DOI:
10.1039/C5RA08086J
(Paper)
RSC Adv., 2015,
5, 51875-51882
Exploitation of a new Schiff-base ligand for boric acid fluorescent sensing in aqueous medium with bio-imaging studies in a living plant system†
Received
2nd May 2015
, Accepted 5th June 2015
First published on 5th June 2015
Abstract
A hydrolysis resistant Schiff-base ligand (L1) consisting of an aromatic aldimine moiety appended to a triazole precursor along with phenolic–OH has been synthesized which acts as a fluorescence probe and exhibits rare selective sensing ability for boric acid (BA) in aqueous buffer medium at pH 7.3 by forming a 1
:
1 chelate complex. Reasons behind the complexation and subsequent fluorescence enhancement are well established using experimental as well as theoretical evidence. Bio-imaging studies are done using Arabidopsis Thaliana as the model plant system.
Introduction
Boric acid (BA) is a useful mineral with insecticidal, fungicidal and herbicidal properties.1 It plays a pivotal role in plant physiology as a boron (B) transporter.2 B is one of the essential micronutrients in plant nutrition, particularly for vascular plants.3 It helps to stabilize the cell membrane,4 accelerates lignin synthesis5 and controls the biosynthesis of phenolic precursors.6 Symptoms of B deficiency occur mainly in growing or expanding organs in the plant body.7 Under B-deficient conditions, leaf enlargement, root elongation, flower development and pollen tube formation are affected.8 B deficiency also results in higher peroxidase and polyphenol oxidase activities.5 Absorption of B from soil mainly occurs in the form of BA present in soil, since B exists mostly as undissociated BA (pKa = 9.25 at 25 °C) in soil at physiological pH,9 although the mechanism of BA intake remains unresolved and its function in cellular and molecular level needs to be investigated in detail.
To understand the details of the BA absorption from soil and the mechanistic aspects of its action in the plant system, suitably designed BA sensing probe is likely to provide valuable information. Till date BA fluorescent sensors are extremely rare and restricted mainly to decades old Alizarin Red S without any knowledge of its molecular level details and lacking bio-imaging facility.10 Another claim by Wang et al. for BA sensor11 exploiting B–N interaction followed by cyclic boronate formation was later contested.12 However, their efforts to show independent (without using saccharide binding13) B–N interaction in protic solvent have prompted us to search such precursors. The existence of B–N bond is primarily observed either in solid state or in aprotic condition13 and often faces solvolysis or solvent insertion to B center that precludes the chance of B–N bond formation in protic media.14 On the contrary, it has been observed that Azomethine-H (8-hydroxy-1-(salicylideneamino)-3,6-naphthalenedisulfonic acid), generally used for spectrophotometric determination of B or BA in aqueous media,15 forms 1
:
1 chelating complex with BA. The aromatic aldimine moiety along with the syn-transposing two phenolic-OH groups facilitate the chelating, where the B–N coordination was later established.16 However, imino-bond in Azomethine-H undergoes hydrolysis16 as often observed for Schiff bases in water.17
Being inspired, we have judiciously chosen the Schiff base ligand (L1) consisting of an aromatic aldimine moiety appended to an electron deficient triazole precursor18 along with the adjacent phenolic –OH group. Unlike the general trend the Schiff base ligand L1 is reluctant to hydrolysis and acts as a BA fluorescent sensor in 100% aqueous medium. Interestingly, B–N quasi-coordination and consequently chelating ring formation in aqueous medium plays the cardinal role to lift the intra-ligand excited state electron or charge transfer (ESCT) possibility to exhibit BA induced exclusive fluorescence enhancement (Scheme 1). The observation equally holds good for phenyl boronic acid (PBA), which has indeed immense applications in synthetic chemistry as well as in industry19 and one could claim the ligand as a PBA sensor also. Hence, in many cases PBA induced comparative data are presented along with BA for broad spectrum readers' interest. However, detail studies are confined mainly on BA owing to its more significance in plant physiology. Probably this is for the first time its competence in bio-imaging in plants has been explored by taking Arabidopsis Thaliana as the model plant system.
Experimental
General experimental methods
Unless otherwise mentioned, chemicals and solvents of purest grade were purchased from Sigma-Aldrich Chemicals Private Limited and were used without further purification. Mili-Q Milipore®18.2 MΩ cm−1 water was used for preparation of buffer medium and other analytical studies. 10 mM HEPES buffer concentration was maintained throughout for in vitro UV-Vis absorption and fluorescence studies. The specific pH for the buffer was adjusted by gradually adding required amount of ∼1.0 M NaOH solution and subsequently checking the pH with Systronics digital pH meter (Model no. 335). For spectroscopic studies, required amount of different analytes were mixed in a 10 mM HEPES for certain pH and if required final pH of the measuring solution was further adjusted by adding either 0.1 M NaOH or 0.1 M HCl before measurements. The ESI-MS were performed with a Waters Qtof Micro YA263 mass spectrometer in the positive mode. 1H and 13C NMR spectra were performed in DMSO-d6 with a Bruker 300 MHz NMR Spectrophotometer using tetramethylsilane (δ = 0) as an internal standard. 11B NMR spectra were recorded on a Bruker 500 MHz NMR Spectrophotometer at a resonance frequency of 160 MHz and the chemical shifts were determined with reference to a 5 mM BA solution as an external standard. Elemental analyses were performed by using Perkin-Elmer 240-elemental analyzer. IR spectra were recorded from KBr pellets with a Perkin-Elmer Spectrum-2 spectrophotometer. The bio-imaging were carried out with a Leica DM 3000 Fluorescence microscope (excitation ca. 370 nm) with a Leica DFC450 C Camera (Leica Fire Wire V1.39.0).
