati Vidyapeeth University, Maharashtra, India. She was a recipient of a National Doctoral Fellowship from AICTE, India. She has pursued a graduate and post graduate course from the University of Pune, India. She has been contributing to academic studies for 10 years. She has published articles related to her expertise in several peer reviewed journals of international repute. She is actively involved in the development of novel drug delivery systems, specifically nanoparticles, liposomes, niosomes, ethosomes and phytopharmaceuticals. |
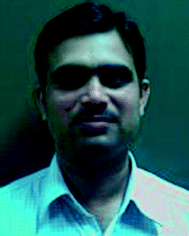 Ashwin Mali | Ashwin is currently employed as an assistant Professor of Pharmaceutics at the Bharati Vidyapeeth University, Pune, Maharashtra, India. He acquired a PhD degree from Bharati Vidyapeeth University, Maharashtra, India. With an academic teaching experience of 10 years, he is also the author of approximately 6 peer-reviewed publications and one Indian patent. His research interest involves the development of dry powder inhaler formulations for anti-asthmatic drugs. |
Introduction
In the past few decades, considerable attention has been focused on the development of new drug delivery systems (NDDS). An ideal NDDS should fulfil two basic requirements: it should deliver the drug at a rate directed as per the needs of the body over the period of treatment and it should channelize the active ingredient to the site of action. The novel drug delivery system is most suitable and approachable in developing a delivery system which improves the therapeutic efficacy of new as well as pre-existing drugs, thus providing controlled and sustained drug delivery to a specific site. Conventional dosage forms are unable to meet any of these requirements. At present, no available drug delivery system behaves ideally.1 Now-a-days vesicles as a carrier system have become the vehicle of choice in drug delivery.2
Lipid based delivery systems have attracted enormous attention by researchers to improve drug delivery. One of them, called liposome, is favorable due to its resemblance with the cell membrane. It possess various advantages such as provides selective passive targeting to tumor tissues, increases efficacy and therapeutic index of drug molecule, increases stability via encapsulation, reduces toxicity of the encapsulated agents, shows site avoidance effect, improves pharmacokinetic parameters of drug molecule (reduced elimination, increased circulation life times), imparts flexibility to couple with site specific ligands to achieve active targeting,3 help to reduce exposure of sensitive tissues to toxic drugs,4 liposomes are biocompatible, completely biodegradable, non-toxic, flexible and non-immunogenic for systemic and non-systemic administrations. However, utilization of liposomes has limitations such as cost of production is high, phospholipid may sometimes undergo oxidation & hydrolysis like reaction, short half-life, low solubility, poor mechanical stability due to leakage & fusion of the formulation, low entrapment efficiency. So the need of the hour was to develop a formulation to answer the above limitations of liposome based vesicular drug delivery systems.4 Cochleates are the vesicular system which could satisfy the present needs of the market.
History
Cochleates were discovered in 1975 by Dr Dimitrious Papahadjoupoulos and his co-workers as precipitates formed by the interaction of negatively charged phosphatidylserine and calcium.5 He named these cylindrical structures “COCHLEATE” (Fig. 1). The term “cochleate” was coined due to the resemblance of the structure to a snail with a spiral shell.6 In the late ‘80s & 90s’, cochleates were used to transport antigens and peptides for vaccine delivery. Cochleate structure is either aggregates of stacked sheets formed by trapping method or large size needles-like structures formed by the dialysis method.7 In 1999, cochleates were introduced to develop smaller, but rather more consistent particles. It was demonstrated that by using a binary phase system, such as two non-miscible hydrogels; cochleates can be formed that display a small mean particle of less than 500 nm. These cochleates were highly suitable for the encapsulation of hydrophobic drugs.8 This technology was able to answer the challenges of oral delivery of different kind of biological molecules, especially the hydrophobic ones. Cochleates differ from liposomes in having water-free interior, rod-shape & rigid stable structure (Fig. 2).5 These unique characteristics make cochleates a great platform for delivery of drugs that were having poor bioavailability.
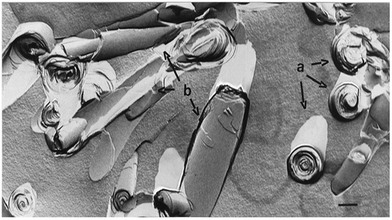 |
| Fig. 1 Freeze-fracture electron micrographs of empty cochleates prepared by the trapping film method. Arrows indicate rolled-up cochleate structures. Bar, 275 nm. Reproduced with permission from ref. 10, 2000 American Society for Microbiology. | |
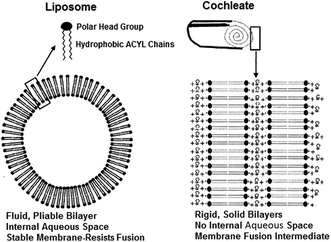 |
| Fig. 2 Structural difference between liposome and cochleate. | |
This review describes cochleates, a lipid based drug delivery technology. Through this review, the reader shall be made acquainted with this relatively more safe and effective, patient friendly drug delivery modality. The review encompasses the basics, composition, properties that offer numerous advantages, methods of preparation and applications of cochleates.9
Basics and composition of cochleates
Cochleate and nanocochleate are cigar like spiral rolls or cockle formed of negatively charged phospholipid bilayers (liposomes), which are rolled up through the interaction with multivalent counter ions (Me(metal)2+) as bridging agents between the bilayers (Fig. 3). During this process, the close approach of bilayers is dependent on dehydration of the head group of the phospholipid. They roll-up in order to minimize their interaction with water.7 They possess little or no aqueous phase. The bilayers in a cochleate are arranged very neatly at a very close repeating distance of 54 Angstrom. Small liposomes rather than the larger ones yield cochleates.11,12 Thus, they are made up of three constituents: the lipid bilayers, the cations and the agent to be delivered; on varying one or more of these constituents, various permutation combinations are possible (Table 1).
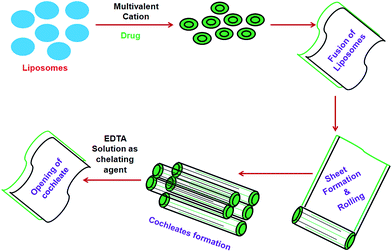 |
| Fig. 3 Cochleates formation by interaction between negatively charged liposome and cations. | |
Table 1 The various constituents of a cochleate
Cations |
Lipids |
Drug |
Zn2+, Ca2+, Mg2+, Ba2+ |
Phosphatidylserine |
Protein |
Phosphatidic acid |
Peptide |
Phosphatidylinosotol |
Polynucleotide |
Phosphotidyl glycerol |
Antiviral agent |
Phosphotidylcholine |
Anaesthetic agent |
Phosphotidylethanolamine |
Anticancer agent |
Diphosphotidylglycerol |
Immunosuppressant |
Dioleoyl phosphatidic acid |
Anti-inflammatory agent |
Distearoyl phosphatidyl serine |
Tranquilizer |
Dipalmitoylphosphatidylglycerol |
Nutritional supplement |
|
Vitamins or vasodilator |
The cochleate forming lipid essentially is a negatively charged lipid component such as phosphatidylserine (PS), dioleoyl phosphatidylserine (DOPS), phosphatidylinositol (PI), phosphatidic acid (PA) and phosphatidyl glycerol (PG) and/or a mixture of one or more of these lipids with other lipids. A study comparing purified soy PS (PSPS) to non-purified soy PS (NPSPS) concluded that PS should be present in an amount of at least 75% of the total lipid in order to allow the formation of cochleates. The other 25% phospholipids present can be selected either from the anionic group such as PA, PI, PG or phosphatidylcholine (PC).2 A binary system containing palmitoyloleoylphosphatidylcholine (POPC) and nervonoylceramide has also been reported to form cochleate-type tubular structures.13 Mixtures of anionic and zwitterionic lipids can also be used to prepare cochleates.
The presence of a divalent cation like Ca2+, Mg2+, Ba2+, Zn2+ or Fe2+ facilitates the rolling of the lipid sheets into a cochleate structure. Cation plays an important role in formation of cochleatal cylinder wherein divalent cations are preferred over the monovalent ones. The multivalent cation destabilizes the outer bilayers of the negatively charged phospholipid in the liposome due to which the bilayered structure begins to collapse. The Ca2+ ions now have easy access to inner bilayers for interaction. Eventually the liposomal sheets roll-up to form a cochleatal cylinder. It can also be said that the liposomal sheets develop hydrophobic surfaces due to interaction with metal ions and in order to minimize their interactions with water tend to roll-up into the cigar-like cochleate (Fig. 3).