Synthesis of Schiff-base ligand (L1)
2-Hydroxy-3-(hydroxymethyl)-5-methylbenzaldehyde (HHMB) was synthesized according to our published procedure.20 For ligand (L1) synthesis, to a methanolic solution of HHMB (0.166 g, 1 mmol), 4-amino-4H-1,2,4-triazole (Tz) (0.084 g, 1 mmol) was added drop-wise with constant stirring and 2 drops of AcOH were further added to it (Scheme 1). The mixture was refluxed for 2 h and then filtered. The filtrate was then evaporated under reduced pressure to get the crude product. It was purified by column chromatography followed by rotary evaporation to obtain the solid product and dried over P2O5 under vacuum. It was further recrystallized from toluene–chloroform mixed solvent (7
:
3, v/v) to afford yellow colored crystals. Yield: 64.8% with respect to HHMB. m.p. 187 °C. Anal. cald for C11H12N4O2: C, 56.89; H, 5.21; N, 24.12; obsd C, 56.99; H, 5.25; N, 24.28; %. Selected IR in cm−1 (KBr): 3200 (b), 3095 (w), 2924 (w), 2880 (w), 1620 (s), 1595 (s), 1510 (s). ESI-MS+ for L1 in aqueous HEPES buffer pH 7.3: m/z calcd for [L1 + H]+: 233.2420 found: 233.0276 (see details mass spectra in ESI, Fig. S5†). 1H NMR (DMSO-d6, 300 MHz): δ = 2.27 (s, 3H, ArCH3), 4.56 (d, 2H, –CH2), 5.20 (t, 1H, alcoholic–OH, J = 2), 7.32 (s, 1H, ArH), 7.36 (s, 1H, ArH), 9.15 (s, 3H, imine-H and triazole ring-H), 9.93 (s, 1H, ArOH) ppm. 13C NMR (DMSO-d6, 75 MHz): 20.54, 58.44, 117.07, 128.72, 129.19, 130.73, 132.93, 139.24, 153.43, 158.79 ppm (see details NMR spectra in ESI, Fig. S6†).
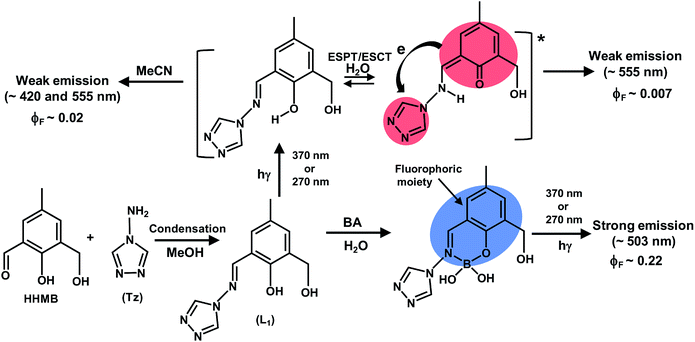 |
| Scheme 1 Synthesis of L1 and its BA induced complexation with proposed sensing mechanism for L1. | |
UV-Vis absorption and fluorescence studies
All absorption and fluorescence measurements were carried out in 10 mM HEPES, pH 7.3 at 20 °C with a UV-2450 spectrophotometer (Shimadzu, Japan) and Perkin Elmer LS-55 spectro fluorimeter (Perkin Elmer, USA), respectively. Quartz cells with 1 cm path-lengths were used for absorption and fluorescence measurements, respectively. Fluorescence measurements were performed by 370 or 270 nm excitation. The contribution of second order scattering at ∼540 nm in the 550 nm fluorescence due to 270 nm excitation was eliminated by subtracting the background spectra for the measuring buffer containing different analytes without L1 from actual solution for measurement. Fluorescence transient decays were monitored with time-correlated-single-photon-counting (TCSPC) techniques. Two separate nanosecond diode with excitation 370 and 280 nm respectively (nano-LED; IBH, U. K.) were used as the light source and a TBX4 detection module (IBH, U. K.) coupled with a special Hamamastu photomultiplier tube (PMT) was used for the detection of the fluorescence decays. The solutions for the analysis were passed through a 0.45 μm filter (Millex, Millipore) before the measurement. All measurements were repeated at least three times to check the reproducibility.
Determination of fluorescence quantum yield (ϕF) and detection limit
The fluorescence quantum yield of L1 as well as L1 + BA or L1 + PBA were determined by adapting the procedure described earlier.20 In brief, 9,10-diphenylanthracene in ethanol medium was used as the reference fluorophore with emission quantum yield (ϕrF) = 0.95. The ϕF for different systems were measured by using following equation: |
ϕsF = [ArFsns2/AsFrnr2]ϕrF
| (1) |
where, A is the absorbance at the excitation wavelength, F is the integrated emission area and n is the refraction index of the solvents used. Subscripts refer to the reference (r) or sample (s) compound.
The limit of detection (LOD) for the BA concentration was determined from following familiar method described by Thomsen et al. with reasonable certainty:21
where,
K = 3 has been considered as per IUPAC recommendation.
sbi = standard deviation for the blank measurement, and
S = Δconcentration/Δintensity (the slope of the calibration curve between fluorescence enhancement with change in BA concentration). By using the above
eqn (2), LOD of BA concentration ∼3.0 μM was obtained in 10 mM HEPES buffer at pH 7.3.
Theoretical calculations
All DFT and time-dependent DFT (TD-DFT) calculations reported in this work were performed with using Gaussian 09 program.22 Geometry based on most probable structure for Schiff-base ligand (L1) and its chelate complex with BA was carried out by considering B3LYP exchange-correlation functional. The 6-31G+(d,p) basis was set for atoms and the geometries were optimized in gas phase. The global minima of all these species were confirmed by the positive vibrational frequencies. To investigate the electronic properties of singlet excited states, TD-DFT calculation in water medium was applied by using the optimized geometries of the ground (S0) states of respective species. Excitation energies and respective oscillator strengths for the ground state geometry were then evaluated. The geometry of lowest singlet excited states was also optimized with the TD-DFT approach and fluorescence wavelength was calculated for this excited state geometry in aqueous medium by adapting conductor polarized continuum model (CPCM).
Bio-imaging studies
Arabidopsis Thaliana (Col-0) seeds were germinated in milli-Q water. Seven days old seedlings were fed with 2 mM BA solution for different period of time in each cases separately, then incubated with the probe (50 μM) for 30 min. Seedlings were then harvested and rinsed properly with water and placed under fluorescent microscope for imaging. Seven days old natural soil grown and MS (Murashige & Skoog basal medium) medium (contains 1 mM BA) grown seedling were similarly incubated with the probe for 30 min and placed under fluorescent microscope for imaging under same condition.
Results and discussion
BA/PBA selective complexation with Schiff-base ligand (L1)
In UV-Vis absorption studies of Schiff-base ligand L1 (5 μM), two characteristic absorption maxima ∼342 and 280 nm were observed in pure aqueous 10 mM HEPES, pH 7.3 buffer medium (Fig. 1A). Interestingly, the observed unmodified absorption profile over a long period of time (t1/2 > 4 days) clearly suggests that L1 is highly stable in pure aqueous buffer medium of pH 7.3 and the imine-bond is reluctant to participate in hydrolysis. In presence of increasing amount of BA (0–0.8 mM or 160 equiv.), the 342 nm absorption band was gradually shifted to higher wavelength with increasing intensity by maintaining an isosbestic point ∼345 nm and reached to its saturation at ∼365 nm for 160 equiv. of BA. Meanwhile, the concomitant decrease in intensity for 280 nm band with a slight red shift ∼4 nm by maintaining isobesticity ∼295 and 312 nm was also detected (Fig. 1A). Almost identical PBA induced L1 absorption profile was also observed with relatively lower analyte (PBA) concentration ∼100 equiv. at saturation (Fig. S1, ESI†). Such results certainly suggest the specific interaction and corresponding single complex species formation in both cases either L1 + BA or L1 + PBA in aqueous buffer, pH 7.3. Complexation in L1 + PBA was more pronounced but the detail analytical characterization of it was not included here owing to its less significance in plant systems. However, different analytical and spectroscopic methods as well as theoretical calculations were utilized to determine the structure of the probable complex, L1 + BA (1) (vide infra). Moreover, the ratiometric linear relation for the ratio of absorption intensities between 365 and 280 nm with BA concentrations is useful for determining the unknown concentration of BA (Fig. 1B).