Divalent cations Ca2+, Mg2+, Ba2+ and Zn2+ can be used for preparing cochleates. It has been reported that Ca2+ forms a more tightly packed, highly ordered and less hydrated structure than does Mg2+ with phospholipids. Also it is required in much lower concentration than Mg2+. It is well documented that Ca2+ plays a vital role in natural membrane fusion phenomena while other cations listed above are ineffective in most such systems. Hence it is most compatible with the body. Thus, calcium is the most suitable divalent cation reported for preparing cochleates.14
Cochleates can entrap macromolecules as well as drugs that are hydrophobic, hydrophilic, positively charged and negatively charged (Fig. 4 and 5). Cochleate technology has been used to encapsulate several moieties as proteins, peptides, anticancer agents, immunosuppressants, herbal products, vitamins, tranquilizers or nutritional supplements. The nature of the drug influences the percentage of encapsulation. Hydrophobic drugs like amphotericin B and clofazimine showed a quantitative encapsulation whereas less was seen for amphipathic molecules.15 As calcium induces dehydration of the inter bilayer domains during cochleate formation, the amount of water in this region is low, therefore, small hydrophilic molecules will not be suitable for cochleate system.16 However, doxorubicin, although a water-soluble drug, is capable of partitioning between the bilayers and the external aqueous phase through its hydrophobic regions to get entrapped into the cochleate.17
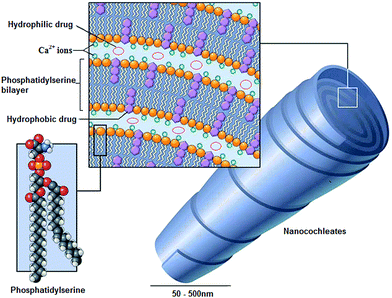 |
| Fig. 4 Schematic illustration of drug delivery via nanocochleate technology. Reproduced with permission from ref. 20, 2012 Springer. | |
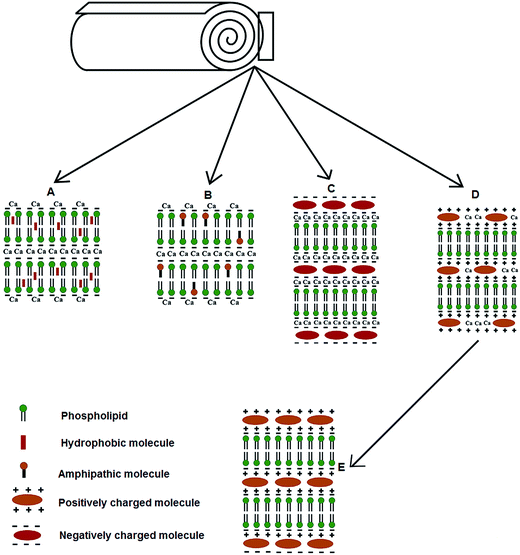 |
| Fig. 5 Cochleate can encapsulate (A) hydrophobic, (B) amphiphilic, (C) negatively charged or (D) and (E) positively charged drugs. Reproduced with permission from ref. 21, 2015 Elsevier. | |
A new type of cochleate, able to microencapsulate water-soluble cationic drugs or peptides into its inter-lipid bi-layer space, was formed through interaction between negatively charged lipids and drugs or peptides such as tobramycin, tetraaminopyridine etc. These drugs or peptides acted as the inter-bi-layer bridges instead of multi-cationic metal ions.18,19
Methods of cochleates preparation
As mentioned earlier, cochleates consist of a series of lipid bilayers, which are formed as a result of the condensation of small unilamellar negatively charged liposomes. In the presence of calcium, the negatively charged phospholipid liposomes fuse and form large sheets. A general approach to cochleate manufacture is to first prepare liposomes and then treat them with a divalent cation to change the spherical vesicle into a cochleate cylinder by one of the following methods.
Trapping method
This method involves forming phospholipid liposomes followed by the drop-wise addition of a solution of calcium chloride (Fig. 6). The liposomes can be generated either by addition of water to phospholipid powder or by adding the water phase to a phospholipid film. This leads, in general, to the formation of aggregates of cochleates and stacked sheets.11
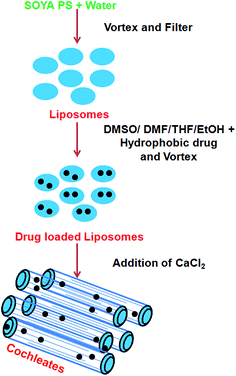 |
| Fig. 6 Cochleates preparation by trapping method. | |
Liposomes before cochleates dialysis method
This liposomes before cochleates (LC) method requires a mixture of lipid and detergent as starting materials. This double step method involves removal of the detergent from the mixture by dialysis whereby liposomes are formed and further dialysis against a solution of calcium chloride to transform liposomes into cochleates (Fig. 7). This method called the ‘LC dialysis method’ is suitable for the encapsulation of hydrophobic material or drugs containing hydrophobic region. This process has slow kinetics; the intermediate liposome is usually small and results in the formation of small cochleates. Generally, ionic detergents such as cholate salts, deoxycholate salts or nonionic detergents such as those containing polyoxyethylene or sugar head groups or heterogeneous polyoxyethylene detergents such as Tween or Brij or Triton are used. The best suitable are nonionic detergents containing sugar head groups e.g. beta-D-octyl-glucopyranoside.22
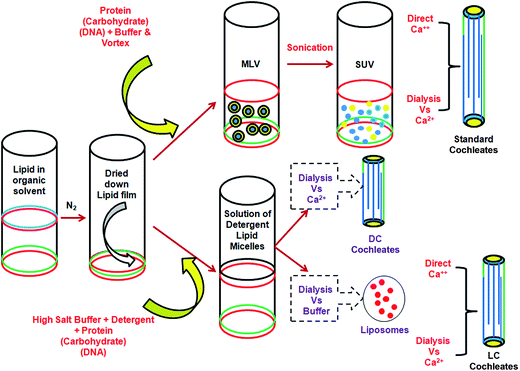 |
| Fig. 7 Cochleates preparation by liposomes before cochleates dialysis and direct cochleates dialysis method. | |
Direct cochleates dialysis method
Direct cochleates (DC) method involves the removal of detergent directly by dialysis against a calcium chloride solution: the process probably does not involve the formation of liposome intermediate (Fig. 7). The mechanism might involve a competition between the removal of detergent from the detergent/lipid/drug micelles and the condensation of bilayers by calcium. It results in needle-shaped structures, having larger diameter and length than the structures from LC dialysis method.23
Binary aqueous–aqueous emulsion system method
This method is based on the incompatibility between two-phase systems of polymers solutions, both of which are aqueous and immiscible with each other. This method does not require organic solvents. The process involves preparation of liposomes, mixing liposomal suspension into polymer solution A, addition of this polymeric liposomal suspension by injection into the polymer solution B; thus creating a two-phase aqueous system. This is followed by addition of a solution of cation salt to form small sized cochleates, washing of the cochleates and re-suspension of cochleates in physiological buffer. Further the cochleates can be lyophilized and filled into soft or hard gelatin capsules, made into tablets or other dosage forms.24
Hydrogel method
In this method small unilamellar drug loaded liposomes prepared by thin film hydration technique are added to polymer solution A (which may be PS, dextran, polyethylene glycol etc.). The dispersion of liposomal solution and polymer solution A is then added to another polymer solution B (which may be polyvinylpyrrolidone, polyvinylalcohol, Ficoll, polyvinyl methyl ether etc.). The two polymers, A and B, are immiscible with each other. Immiscibility of the polymers leads to formation of an aqueous two-phase system. The cationic cross-linking of the polymers is achieved by adding a solution of cation salt to the two-phase system, such that the cation diffuses into second polymer and then into the particles comprised of liposomes/polymer (Fig. 8). The formed cochleates are then washed to remove polymer and re-suspended into a physiological buffer.25
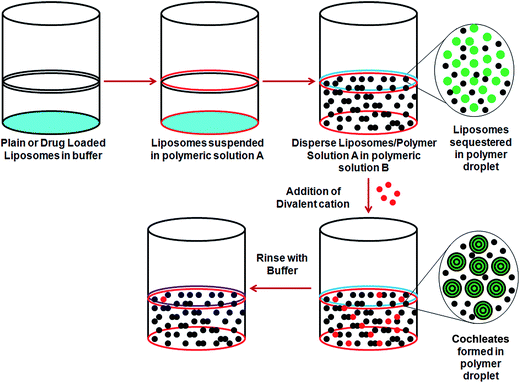 |
| Fig. 8 Cochleates preparation by hydrogel method. | |
Characterization of cochleates
The particle size is one of the most important parameter. Two techniques are used to determine the particle size distribution of which includes photon correlation spectroscopy (PCS) and electron microscopy (EM). The latter includes scanning electron microscopy (SEM), transmission electron microscopy (TEM) and freeze-fracture techniques. The size evaluation of cochleate dispersion demonstrates better results with freeze-fracturing microscopy and photon correlation spectroscopy as quantitative methods. However, electron microscopy could be adopted as an alternative option that measures individual particle for size and distribution. It is relatively less time consuming. Additionally, the freeze-fracturing of particles allows for morphological determination of their inner structure.26 To determine the encapsulation efficiency, EDTA (pH 9.5) adding in to cochleates pellets to allow the opening of cochleates into liposomes and the release of drug. Encapsulation efficiency can be calculated by using below equation.