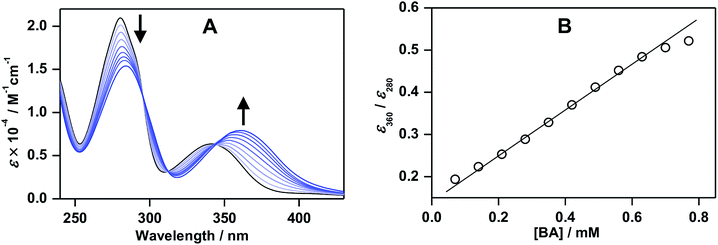 |
| Fig. 1 (A) UV-Vis absorption profile of L1 with increasing concentration of BA in 10 mM HEPES, pH 7.3: black curve, spectrum without BA. Measurement condition: L1, 5 μM; BA, 0–0.8 mM. The intensity changes with BA are depicted by arrows. (B) The extinction coefficient ratio between 365 and 280 nm with BA concentrations are shown. | |
Fluorescence property of L1: suitable for off-mode fluorometric studies in aqueous media
In fluorometric studies, a weak emission peak centered ∼555 nm for L1 (5 μM) was observed for either 370 nm or 270 nm excitation with a low fluorescence quantum yield (ϕF) ∼7.0 × 10−3 in aqueous 10 mM HEPES, pH 7.3 (Fig. 2A and C). On the contrary, a new emission band ∼420 nm with a shoulder ∼555 nm in non-aqueous acetonitrile (MeCN) medium upon 370 nm excitation occurs probably from locally excited singlet state and perhaps to some extent it may also participate in excited state reaction to generate red shifted shoulder fluorescence (Fig. S2, ESI†). These results suggest that the excited state reaction in aqueous medium is responsible for observing weak 555 nm fluorescence, whereas the intensity of 420 nm fluorescence arising from its locally excited singlet state is negligibly small. It has generally been observed that in comparison with that of ground state acidity or proton donation ability for the phenolic –OH moiety in the excited state is enhanced considerably and susceptible to participation in excited state proton transfer (ESPT) reaction with adjacent electron rich atom. Such excited state proton-reorganization generates additional stability of the ESPT state, but its corresponding ground state becomes less stable due to loss of aromaticity, which may cause large red-shift in the emission spectra.23 Schiff-base ligands containing phenolic –OH adjacent to the imine-N, excited state proton transfer (ESPT) reaction is primarily responsible for presently observed ∼185 nm stoke-shifted emission (Scheme 1). Probably the solvent water molecules play crucial role24 for transferring the proton to adjacent imine-N in its excited state, generating 555 nm fluorescence and that was clearly evident from DFT calculation with Gaussian 09 (vide infra). However, irrespective of solvent selection, the ESCT characteristic witnessing from DFT calculation (vide infra) in the ESPT state enhancing efficient non-radiative fluorescence deactivation process may be responsible for weak fluorescence.25 The low ϕF of L1 is suitable for off-mode fluorometric studies in aqueous medium. Selective enhancement of fluorescence in presence of suitable external regent in its on-mode may recognize L1 as a fluorescent sensor in aqueous media.
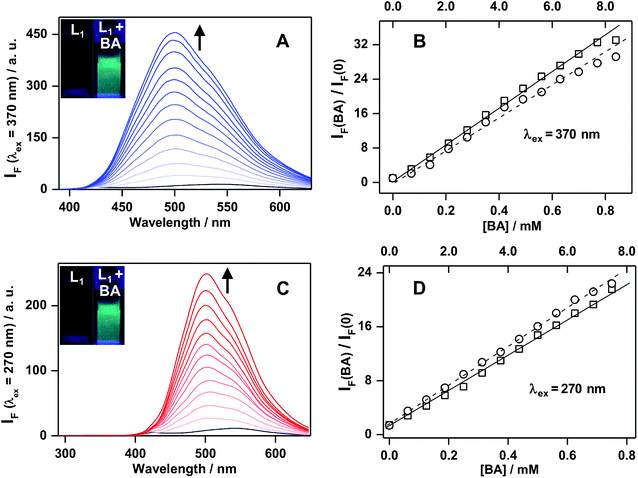 |
| Fig. 2 Fluorescence spectra of L1 (5 μM) on excitation by (A) 370 nm and (C) 270 nm with increasing concentration of BA (0–0.8 mM) in 10 mM HEPES, pH 7.3: black curve, spectrum without BA. Fluorescence intensity ratio in presence (0–0.8 mM) and absence of BA on excitation by (B) 370 nm and (D) 270 nm for two different L1 concentrations (5 μM: square, solid line; 50 μM: circle, dash line) are plotted. (B and D) The amount of BA used for 50 μM L1 are depicted by the upper x-axis. | |
BA/PBA selective ratiometric fluorescence response
A large increase of fluorescence centered ∼503 nm was observed with increasing amount of BA in presence of 5 μM of L1 and reached to its saturation at 160 equiv. in aqueous 10 mM HEPES buffer medium, pH 7.3. It is noteworthy that the amount of BA required for the fluorescence saturation is identical to that for obtaining UV-Vis intensity saturation, which indicates that the formation of BA + L1 complex may responsible for the large fluorescence enhancement. At saturation, BA induced such large fluorescence enhancement ∼35 fold (ϕ370 ∼ 0.22) for 370 nm excitation and ∼25 fold (ϕ270 ∼ 0.18) for 270 nm excitation was found respectively (Fig. 2A and C). Compared to BA, similar fluorescence intensity profiles were identified for PBA. Here only the exception for respective quantum yields (ϕF) at saturation for either excitation is higher by a factor ∼1.4, where the greater ϕF is probably due to the additional phenyl ring which imparts greater stability of the excited state of PBA + L1 complex. In addition, stronger complex forming affinity of PBA in comparison to BA was also confirmed from its lower requirement (∼100 equiv.) to reach fluorescence saturation (Fig. S3, ESI†). In presence of BA or PBA, the identical fluorescence spectra with a maximum at ∼503 nm for either excitations (370 or 270 nm) indicate their generation from same excited state species, though the ϕ270 is to some extent lower than to that of ϕ370 (Fig. 2 and S3, ESI†). For either excitation, the fluorescence intensity ratio in presence and absence of BA for L1 (5 μM) increases linearly with BA concentration (0–0.8 mM). Such ratiometric linear response even for 10 times higher L1 concentration (50 μM) for either excitation follow identical behavior for exactly same extent of different higher BA concentrations (0–8.0 mM), which is highly beneficial for the quantitative detection of any unknown BA concentration in the range 0–8.0 mM by suitably changing the L1 concentration (Fig. 2B and D). Moreover, the large stoke-shift between excitation and emission wavelength, particularly for 270 nm excitation, is advantageous for in vivo detection, since some artifact fluorescence at near visible region due to simultaneous excitation of small aromatic moieties/molecules present in biological systems would not perturb the desired 503 nm fluorescence.