Encapsulation efficiency% = amount of drug present in cochleates/total amount of the drug present × 100 |
The density of cochleates is determined with helium or air using a gas pycnometer. The value obtained with air and helium is much more pronounced due to the specific surface area and porosity of the structure.27 The specific surface area of freeze-dried cochleate is generally determined with the help of a sorptometer.26 The equation given below can be used to calculate specific surface area
where
A is the specific surface area,
ρ is the density and
d is the diameter of the cochleate.
The nature and intensity of the surface charge of cochleate is very important as it determines their interactions with the biological environment as well as their electrostatic interaction with bioactive compounds. The surface charge of colloidal particles in general and cochleate in particular can be determined by measuring the particle velocity in an electric field. Laser light scattering techniques such as Laser Doppler Anemometry or Velocimetry (LDA/LDV) are used as fast and high-resolution techniques for determining cochleate velocities. The surface charge of colloidal particles can also be measured as electrophoretic mobility. The charge composition critically decides the bio-distribution of drug carrying cochleate. Generally, the electrophoretic mobility of particle is determined in a phosphate saline buffer and human serum. The phosphate saline buffer (pH 7.4) reduces the absolute charge value due to ionic interaction of buffer components with the charged surface of cochleate. The zeta potential can be obtained by measuring the electrophoretic mobility by applying the Helmholtz–Smoluchowski equation.27
The surface hydrophobicity of cochleates influences the interaction of colloidal particles with the biological environment. Hydrophobicity and hydrophilicity collectively determine the bio-fate of cochleates and their contents. Hydrophobicity regulates the extent and type of hydrophobic interactions of cochleates with blood components. Several methods including hydrophobic interaction chromatography, two-phase partition, adsorption of hydrophobic fluorescent or radiolabelled probes, and contact angle measurements have been adopted to evaluate surface hydrophobicity. Recently, several sophisticated methods of surface chemistry analysis have also been used.28
Several biophysical techniques such as nuclear magnetic resonance (NMR), dynamic light scattering (DLS), negative stain and freeze fracture electron microscopy (EM), and infrared spectroscopy (IR) have been used to study this unique lipid system. Formation of cochleate structure can be identified by 31P-NMR. The addition of divalent Ca++ to phosphatidylserine precipitates the lipid. Chemically, it can be said that the phosphate group motion in such structures is severely restricted, which can be identified by the concomitant total loss of the 31P-NMR signal or a very broad 31P-NMR spectra.29,30
Laurdan spectroscopy finds application in investigating the morphology of cochleate cylinders. The membrane probe, Laurdan (6-dodecanoyl-2-dimethylamino naphthalene), is localized at the hydrophilic (polar glycerol head group)–hydrophobic (acyl chains of phospholipids) interface of a bilayer and is thus very sensitive to the polarity of its environment. In membranes, the probe displays a 50 nm emission red spectral shift during transition from a gel to liquid crystalline phase. This spectral shift is due to Laurdan's sensitivity to the dipolar relaxation phenomenon caused by reorientation of water molecules in the liquid crystalline phase. The fluorescent spectra of PS liposomes and PS cochleate cylinders labeled with the probe Laurdan were compared in the presence and absence of the encapsulated protein, FVIII. FVIII is an essential blood-clotting protein, also known as anti-hemophilic factor (AHF). At 0 °C, in the absence of CaCl2, the spectrum of Laurdan liposomes displayed a fluorescent peak maxima at ∼430 nm, which is a typical Laurdan profile for liposomes in the gel phase. When the temperature was increased, the liquid crystalline phase was formed and a second peak at 490 nm was observed suggesting that the reorganization of water molecules in the liquid crystalline phase affected the behavior of Laurdan. This probably transiently stabilized the ground state of Laurdan. The spectrum of Laurdan in cochleate cylinders too demonstrated a second peak at 490 nm; however of a low intensity and a blue shift in the band at 430 nm. This indicated that the ground state of the probe was not stabilized by dipolar interactions with the solvent. These observations again point towards the dehydrated environment of the cochleate cylinders. Also noteworthy was that the spectral properties of Laurdan incorporated in cochleate cylinders in the presence and absences of FVIII were comparable, indicating that FVIII did not substantially affect the packing of lipids in cochleate cylinders.31 Thus, Laurdan, which exhibits different properties in cochleate, gel and the liquid crystalline phases can be used to follow membrane dynamics in a PC/PS system in the presence of Ca2+ ions and to screen for the existence of non-liposomal lipid structures like cochleate cylinders in lipid systems.32
IR spectroscopy facilitates detection of ion-induced perturbations at particular phospholipid sites without the use of a probe molecule. The PS-ion interactions have been reported by several groups. Dluhy reported Ca2+ induced alterations of phosphate group vibrations.33 More specifically, dehydration of the phosphate was identified through a characteristic increase in the asymmetric phosphate group (PO2) stretching frequency from 1221 to 1238 cm−1. Casal have evaluated the conformation around the phosphodiester linkages in PS-cation systems34 whereas Choi et al. studied PS/Ca2+ systems in the presence of cholesterol.35 Such studies have also been reported for 1,2-dimyristoylphosphatidylserine (DMPS) and for DMPS/1,2-dimyristoylphosphatidylcholine (DMPC) mixtures in the presence and absence of Ca2+ ion. Another unique IR spectroscopic marker, the methyl umbrella, has been identified for cochleate cylindrical phases. A Ca2+ induced transition of a gel or liquid crystal phase to cochleate cylinder causes an increase in the frequency of the methyl umbrella from 1378 to 1386 cm−1.
This same marker was used by Flach and Mendelsohn to study Mg2+ induced fusion of saturated DMPS to produce the cochleate structures. Fusion of PS membranes to cochleates, especially Mg2+ driven, is dependent on vesicle size and on the degree of unsaturation in the lipid acyl chains. Systems containing small unilamellar vesicles fuse sufficiently whereas limited fusion is observed with large unilamellar vesicles. This lipid saturation specific Mg2+ induced fusion may be related to the molecular area at the lipid/water interface.36 Casal have reported that the binding affinity of Mg2+ to PS decreases as the molecular area increases. Also, cochleates obtained on Mg2+ induced fusion have larger dimensions probably due to incomplete dehydration leaving trapped water molecules between the bilayers. The Ca2+/PS complex is reported to be more dehydrated compared to the Mg2+ structures. A synergistic effect of Ca2+ and Mg2+ was observed as Mg2+ promotes aggregation and the close apposition of membranes which lowers the Ca2+ threshold required for fusion.
The in vitro release profile of cochleates can be determined using standard dialysis, diffusion cell or modified ultra-filtration techniques which have been recently introduced and which use phosphate buffer utilizing double chamber diffusion cells on a shake stand. A Millipore, hydrophilic, low protein-binding membrane is placed between the two chambers. The donor chamber is filled with cochleates and the receptor compartment is assayed at different time intervals for the released drug using standard procedures. The modified ultra-filtration technique is also used to determine the in vitro release behavior of cochleates. Here the cochleate is added directly into a stirred ultra-filtration cell containing buffer. At different time intervals, aliquots of the dissolution medium are filtered through the ultra-filtration membrane using <2 positive nitrogen pressure and assayed for the released drug using standard procedures.37
Properties of cochleates
The transformation of liposomes to cochleates is independent of the transition temperature of the lipid. Also, cochleates are able to resist structural changes induced due to heating above the Tm of the lipid. Cochleates can be lyophilized and stored at room temperature indefinitely at least for one year or can be stored in a divalent cation-containing buffer at 4 °C for at least two years.11,22 Cochleates can be re-transformed into liposomes by treatment with EDTA. The divalent cation responsible for close approach of bilayers is chelated by EDTA which leads to collapse of the cochleate structure into intact large liposomes (Fig. 3). However, this transformation is pH dependent. Cochleates will not convert to liposomes below pH 9.5 as the release of hydrogen ions upon the binding of calcium to the acetate groups of the chelating agent lowers the pH of the solution.17,23
Cochleates form by the calcium-induced restructuring and fusion of PS. Due to the hydrophobic nature of the surfaces of cochleates in aqueous calcium-containing solutions it was observed that the plain PS cochleates aggregate and slowly form large, needle-like structures. To prevent aggregation into larger particles, one can use excipients, such as methylcellulose, or natural products, such as casein which yield stable cochleate structures and also help in regulating the size of the particles.7,38 Cochleates can encapsulate both hydrophilic and hydrophobic drugs. Krause has demonstrated the ability to encapsulate amphotericin B, acetaminophen, acetylsalicylic acid, tobramycin, vancomycin and an anti-sense molecule.39 Table 2 show the list of drug category which can be loaded into the cochleates formulation to improve their therapeutic efficacy. Cochleate formation, in particular the size and abundance of liposomes and cochleates, also depends on the type of anionic lipid component. When compared, cardiolipin (CL) rather than phosphatidylethanolamine (PE) and PG yielded smaller and large number of cochleates as it carries more anionic charge.40
Table 2 List of drug category for cochleates formulation39a
Category |
Name of drug |
NSAIDs: non-steroidal anti-inflammatory drugs, CVS: cardiovascular system, CNS: central nervous system, BCS: biopharmaceutical classification system. |
NSAIDs |
Aspirin, diclofenac, indomethacin, ketorolac, sulindac, tolmetin, piroxicam, meloxicam, celecoxib, valdecoxib and phenylbutazone |
Anticancer |
Capecitabine, 5-fluorouracil, fludarabine, gemcitabine, doxorubicin, etoposide, docetaxel, irinotecan, paclitaxel, topotecan, vinblastine, vincristine, vinorelbine and cisplatin |
CVS |
Verapamil, diltiazem, captopril, hydralazine, mexiletine, encainide, quinidine, atenolol, amiloride |
CNS |
Chlorpromazine, haloperidol, clozapine, risperidone, amitriptyline, fluoxetine, maprotiline, benzodiazepines, buspirone and zolpidem |
BCS III drugs |
Acyclovir, amoxicillin, atropine, bisphosphonates, bidisomide, cefazolin, cetirizine, cimetidine, ciprofloxacin, cloxacillin, dicloxacillin erythromycin and famotidine |
BCS IV drugs |
Amphotericin, chlorthalidone, chlorothiazide, colistin, furosemide, hydrochlorothiazide and neomycin |
Oligomers of acylated lysines (OAKs) constitute a novel class of synthetic antimicrobial peptides that consists of alternating amino acyl chains and cationic amino acids arranged to create an optimal molecular charge and hydrophobicity for enhanced potency.41 The different length acyl chains impart a wide range of hydrophobicity (up to 60%) and the amino acids impart a high positive charge (up to 11).42 Out of the various OAKs, of particular interest to cochleate technology is the octamer C12K-7α8; widely used as clustering agent. This particular OAK has higher binding affinity to phospholipid membranes and also ability to induce the clustering of anionic lipids. The clustering leads to the lateral segregation of domains rich in anionic or zwitterionic lipids.42
In presence of clustering agent, cochleate formation depends on the gel–liquid crystalline phase-transition temperature of the anionic lipid mixture. Different mixtures of lipids when hydrated at room temperature produced differently sized cochleates depending on their transition temperature and presence or absence of clustering agents. The clustering agents, erythromycin and OAK C12K-7α8, affect cochleate formation by either synergizing or reversing the clustering. A mixture containing palmitoyl oleoyl phosphatidyl ethanolamine (POPE)
:
TOCL (1′2,2′-tetraoleoylcardiolipin) (75
:
25) exhibits good miscibility at room temperature as it is in the liquid crystalline state at this temperature. During hydration at room temperature, erythromycin (having single positive charge) interacts weakly shifting the temperature of the phase transition slightly lower. However, the highly charged (8 positive charges) OAK C12K-7α8 clusters anionic lipid, shifting the transition temperature to higher values and resulting in more number of cochleates. Addition of erythromycin together with the OAK to the lipid mixture partially reverses the clustering caused by the OAK by competing with the OAK for anionic charge. As a consequence, there are fewer and smaller cochleates and more liposomes formed. It can also be said that thus erythromycin destabilizes the OAK cochleates or it slows the rate and extent of cochleate formation.40
Lipid mixture containing DPPE
:
TOCL (75
:
25) exhibit gel–liquid crystalline phase-transition temperature at 61 °C. It is thus, in the gel state at room temperature; the lipids are immiscible; mixture is mostly dehydrated and exhibiting greater rigidity. Most of the dipalmitoyl phosphatidyl-ethanolamine (DPPE) remains segregated as a single component, although a small amount of TOCL has become miscible with DPPE. Erythromycin has little effect on the phase-transition properties in the presence of the OAK. It cannot revert the clustering caused by the OAK; it synergizes with it. It cannot destabilize the OAK cochleates to an extent as in POPE
:
TOCL. Here, it increases the extent of cochleate formation. Consequently, larger cochleates and fewer liposomes will form than in POPE
:
TOCL. Positively, this also leads to better encapsulation efficiency and in vivo efficacy of erythromycin.40
For lipid mixture containing dimyristoyl phosphatidyl-ethanolamine (DMPE)
:
TOCL (75
:
25), the gel–liquid crystalline phase-transition temperature is observed at 51 °C. Here again the lipid mixture is in the gel state when hydrated at room temperature. Erythromycin alone causes some interaction and sharpens the transition of the lipid mixture. The OAK alone causes clustering to form a new phase segregated state. When erythromycin and the OAK are added together, the phase segregation or clustering is retained, resulting in the formation of larger cochleates and few liposomes. The mixtures with DMPE
:
TOCL behave similarly to those of DPPE
:
TOCL described above, and the two systems have almost identical drug entrapment and antibacterial properties.40
The phase transition behavior of lipid mixture containing DMPE
:
DOPG (75
:
25) is similar to that of DMPE
:
TOCL, although the addition of OAK causes a less marked phase separation. Erythromycin itself has very little effect on the OAK. However, the capacity to entrap erythromycin when added together with the OAK is greatly diminished in DMPE
:
dioleoylphosphatidylglycerol (DOPG) compared to DPPE
:
TOCL or DMPE
:
TOCL. This may be a consequence of the cylinders formed with DMPE
:
DOPG being more tightly rolled up. In mixtures with DOPG, the larger excess of positive charge from the OAK completely neutralizes the charge repulsion between adjacent bilayers compared with the mixtures with TOCL. In TOCL mixtures, there is a probable residual charge that would expand the stacking, allowing for greater drug entrapment. There are also likely to be differences in the cross-sectional area of the head groups and their hydration between the mixtures with TOCL and those with DOPG; however, this remains to be investigated. Also, the compacted cochleates of DMPE
:
DOPG would fail to open easily into bilayer sheets, thus reducing drug delivery and in vivo efficacy.40
In vitro release behavior of cochleates
Similar to liposomes and other nanotechnological systems, they are capable of providing controlled and delayed release too. Cochleates act as matrix for release of drugs. An initial phase of burst release cannot be ruled out. The release of drugs from cochleates seems to be dependent upon a multitude of factors associated with the bilayer structure. However, in-depth studies analyzing drug release kinetics and developing models capable of predicting drug release are warranted. Such studies must integrate thermodynamic properties (e.g. drug ionization state, self-association, interfacial binding) and kinetic (e.g. drug species' permeability and/or kinetics of drug degradation of drug, cochleate, and/or drug–cochleate linkages) properties inherent to the drug/cochleate system.
Mechanism of cochleates drug delivery
After oral administration cochleates absorption takes place from intestine. Cochleates cross across the digestive epithelium and deliver their cargo molecule into blood vessel (Fig. 9). In case of other route except intravenous they cross across the associated cell and reach into circulation. After reaching into circulation they are delivered to targeted cell.28
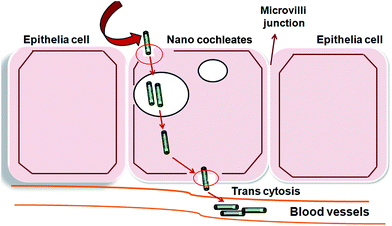 |
| Fig. 9 Absorption mechanism of cochleates. | |
Cochleates–cell interaction
The high tension at the bilayer edges of cochleates is the driving force of cochleate's interaction with the tissue membrane.43 Many natural membrane fusion processes involve calcium-induced perturbations of membranes containing negatively charged lipids. The mechanism of action of cochleates can also be described in a similar way. Hypothesis is that contact of the calcium-rich, highly ordered membrane of a cochleate with a natural membrane causes a perturbation and reordering of the cell membrane.44 Subsequently there is fusion between the outer layer of the cochleate and the cell membrane. As a result, a small amount of the encochleated material is transferred into the cytoplasm of the target cell (Fig. 10). The cochleate could then break free of the cell and be available for another fusion event, either with this or another cell.