In case of fluorescence transient studies, the mono-exponential behavior of excited state lifetime ∼1.4 ns for L1 in presence of BA compared to that of double-exponential behavior in absence of it with lifetimes ∼0.4 and 3.5 ns probably due to respective locally excited and ESPT state for L1 may be utilized for selective detection of BA in aqueous medium (Fig. 3 and Table 1). To verify BA selectivity, the fluorescence of L1 (5 μM) was monitored individually in presence of different biologically important molecules/ions viz. glucose, fructose, mannose, galactose, glycerol, Na+, Ca2+, K+, Mg2+, Fe2+/3+, Cu2+ and Zn2+ (∼200 equiv. each) in aqueous 10 mM HEPES buffer, pH 7.3, but failed to generate any noticeable enhanced fluorescence with an exception for Zn2+ ion with small fluorescence increment ∼4-fold (Fig. 4). Interestingly, BA induced fluorescence increment was not disturbed in presence of vicinal diol containing molecules like glucose, glycerol etc. under physiological buffering conditions (Fig. 5). Such unperturbed ratiometric response for L1 in presence of large excess of those molecules is also important for in vivo BA detection in leaving species. It is noteworthy to mention that the obtained limit of detection (LOD) ∼3.0 μM is useful for detection of trace amount of BA. Hence, L1 can be an excellent choice for analytical determination of BA in leaving cells as well as in industrial wastes.
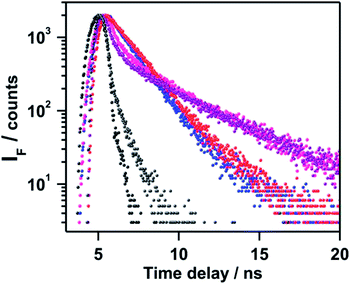 |
| Fig. 3 Fluorescence transients of L1 (5 μM) (purple and pink for 370 and 280 nm excitation respectively, emission collected at 555 nm) and L1 in presence of 0.8 mM of BA (blue and red for 370 and 280 nm excitation respectively, emission collected at 505 nm) in 10 mM HEPES, pH 7.3. Scattering profile for 370 and 280 nm are shown by black and grey respectively. | |
Table 1 Fluorescent transient decay parameter
System |
Excitation/emission (nm) |
Lifetimes (ns) |
Contribution (%) |
Residual(χ2) |
L1 |
370/550 |
0.41 |
81.7 |
1.01 |
3.32 |
18.3 |
L1 |
270/550 |
0.35 |
84.9 |
1.12 |
3.30 |
15.1 |
L1 + BA |
370/500 |
1.42 |
— |
0.98 |
L1 + BA |
270/500 |
1.45 |
— |
1.02 |
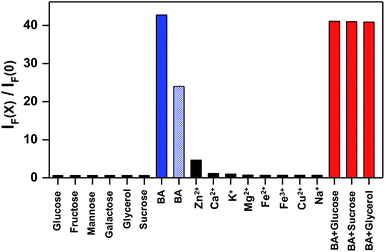 |
| Fig. 4 Ratio of 505 nm fluorescence intensity of L1 (5 μM) in presence of different analytes or mixture of analytes in 10 mM HEPES, pH 7.3 are depicted by bar-diagram; excitation wavelength: 370 nm (solid bar) and 270 nm (shaded bar). | |
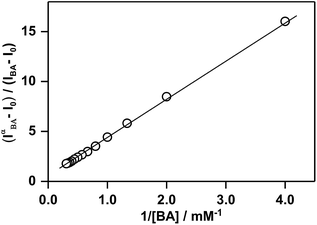 |
| Fig. 5 The Benesi–Hildebrand plot of (IαBA − I0)/(IBA − I0) vs. 1/[BA]: (IαBA), fluorescence intensity in presence of large excess of BA; I0, intensity in absence of BA and IBA, intensity at various concentration of BA. Experimental conditions: L1, 5 μM; BA, 0–0.8 mM; buffer, 10 mM HEPES, pH 7.3 (excitation wavelength: 370 nm). | |
BA sensing mechanism
Though the broad spectral line obscure any assignment of proper chemical shift for tetrahedral borate in aqueous buffer medium, pH 7.3, relatively higher peak intensity of borate formation compared to such generation from standard BA as in reference for 11B NMR spectra indicates the L1 + BA complex formation (Fig. S4, ESI†). In ESI-MS+ analysis, the observed m/z at 277.9687 (cald m/z: 277.0637) for L1 in presence of 160 equiv. of BA suggests that one water molecule is eliminated during condensation reaction to form 1
:
1 complex in between L1 and BA (Fig. S5, ESI†). The 1
:
1 stoichiometry was also verified from the linearity with almost unit intercept (0.85 ± 0.10) of Benesi–Hildebrand26 plot of (IαBA − I0)/(IBA − I0) vs. inverse of BA concentrations, where IαBA and I0, fluorescence in presence of large excess and absence of BA; IBA, fluorescence in presence of various BA concentrate ions (Fig. 5).
Based on evidences, molecular formula for 1
:
1 chelate complex as revealed from ESI-MS+ analysis, the ground state geometry for most probable structure was optimized by DFT method for investigating the absorption to the singlet excited states via TD-DFT calculations in solvent water (Fig. S7, ESI†). In addition, similar calculation was performed for free L1 to justify for present consideration for the proposed structural geometry of the L1 + BA chelate complex. It has been presumed that electron deficient planar trigonal BA adopts tetrahedral geometry after coordination with electron rich phenolic-O and imine-N centers in the proposed structure (Scheme 1). The excitation energies and oscillator strengths as well as excited state compositions for L1 and 1
:
1 complex are depicted in Table S1, ESI.† The orbital energy diagrams for HOMO to LUMO transition of these systems are also plotted in Fig. 6. On comparing the experimental absorption spectrum of pure L1, HOMO (42a) to LUMO (43a) transition (fcal = 0.142, ΔE = 3.47 eV or 357 nm) is mainly responsible for experimentally observed 342 nm absorption peak, whereas high energy 280 nm absorption maxima is observed for HOMO − 1 (41a) to same LUMO (43a) transition (fcal = 0.623 ΔE = 4.27 eV or 290 nm) (Fig. 6 and S8, Table S1, ESI†). Upon chelation with BA, both those L1 absorption bands were red shifted to 365 and 285 nm as observed experimentally and that are nicely correlated with theoretically obtained ΔE values 3.20 eV (387 nm in wavelength unit) and 3.98 eV (299 nm in wavelength unit), respectively (Fig. 6, Table S1, ESI†). The emissive property for L1 in absence and presence of BA were investigated using the TD-DFT method in solvent water with the optimized excited state geometry. The ESPT reaction for the L1 is clearly evident in the excited state optimized geometry, where H-atom was transferred to imine-N by the deprotonation from adjacent phenolic-OH moiety (Fig. S7, ESI†).
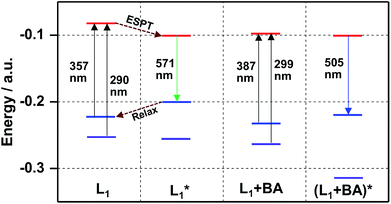 |
| Fig. 6 Energies of frontier molecular orbital (FMO) for ligand (L1: ground state (S0) and L*1: first excited state (S1)) and L1 + BA (LB: ground state, LB*: first excited state) obtained from DFT and TD-DFT calculation. The relative energy of HOMO − 1, HOMO, LUMO are depicted by purple, blue and red line respectively. Vertical electronic transitions from HOMO − 1 or HOMO to LUMO for respective wavelength are indicated by arrow. The opposite arrow direction for excited state is for the conveniences of explaining the respective emission wavelength. The ESPT reaction and corresponding ground state relaxation (Relax) are shown by brown arrow. | |
The calculated 2.17 eV (wavelength unit, 571 nm) vertical transition energy for S0 → S1 transition nicely matched with experimentally observed fluorescence at ∼555 nm (Fig. 6, Table S1, ESI†). It is clearly evident that such a large red shifted fluorescence at ∼555 nm from its excitation wavelength 370 or 270 nm is probably not due to stabilization of excited state by dipole–dipole interaction in high dielectric water medium, rather due to excited state ESPT reaction (Scheme 1, Fig. 6, Table S1, ESI†). Interestingly, some extent of charge transfer character from main fluorophoric moiety containing benzene ring in the HOMO to triazole nitrogen atoms in the LUMO, particularly for the excited state geometry, may also be responsible for weak 555 nm ESPT fluorescence (Fig. S8, ESI†). Since two centers (phenolic-O and imine-N) involving for ESPT reaction are now coordinated with B centre upon chelation with BA, ESPT process may not be feasible and hence the blue-shifted strong fluorescence at ∼503 nm was found experimentally (Scheme 1). This 503 nm emission probably generates mainly from locally excited singlet state. Indeed, the TD-DFT calculation on the optimized geometry in its excited state has shown S0 → S1 transition of energy ∼2.45 eV corresponding with 505 nm transition (Fig. 6, Table S1, ESI†). It is noteworthy to mention that the experimentally observed the same 503 nm fluorescence by either 270 or 370 nm excitation indicates the generation of same excited states by either excitations, which is rationalized from theoretically obtained HOMO − 1 (67a) to LUMO (68a) and HOMO (66a) to same LUMO (68a) transition (Fig. 6 and S8, ESI†). Moreover, HOMO and LUMO involving for this transition hardly shows any charge transfer behaviour particularly in the fluorophoric moiety, which may responsible for large increase of fluorescence quantum yield by chelation with BA compared to that of free L1 (Fig. S8, ESI†).