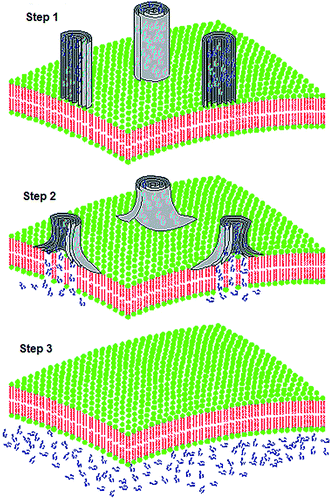 |
| Fig. 10 Cochleates fusion with tissue membrane. Reproduced with permission from ref. 28, 2008 Elsevier. | |
Cell-targeted delivery
Another hypothesis put forward is the idea of phagocytosis for cochleates' delivery. The phosphatidylserine receptors are common between the liposomal membranes of macrophages as well as those of cochleates. When in close proximity, the liposome membrane and the outer cochleate layer fuse to release the drugs into cell cytoplasm (Fig. 11). Divalent cation concentrations in vivo, in serum and in mucosal secretions are such that the cochleate structure is maintained. Hence, the majority of cochleate associated molecules are present in the inner layers of a solid, stable, impermeable structure. Once within the interior of a cell, however, the low calcium concentration results in the opening of the cochleate crystal and release of the entrapped drug.45
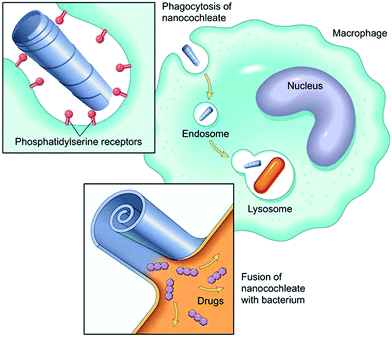 |
| Fig. 11 Drug delivery of cochleates into macrophage through fusion mechanism. Reproduced with permission from ref. 46, 2011, JP Publications. | |
Stability of cochleates
Lipids in the cochleate phase are tightly packed in the all trans configuration and are extremely motionally restricted compared to the liquid crystalline phase. This tight packing (and resulting increase in cohesive chain–chain interactions) should confer increased mechanical stability similar to the five-fold increase seen when single-component liquid crystalline membranes are frozen into the tightly packed gel phase.13 The non-aqueous cochleate structure is resistant to penetration by oxygen and consequently less susceptible to lipid oxidation. This makes them more stable than liposomes. Moreover, they are stable in acidic environment of the stomach, protecting the susceptible biologic molecules such as polypeptides or polynucleotides; a characteristic used as means for stabilizing and delivering biologic molecules. In contrast to liposomes, cochleates can be lyophilized without destroying the structure. On lyophilization, they maintain their structure, which provides the potential to be stored for long periods of time at room temperatures.47 Advantages and limitations of cochleates were given in the Table 3.
Table 3 Advantages and disadvantaged of cochleates44
Advantages |
• Cochleates are more stable than liposomes because the lipids in cochleates are less susceptible to oxidation. They maintain structure even after lyophilization, whereas liposome structures are destroyed by lyophilization |
• They exhibit efficient incorporation of biological molecules, particularly with hydrophobic moieties into the lipid bilayer of the cochleate structure |
• They have the potential for slow or timed release of the biologic molecule in vivo as cochleates slowly unwind or otherwise dissociate47 |
• They have a lipid bilayer matrix which serves as a carrier and is composed of simple lipids which are found in animal and plant cell membranes so that the lipids are non-toxic, non-immunogenic and noninflammatory48 |
• They are produced easily and safely22 |
• By the use of cochleate intravenous drugs to be administered orally (e.g. amphotericin B, a potent antifungal) |
• They improve oral bioavailability of a broad spectrum of compounds such as those with poor water solubility and protein and peptide biopharmaceuticals which have been difficult to administer (e.g. ibuprofen for arthritis)46 |
• They reduce toxicity stomach irritation and other side effects of the encapsulated drug |
• They encapsulate or entrap the subject drug within a crystal matrix rather than chemically bonding with the drug |
• They provide protection from degradation to the encapsulated drug caused by exposure to adverse environmental conditions such as sunlight, oxygen, water and temperature46 |
• Cochleates can be administered by oral or topical, subcutaneous, intradermal or intramuscular route49–59 |
![[thin space (1/6-em)]](https://www.rsc.org/images/entities/char_2009.gif) |
Disadvantages20 |
• They require specific storage condition |
• Sometimes aggregation may occur during storage; this can be avoided by the use of aggregation inhibitor |
• The cost of manufacturing is high |
Applications
Delivery of vaccines
The intrinsic properties of cochleates led to advantages in the important areas of safety, stability, efficacy, immune response targeting, combining vaccines to multiple infectious agents, alternate routes of administration (including oral and intranasal) and the generation of mucosal immunity.60–62 Cochleates containing pathogen proteins or DNA can be formulated, adjuvanted and delivered to direct the immune response to complex pathogens.63–66 Bacterial membrane proteins or the surface glycoproteins of enveloped viruses can be efficiently incorporated into the lipid bilayers of the cochleates. The gentle conditions of formulation and increased stability of the cochleates retain their biological activity and conformation. The relative amounts of the different classes and subtypes of antibodies generated in mice in response to the oral administration of influenza glycoprotein cochleates were investigated in a study. Analysis of circulating antibody revealed significant levels of flu glycoprotein-specific IgG, IgM, and IgA class, and IgGl and IgG2a subtype antibodies. Oral administration of influenza glycoprotein cochleates also induced antigen-specific salivary IgA levels. DNA plasmids and oligonucleotides can also be formulated into cochleates. Cochleates containing a plasmid that expresses the human immunodeficiency virus (HIV-l), proteins env gp I60L rev and tat, in mammalian cells, was given to mice orally or by intramuscular injection. The oral administration yielded strong splenocyte, cytolytic and proliferative responses. These cellular responses were essentially the same as those obtained by analogous intramuscular injection of DNA cochleates. Very small quantities of encochleated DNA were required to induce these responses.67,68 It was observed by Romeu et al. that proteoliposome-derived cochleate (AFCo1) acts as a potent mucosal adjuvant.69 Using AFCo1, the mucosal and systemic immune responses improved to capsular polysaccharide of Neisseria meningitidis serogroup C (PsC), a model of a thymus-independent (TI) antigen.43
Delivery of antifungal agents
AmB is a drug of choice for life threatening invasive fungal infections. However its narrow therapeutic index and adverse drug effects, specifically, nephrotoxicity, limit its use. The cochleate lipid cylinders have attracted interest as vehicles for the delivery of antifungal drugs, particularly by the oral route.50 AmB loaded cochleates have been shown to be highly effective at mediating the oral delivery of AmB that is currently available only in injectable formulations. It has been shown to be as effective as equivalent, injectable doses of the leading AmB formulation (Fungizone) in mouse models of systemic candidiasis, cryptococcal meningitis, and aspergillosis. In addition, AmB-cochleates demonstrated increased stability and substantially lower toxicity than currently existing products.41,50
Delivery of anti-inflammatory compounds
Delmarre et al. have performed tissue-culture to study the effect of aspirin and acetaminophen encapsulated nano-cochleate formulations on macrophage-derived cell line, J774.38 These aspirin and acetaminophen formulations were 5 to 10-folds more efficient than free drug at inhibiting nitric oxide synthase, one of the enzymes used by macrophage during the inflammatory response. These cochleate formulations proved highly effective drug delivery system as they hide and protect the drug molecule from degradation due to gastric atmosphere as well as protect gastro intestinal tract (GIT) from side effects of drug molecules.
Delivery of the volatile oils
Oils/extracts are sensitive to degradation by oxidation. Moreover their processing is a multistep operation which makes them costly. The cochleate technology might provide stabilization of these otherwise volatile and expensive flavor substances. Controlled release and enhanced physical and chemical stability can also be achieved by the encapsulation of flavors into cochleates.38 Flavor-cochleates can also be incorporated into consumable food preparations as flavor enhancers. Examples include flavor substances generally associated with essential oils and extracts obtained from botanical sources such as herbs, citrus, spices and seeds.
Delivery of antibacterial agent
The antibacterial agent like clofazimine, aminoglycosides, beta-lactam/beta-lactamase combinations, vancomycin, imipenem and ethionamide require long term treatment, thereby produce toxicity and drug resistance in host. Cochleates can reduce toxicity and improve their bactericidal activity. It was proved by a study on clofazimine cochleates which were prepared by trapping method and whose safety and efficacy was analysed in cell culture. It was concluded that clofazimine cochleate was 500 times less toxic than free clofazimine.11
OAKs, as discussed in an earlier section, are a family of antimicrobial agents based on the covalent attachment of acylated lysines to form oligomers. OAKs acted synergistically with several antibiotics whose resistance mechanism is mediated by efflux pumps against Gram negative multidrug-resistance strains of E. coli. In the presence of sub-MIC (minimum inhibition concentrations) of the OAK C12K-7α8, the sensitivity to several antibiotics to overcome multidrug resistance was markedly enhanced. The mechanism of this synergistic effect was shown to be through reversing the action of bacterial efflux pumps.40
Topical drug delivery
Landge et al., successfully entrapped ketoconazole in cochleates and concluded that cochleates hold promise as novel lipid carriers for dermal and transdermal delivery of drugs.54
Gene delivery
Gene therapy is an emerging field of medicine that combines a DNA plasmid and proteins with a delivery vehicle to insert into the genome of a defective cell. Gene transfer was first reported in animals in 1988. The treatment holds the potential to cure many genetic disorders such as sickle cell anemia, cystic fibrosis, hemophilia, Gaucher's disease and many other immunodeficiency diseases. DNA protein cochleates are handsome candidates for gene therapy applications. Cochleate formulations facilitate stable gene transfer in CD34+ neonatal cord blood cells. In vitro gene transfer ability of cochleate complexes in human hematopoietic cells holds latent potential to treat many genetic and infectious diseases.70 Scientists have tested for the feasibility of using rhodamine-labeled cochleates to deliver a green fluorescent protein (GFP) plasmid in cell lines and primary cells. They found that cochleates are efficiently accumulated by macrophages in both cell lines and primary cells in vitro and in vivo.71 In animal models, cochleates have been reported to be proficient in the delivery of antisense oligonucleotide for chronic lymphocytic leukemia.72
Delivery of nutrient-cochleates
Vitamin A (retinol) is sensitive to air-oxidation and is inactivated by ultraviolet light. Stability of vitamin A is enhanced by its encapsulation into the intra-bilayers of cochleates.23
Unsaturated fatty acids are biologically important in that they control the level of cholesterol in blood and are the precursors of prostaglandins. However, in the presence of oxygen, unsaturated fatty acids undergo autoxidation to produce aldehydes and ketones, which provide fishy unpleasant odor and flavor. The autoxidation of unsaturated fats can be controlled by incorporating them into the bilayers of a cochleate whereby the polyunsaturated fatty acids (PUFA) are placed in close contact with oxygen-stable saturated fatty esters of the phosphatide.22,23
Hemophilia A, a life-threatening bleeding disorder, is caused by deficiency of factor VIII (FVIII). Replacement therapy using recombinant FVIII (rFVIII) is the first line therapy for hemophilia A. However, 15–30% of patients develop neutralizing antibody, mainly against the C2, A3 and A2 domains. It has been reported that PS-FVIII complex reduced total and neutralizing anti-rFVIII antibody titers in hemophilia A murine models. Approximately 75% of the protein was associated with cochleate cylinders. Sandwich ELISA, acryl amide quenching and enzymatic digestion studies established that rFVIII was shielded from the bulk aqueous phase by the lipidic structures, possibly leading to improved in vivo stability. Freeze–thawing and rate-limiting diffusion studies revealed that small cochleate cylinders with a particle size of 500 nm or less could be generated. The release kinetics and in vivo experiments suggested that there is slow and sustained release of FVIII from the complex upon systemic exposure. In vivo studies using tail clip method indicated that FVIII–cochleate complex is effective and protects hemophilic mice from bleeding.31
Delivery of insulin
Magnetocochleate is a fused lipid microstructure embedded with ferrite nanoparticles. Dwivedi et al., used to encapsulate protein macromolecules like insulin.73 By making use of the lipid phase transition from the fluidic lamellar phase to the gel phase at pH 2, these fused microstructures protect insulin from the action of enzymes and from pH changes, which is necessary to maintain its bioactivity. The magnetocochleate obtained by tuning the hydrophilic head group hydration of phosphatidylserine in the presence of ferrite encapsulates a larger amount of insulin. Freeze fracture transmission electron microscopy revealed the gel-like phase of fused lipid bilayers and the presence of ferrite in magnetocochleate. Confocal micro-Raman, high performance liquid chromatography (HPLC) studies confirmed the presence of ferrite and insulin within the lipid microstructures. Thus magnetocochleates have potential to deliver sensitive molecules such as insulin orally. In vivo subcutaneous activity was studied in a rats model and the positive result obtained there signifies the promising potential of magnetocochleates in subcutaneous delivery of macromolecules.
Delivery of fisetin
Fisetin (3,3′,4′,7-tetrahydroxy flavone), one of the major flavonoids, is widely found in fruits, vegetables, nuts and wine such as strawberry, apple, persimmon, grape, kiwi fruit, onion and cucumber. It has been shown to exert antioxidant, anti-inflammatory, anti-angiogenic, anti-invasive, hypoglycemic and anti-convulsant activity. The great therapeutic interest of fisetin is however marred by its low oral bioavailability (44.1%) probably due to its high lipophilicity (log
P 3.2) and low (10.45 μg mL−1) aqueous solubility. Moreover, the fisetin molecule bearing 4 hydroxyl substituents, including a catechol on the phenyl B ring, is extensively metabolized in vivo. The above factors lead to high/frequent dosing to achieve optimum therapeutic efficacy which often causes severe side effects. Bothiraja et al., developed fisetin-loaded nanocochleates exhibiting higher drug loading, sustained release and improved in vitro anticancer potency of fisetin were developed.74 The developed nanocochleates displayed enhanced systemic bioavailability and low tissue distribution. The nanocochleates technology could therefore advantageously be employed to improve the antiangiogenic and anticancer activities of fisetin as well as other poorly water soluble flavonoids.
Delivery of paclitaxel
Paclitaxel (PTX) is an antineoplastic agent with a proven activity against a number of tumors.75 The currently marketed forms of PTX for intravenous formulations (Taxol, Paxene) contain a mixture of Cremophor EL (poly-oxyethylated castor oil derivatives) and ethanol (1
:
1, v/v) as a solvent, known to induce severe side effects including neurotoxicity, nephrotoxicity, vasodilatation, labored breathing, lethargy, hypotension and hypersensitivity.76 Premedication with corticosteroids and antihistamines are needed to reduce intensity and incidence of serious hypersensitivity.77 In this context, the oral administration of PTX may be alternative in order to improve the patient's compliance which would decrease the cost of the therapy. Nevertheless, oral treatment with PTX is severely hampered because of its low bioavailability (7%) that is attributed to its high lipophilicity (log
P 3.5), poor aqueous solubility (12.31 mg mL−1), efflux and affinity for intestinal and liver cytochrome P450 metabolic enzymes.78
Pawar et al., developed and investigated nanocochleates composed of phosphatidylcholine (PC), cholesterol and calcium ions as an effective oral nanocarrier for PTX.79 The developed nanocochleates exhibited higher encapsulation efficiency and sustained release of PTX. Moreover, the PTXNC demonstrated higher in vitro anticancer activity in lung adenocarcinoma cancer A-549, ovarian cancer OVCAR-3 and human breast cancer MCF-7 cells and displayed enhanced oral bioavailability with low tissue distribution than its free form, which may bring about reduction in dose as well as cost and increase patient compliance. These nanocochleates could therefore advantageously be employed to improve anticancer activity of PTX and an alternative to the present intravenous administration. Table 4, shows that the bioavailability and therapeutic efficacy of bioactive from cochleates formulation and other delivery systems.