Bio-imaging studies for living plant
In control experiment, A. thaliana seeds were germinated and grown in water and no BA was supplied externally. When these seedlings were incubated with L1 (50 μM) for 30 min and the roots were monitored under fluorescence microscope no fluorescence was detected (Fig. 7A). Next we fed these water grown seedlings with 2 mM BA for 1, 2 and 4 h, rinsed, incubated with the L1 (50 μM) for 30 min and imaged the roots under fluorescence microscope. A greenish-blue fluorescence was observed from the roots for 370 nm excitation and the emission intensity was increased gradually with time until the saturation was identified at 4 h of BA feeding (Fig. 7B–D). Increasing fluorescence intensity with time suggests that BA concentration within roots increases gradually with time and that may reflect more detail insight in natural BA intake by plants. To assess the natural BA concentration in roots, seedlings grown in soil under natural conditions and seedlings grown in tissue culture medium were incubated both with the L1 (50 μM) for 30 min respectively. Very weak fluorescence intensity for soil grown roots compared to that of large fluorescence enhancement in artificial growth conditions clearly indicates that natural BA intake in the roots is well below the mM range of concentration (Fig. 8). It is important to emphasize here that the B concentration in natural soil depends on geographical location and varies from places to places within the range from 2–100 ppm.27 Obviously B accumulation in plant roots varies accordingly. Again high B accumulation in roots is toxic irrespective of the nature of the plants. The range for deficiency and toxicity is very narrow and delicately changes from one plant system to others. Our present investigation suggests that the probe can nicely detect the root B concentration as low as 20 ppm. Extensive investigations with improved sensing ability by suitably tuning the Schiff-base precursor are further required by taking individual plant system for monitoring actual B accumulation, its requirement and toxicity level in different soil sources.
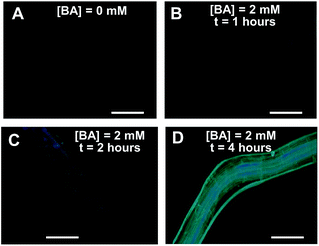 |
| Fig. 7 Fluorescence images of the plant root grown in water (A) without or (B–D) with BA feeding. BA feeding was done for different time points: B, 1 h; C, 2 h; C, 4 h. The scale bars: 400 μm. | |
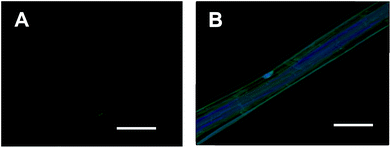 |
| Fig. 8 Comparison of BA-dependent fluorescence in the roots of seedlings under (A) natural (soil grown) and (B) in artificial (MS medium grown) conditions. The scale bars: 400 μm. | |
Conclusions
The hydrolysis resistant Schiff-base ligand (L1) consisting of an aromatic aldimine moiety along with the adjacent phenolic-OH group is reluctant to exhibit noticeable fluorescence owing to ESPT followed by charge transfer from phenolic to triazole precursor in aqueous buffer medium at pH 7.3. On the contrary, under same physiological conditions, the ligand (L1) selectively binds with BA to form 1
:
1 chelate complex which inhibits the charge transfer process, resulting large increment of fluorescence. Various spectroscopic, analytical techniques as well as theoretical studies are done mechanistically to ascertain the chelate complex formation and subsequent explanation for fluorescence enhancement. BA sensing ability of L1 remains invariant in presence of different biologically relevant molecules/ions including cis-diols and undoubtedly considered as a rare BA sensor in aqueous medium and probably for the first time it has been explored for bio-imaging purpose taking Arabidopsis Thaliana as model plant system.
Acknowledgements
Authors acknowledge JU and Maulana Azad College, Kolkata, for departmental facilities. This work was supported by Research Projects (SB/FT/CS-089/2013) (PPP) and UGC (ERO) MRP (PSW-196/13-14) (AR). SD acknowledged UGC (ERO) MRP, for research Grant (PSW-197/13-14).
Notes and references
-
(a) W. G. Wood, Environ. Health Perspect., 1994, 102, 5 CrossRef;
(b) D. Habes, S. Morakchi, N. Aribi, J. P. Farine and N. Sultani, Pestic. Biochem. Physiol., 2006, 84, 17 CrossRef CAS PubMed;
(c) B. Lesar, F. Budija, P. Kralj, M. Petric and M. Humar, Eur. J. Wood Wood Prod., 2012, 70, 365 CrossRef CAS;
(d) A. Temiz, G. Alfredsen, M. Eikenes and N. Terzıev, Bioresour. Technol., 2008, 99, 2102 CrossRef CAS PubMed.
- C. Dordas, M. J. Chrispeels and P. H. Brown, Plant Physiol., 2000, 124, 1349 CrossRef CAS PubMed.
- C. D. Eckhert, J. Nutr., 1998, 128, 2488 CAS.
- T. Matoh, K. I. Ishigaki, O. Kaori and T. I. Azuma, Plant Cell Physiol., 1993, 34, 639 CAS.