Table 4 Bioavailability and therapeutic efficacy of bioactive from cochleates and other delivery systems: a comparison49,54,74,80–84
Cochleates delivery system |
Other delivery system |
Fisetin-loaded nanocochleates demonstrated 141-fold increase in fisetin oral bioavailability |
Fisetin-loaded nanoemulsion demonstrated 24-fold increase in fisetin oral bioavailability |
Ketoconazole loaded cochleates showed 25% improved antifungal activity |
Ketoconazole loaded niosomal gel showed 10% antifungal activity |
Oral cochleate-amphotericin B demonstrated potent efficacy against systemic candidiasis |
Oral liposomal-amphotericin B demonstrated 10 fold least effective than cochleates |
Paclitaxel loaded nanocochleates showed significantly 19 fold, improved oral bioavailability |
Paclitaxel loaded-lipid based nanoparticles, layersome and polymeric micelles improved 6, 4 and 15 fold oral bioavailability |
Commercial status
BioDelivery Sciences International, Inc. (BDSI) is a biopharmaceutical company engaged in developing clinically significant formulations of proven therapeutics. The Company's patented Bioral® (cochleate) drug delivery technology developed in collaboration with The University of Medicine and Dentistry of New Jersey and the Albany Medical College encochleates selected drugs or therapeutics. The Company's lead Bioral® formulation is an encochleated version of AmB (which the Company refers to as CAMB) an anti-fungal treatment for treating systemic fungal infections. This Bioral® formulation of Am B would have the potential for oral delivery. Following the completion of preclinical testing in 2006, BDSI submitted an IND to the FDA for CAMB, in December 2006, which was accepted by the FDA.85 The products under development using Bioral® technology are
(a) Bioral® amphotericin B (fungal infections, under preclinical trials, inhouse commercialization).
(b) Bioral® non-steroidal anti-inflammatory compounds like aspirin, ibuprofen, naproxen, acetaminophen and COX-2 inhibitors. (pain/inflammation, under preclinical trials, available for licensing).
(c) Bioral® paclitaxel (oncology, under preclinical trials, available for licensing).
(d) Bioral® siRNA therapeutics (infectious diseases/cancer, under preclinical trials, available for licensing).
Conclusion
Cochleates are lipidic structures formed by fusion of negatively charged liposomes with metal cation and/or multivalent drug molecule which act as a bridging agent for the formation of cochleate cylinders. These unique cockle shaped structures are formulated from various negatively charged lipids and can be characterized using various techniques such as P-NMR, IR spectroscopy etc. Cochleate are devoid of water and can encapsulate both hydrophilic and hydrophobic drugs and a wide range of biologically important molecules. Encochleation is known to improve an end product by enhancing the quality of the formulation, increasing processing and shelf-life stability, enhancing bioavailability, reducing toxicity and increasing efficacy. Cochleates are promising for oral, transdermal and dermal delivery. Research efforts in this segment have led to commercialization of cochleates. Many more drug candidates can be encapsulated into cochleates for their effective delivery.
Future applications
Cochleate technology can be applied to delivery of photolabile drugs, other drugs degraded by oxidation and phytoconstituents. Another sphere in cochleate technology that needs attention is use of anionic marine lipids. Nevertheless, they can be investigated for alternative routes of administration such as nasal, transdermal and vaginal. Another possibility that remains to be investigated is active targeting of potential drugs via use of targeting ligands.
Conflict of interest
The authors declare that they have no conflicts of interest concerning this article.
Acknowledgements
The authors are thankful to All India Council for Technical Education, New Delhi, India for providing financial support in form of Research Promotional Scheme fund to carry out this project.
Notes and references
- V. Li, J. Robinson and V. Lee, Controlled drug delivery: Fundamentals and applications, 1987, vol. 29, p. 3 Search PubMed
. - N. K. Dahiya, R. Rao and S. Nanda, J. Pharm. Biomed. Sci., 2011, 5, 1 Search PubMed
. - L. Stryer, Biochemistry, W. Freeman and Co, San Francisco, 1981, vol. 24, p. 7 Search PubMed
. - H. Anwekar, S. Patel and A. Singhai, Int. J. Pharm. Life Sci., 2011, 2, 945 CAS
. - D. Papahadjopoulos and J. Wilschut, Nature, 1979, 281, 690 CrossRef PubMed
. - L. Zarif, Cochleates as nanoparticular drug carriers, in Nanoparticulates as drug carriers, ed. V. P. Torchilin, Imperial College Press, London, 2006, pp. 349–366 Search PubMed
. - L. Zarif, J. R. Graybill, D. Perlin and R. J. Mannino, J. Liposome Res., 2000, 10, 523 CrossRef CAS
. - L. Zarif, in methods in enzymology, Liposomes, Part E, ed. N. Duzgunes, Academic Press, UK, 2005, vol. 391, p. 314 Search PubMed
. - P. Papahadjopoulos, Cell Surf. Rev., 1978, 5, 765 Search PubMed
. - L. Zarif, J. R. Graybill and D. Perlin, Antimicrob. Agents Chemother., 2000, 44, 1463 CrossRef CAS
. - L. Zarif, J. Controlled Release, 2002, 81, 7 CrossRef CAS
. - R. J. Mannino, S. Gould-fogerite, L. S. Krause-Elsmore, D. Delmarre and L. Ruying, US Pat., Number 0237814 A1, 2007
. - S. N. Pinto, L. C. Silva, F. M. Rodrigo and M. Prieto, Biophys. J., 2008, 95, 2867 CrossRef CAS PubMed
. - C. Newton, W. Pangborn, S. Nir and D. Papahadjopoulos, Biochim. Biophys. Acta, 1978, 506, 281 CrossRef CAS
. - C. Popescu, S. Franzblau and L. Zarif, 41st ICAAC, in Chicago, IL 2001.
- A. Portis, C. Newton, W. Pangborn and P. Papahadjopoulos, Biochem. J., 1979, 18, 780 CrossRef CAS
. - R. J. Mannino, S. Gould-Fogerite, S. L. Krause-Elsmore and D. L. Delmarre, Patent WO/2004/091578, 2004
. - T. Jin, Patent application WO/2004/012709, 2004
. - T. Jin, US Pat., Number US2004/0092727A1, 2004
. - H. Kaya-Celiker and K. Mallikarjunan, Food Eng. Rev., 2012, 2, 114 CrossRef
. - J. Li, X. Wang, T. Zhang, C. Wang, Z. Huang, X. Luo and Y. Deng, Asian J. Pharm. Sci., 2015, 10, 81 CrossRef PubMed
. - S. Gould-Fogerite and R. J. Mannino, US Pat., Number 5,643,574, 1997
. - S. Gould-Fogerite and R. J. Mannino, US Pat., Number 5,994,318, 1999
. - T. Jin, L. Zarif and R. Mannino, US Pat., Number 6,153,217, 2000
. - L. Zarif, T. Jin, I. Seqarra and R. J. Mannino, US Pat., Number 6,592,894, 2003
. - M. S. Uwais, W. Amyf, J. B. Tuo and Z. Hua, Int. J. Pharm., 2008, 363, 118 CrossRef PubMed
. - A. M. Villa, E. Caporizzo and A. Papagin, Eur. J. Cancer, 2005, 41, 1453 CrossRef CAS PubMed
. - U. M. Syed, A. F. Woo, F. Plakogiannis, T. Jin and H. Zhu, Int. J. Pharm., 2008, 363, 118 CrossRef CAS PubMed
. - P. R. Cullis and A. J. Verkleij, Biochim. Biophys. Acta, Biomembr., 1979, 552, 546 CrossRef CAS
. - M. Roux and M. Bloomt, Biophys. J., 1991, 60, 38 CrossRef CAS
. - R. D. Miclea, P. R. Varma, A. Aaron Peng and S. V. Balu-Iyer, Biochim. Biophys. Acta, 2007, 1768, 2890 CrossRef CAS PubMed
. - K. Ramani and S. V. Balasubramanian, Biochim. Biophys. Acta, 2003, 1618, 67 CrossRef CAS PubMed
. - R. A. Dluhy, D. G. Cameron, H. H. Mantsch and R. Mendelsohn, Biochemistry, 1983, 22, 6318 CrossRef CAS
. - R. F. Carol and M. Richard, Biophys. J., 1993, 64, 1113 CrossRef
. - S. Choi, W. Ware, S. R. Lauterbach and W. M. Phillips, Biochemistry, 1991, 30, 8563 CrossRef CAS
. - C. R. Flach and R. Mendelsohn, Biophys. J., 1993, 64, 1113 CrossRef CAS
. - L. Zarif and D. Perlin, Drug Delivery Technol., 2002, 4, 34 Search PubMed
. - D. Delmarre, R. Lu, N. Tatton, S. Krause-Elsmore, S. Gould-Fogerite and R. Mannino, Bioral®™ formulation kit, BDSI, 2004, vol. 4, p. 64 Search PubMed
. - S. Krause, Dissertation, graduate school of biosciences, University of medicine and dentistry of New Jersey, 2003
. - H. Sarig, D. Ohana, R. F. Epand, A. Mor and R. M. Epand, FASEB J., 2011, 25, 3336 CrossRef CAS PubMed
. - M. O. Makobongo, T. Kovachi, H. Gancz, A. Mor and D. S. Merrell, Antimicrob. Agents Chemother., 2009, 53, 4231 CrossRef CAS PubMed
. - S. A. Rotem and A. Mor, Biochim. Biophys. Acta, 2009, 1788, 1582 CrossRef CAS PubMed
. - R. Mannino, S. Gold-Foserite, M. T. Kheiri, F. Zhang and Z. Wang, Adv. Drug Delivery, 1998, 32, 237 Search PubMed
. - E. C. Unger, US Pat., Number 6403056, 2002
. - Aquariusbio, Matinas Biopharm, 2013, http://www.aquariusbio.com/wp-content/uploads/2013/04/Biotech-2012-Innovation-Corridor.pdf.
- V. Panwar, V. Mahajan, A. S. Panwar, G. N. Darwhekar and D. K. Jain, Int. J. Pharma Bio Sci., 2011, 1, 31 CAS
. - D. David, S. Dould-Fogerite, S. Krause-Elsmore, L. Lu, M. Ruying and J. Raphael, Patent WO 2004091578, 2005
. - C. Zayas, Vaccine, 2006, 24, 94 CrossRef PubMed
. - R. Santangelo, P. Paderu, G. Delmas, Z. W. Chen, R. Mannino, L. Zarif and D. S. Perlin, Antimicrob. Agents Chemother., 2356, 200, 44 Search PubMed
. - T. J. Walsh, M. A. Viviani, E. Arathoon, C. Chiou, M. Ghannoum, A. H. Groll and F. C. Odds, Med. Mycol., 2000, 38, 335 CrossRef CAS PubMed
. - J. R. Graybill, L. K. Najvar, R. Bocanegra, A. Scolpino, R. J. Mannino and L. Zarif, 39th International conference on antimicrobial agents and chemotherapy, American Society for Microbiology, San Francisco, CA, Washington DC, Abstract, 1999, vol. 2009, p. 26 Search PubMed
. - I. Seagram, T. Jin, D. A. Hyra, R. J. Mannino and L. Zarif, 39th International conference on antimicrobial agents and chemotherapy, American Society for Microbiology, San Francisco, CA, Washington DC, Abstract, 1999, vol. 1940, p. 26 Search PubMed
. - S. Gould-Fogerite, J. Mazurkiewicz, J. Lehman, J. K. Raska, K. Voelkerding and R. J. Mannino, Gene, 1999, 84, 429 CrossRef
. - A. Landge, A. Pawar and K. Shaikh, Int. J. Pharm. Pharm. Sci., 2013, 5, 314 CAS
. - G. Delmas, S. Park and Z. W. Chen, Antimicrob. Agents Chemother., 2002, 46, 2704 CrossRef CAS
. - L. Zarif, J. R. Graybill and D. Perlin, Antimicrob. Agents Chemother., 2000, 44, 1463 CrossRef CAS
. - I. Segarra, D. A. Movshin and L. Zarif, J. Pharm. Sci., 2002, 91, 1827 CrossRef CAS PubMed
. - A. M. Sesana, R. M. Rocha, S. A. Vinhas, C. G. Morais, R. Dietze and E. M. Lemos, In vitro activity of Amphotericin B cochleates against Leishmania chagasi, Memorias do Instituto Oswaldo Cruz, Impresso, 2011, vol. 106, p. 251 Search PubMed
. - R. Dietze, E. P. Milan, J. D. Berman, M. Grogl, A. Falqueto, T. F. Feitosa, K. G. Luz, F. A. Suassuna, L. A. Marinho and G. Ksionski, Clin. Infect. Dis., 1993, 17, 981 CrossRef CAS PubMed
. - S. Gould-Fogerite, M. Kheiri, F. Zhang and R. J. Mannino, J. Liposome Res., 2005, 10, 339 CrossRef
. - S. Gould-Fogerite and R. J. Mannino, vaccine adjuvants: Preparation methods and research protocols, ed. D. T. O'Hagan, Humana Press, Totowa, New Jersey 07512, 2000, vol. 2, p. 179 Search PubMed
. - R. J. Mannino and G. F. Susan, US Pat., Number 5,840,707, 1998
. - S. Gould-Fogerite and R. J. Mannino, J. Liposome Res., 1996, 6, 357 CrossRef
. - R. J. Mannino and S. Gould-Fogerite, in New Generation Vaccines, ed. M. M. Levine, G. C. Woodrow, J. B. Kaper and G. S. Cobon, Marcel Dekker, Inc., New York, NY, 1997, vol. 2, p. 229 Search PubMed
. - S. Gould-Fogerite, M. T. Kheiri, F. Zhang, Z. Wang, A. Scolpino, E. Feketeova, M. Canki and R. J. Mannino, Adv. Drug Delivery Rev., 1998, 32, 273 CrossRef CAS
. - S. M. Michalek, D. T. Hagen, S. Gould-Fogerite, A. D. M. E. Osterhaus and G. F. Kimtnelzwaan, Antigen delivery systems: Nonliving microparticles, liposomes, cochleates and ISCOMS, in Mucosal ImmunologyNew Generation Vaccines, ed. P. Ogra, Marcel Dekker, New York, NY, 1998, vol. 2, p. 759 Search PubMed
. - D. Margolis, S. Gould-Fogerite and R. J. Mannino, US Pat., Number 6,340,591, 1998
. - G. Susan, Adv. Drug Delivery Rev., 1998, 32, 273 CrossRef
. - R. Acevedo, B. Romeu, C. Zayas, E. Gonzalez, M. Lastre, C. Judith del, A. Mullen, V. A. Ferro and O. Perez, Vaccimonitor, 2012, 21, 1 Search PubMed
. - Drug development and delivery, http://www.drugdeliverytech.com.
- B. Gibson and A. M. Duffy, Anticancer Res., 2004, 24, 483 CAS
. - G. Parker, B. Peng, M. He, S. Gould-Fogerite, C. Chou and E. S. Raveche, in Methods in Enzymology, ed. M. I. Phillips, Academic Press, NY, USA, 1999, p. 411 Search PubMed
. - N. Dwivedi, M. A. Arunagirinathan, S. Sharma and J. Bellare, J. Phys. Chem. B, 2009, 113, 13782 CrossRef CAS PubMed
. - C. Bothiraja, B. Y. Dilip, K. S. Shaikh, A. P. Pawar and U. H. Thorat, Expert Opin. Drug Delivery, 2014, 11, 17 CrossRef CAS PubMed
. - C. Bothiraja, U. H. Thorat, A. P. Pawar and K. S. Shaikh, Mater. Technol., 2014, 29(B2), B120 CrossRef CAS PubMed
. - R. B. Weiss, R. C. Donehower, P. H. Wiernik, T. Ohnuma, R. J. Gralla and D. L. Trump, J. Clin. Oncol., 1990, 8, 1263 CAS
. - P. E. Kintzel, Ann. Pharmacother., 2001, 35, 1114 CAS
. - M. Agueros, L. Ruiz-Gaton, C. Vauthier, K. Bouchemal, S. Espuelas and G. Ponchel, Eur. J. Pharm. Sci., 2009, 38, 405 CrossRef CAS PubMed
. - A. P. Pawar, D. Vinugala and C. Bothiraja, Biomed. Pharmacother., 2014 DOI:10.1016/j.biopha.2014.11.014
. - H. Ragelle, S. Crauste-Manciet, J. Seguin, D. Brossard, D. Scherman, P. Arnaud and G. G. Chabot, Int. J. Pharm., 2012, 427, 452 CrossRef CAS PubMed
. - S. B. Shirsand, M. S. Para, D. Nagendrakumar, K. M. Kanani and D. Keerthy, Int. J. Pharm. Invest., 2012, 2, 201 CrossRef CAS PubMed
. - D. Pandita, A. Ahuja, V. Lather, B. Benjamin, T. Dutta and T. Velpandian, AAPS PharmSciTech, 2011, 12, 712 CrossRef CAS PubMed
. - S. Jain, D. Kumar, N. K. Swarnakar and K. Thanki, Biomaterials, 2012, 33, 6758 CrossRef CAS PubMed
. - F. Z. Dahmani, H. Yang, J. Zhou, J. Yao, T. Zhang and Q. Zhang, Eur. J. Pharm. Sci., 2012, 47, 179 CrossRef CAS PubMed
. - N. C. Raleigh, BioDelivery Sciences International, http://www.understandingnano.com/clinicalstudy-bioral-Amphotericin-B.html, accessed 5 February 2009 Search PubMed.
|
This journal is © The Royal Society of Chemistry 2015 |