- D. H. Lewis, New Phytol., 1980, 84, 209 CrossRef CAS PubMed.
- Ed. C. D. Hunt, R. Macrae, R. K. Robinson and M. J. Sadler, Boron, in Encyclopedia of Food Science, Food Technology and Nutrition, Academic Press, London, 1993, vol. 1, p. 440 Search PubMed.
- W. M. Dugger, A. Laiichi and R. L. Bicleski, Inorganic plant nutrition, 1983, vol. 15B, p. 626 Search PubMed.
-
(a) J. Polster, M. Schwenk and E. Bengsch, Z. Naturforsch., C: J. Biosci., 1992, 47, 102 CAS;
(b) W. D. Loomis and R. W. Durst, BioFactors, 1992, 3, 229 CAS.
- P. P. Powers and W. G. Wood, Plant Soil, 1997, 193, 1 CrossRef.
-
(a) A. M. G. Campana, F. A. Barrero and M. R. Ceba, Analyst, 1992, 117, 1189 RSC;
(b) N. Chimpalee, D. Chimpalee, B. Boonyanitchayakul and D. T. Burns, Anal. Chim. Acta, 1993, 182, 643 CrossRef.
- W. Wang, G. Springsteen, S. Gao and B. Wang, Chem. Commun., 2000, 1283 RSC.
- S. Arimori, C. J. Ward and T. D. James, Chem. Commun., 2001, 2018 RSC.
- R. Nishiyabu, Y. Kubo, T. D. James and J. S. Fossey, Chem. Commun., 2011, 47, 1106 RSC.
-
(a) L. I. Bosch, T. M. Fyles and T. D. James, Tetrahedron, 2004, 60, 11175 CrossRef CAS PubMed;
(b) L. Zhu, S. H. Shabbir, M. Gray, V. M. Lynch, S. Sorey and E. V. Anslyn, J. Am. Chem. Soc., 2006, 128, 1222 CrossRef CAS PubMed;
(c) S. Franzen, W. Ni and B. Wang, J. Phys. Chem. B, 2003, 107, 12942 CrossRef CAS;
(d) W. J. Ni, G. Kaur, G. Springsteen, B. H. Wang and S. Franzen, Bioorg. Chem., 2004, 32, 571 CrossRef CAS PubMed.
-
(a) R. Capelle, Anal. Chim. Acta, 1964, 24, 555 CrossRef;
(b) J. Koos, F. V. Staden and T. A. V. Merwe, Analyst, 2000, 125, 2094 RSC.
- H. Matsuo, Y. Miyazaki, H. Takemura, S. Matsuoka, H. Sakashita and K. Yoshimura, Polyhedron, 2004, 23, 955 CrossRef CAS PubMed.
- S. Das, Y. Sarkar, S. Mukherjee, J. Bandyopadhyay, S. Samanta, P. P. Parui and A. Ray, Sens. Actuators, B, 2015, 209, 545 CrossRef CAS PubMed.
- K. L. Wang, M. K. Leung, L. G. Hsieh, C. C. Chang, K. R. Lee, C. L. Wu, J. C. Jiang, C. Y. Tseng and H. T. Wang, Org. Electron., 2011, 12, 1048 CrossRef CAS PubMed.
-
(a) M. Blangetti, H. Rosso, C. Prandi, A. Deagostino and P. Venturello, Molecules, 2013, 18(1), 1188 CrossRef CAS PubMed;
(b) J. X. Qiao and P. Y. S. Lam, Synthesis, 2011, 829 CrossRef CAS PubMed;
(c) S. Kotha, K. Lahiri and D. Kashinath, Tetrahedron, 2002, 58, 9633 CrossRef CAS;
(d) J. Luong, R. Gras, H. J. Cortes and R. A. Shellie, Anal. Chim. Acta, 2013, 805, 101 CrossRef CAS PubMed.
- S. Das, S. Biswas, S. Mukherjee, J. Bandyopadhyay, S. Samanta, I. Bhowmik, D. K. Hazra, A. Ray and P. P. Parui, RSC Adv., 2014, 4, 9656 RSC.
- V. Thomsen, D. Schatzlein and D. Mercuro, Spectroscopy, 2003, 18, 112 CAS.
-
(a) M. J. Frisch, et al., Gaussian 09, revision D. 01, Gaussian, Inc., Wallingford, CT, 2009 Search PubMed;
(b) S. Miertus, E. Scrocco and J. Tomasi, Chem. Phys., 1981, 55, 117 CrossRef CAS;
(c) V. Barone, M. Cossi and J. Tomasi, J. Comput. Chem., 1998, 19, 404 CrossRef CAS.
- A. P. Demchenko, K. C. Tangb and P. T. Chou, Chem. Soc. Rev., 2013, 42, 1379 RSC.
-
(a) P. Jaramillo, K. Coutinho and S. Canuto, J. Phys. Chem. A, 2009, 113, 12485 CrossRef CAS PubMed;
(b) V. S. Padalkar, A. Tathe, V. D. Gupta, V. S. Patil, K. Phatangare and N. Sekar, J. Fluoresc., 2012, 22, 311 CrossRef CAS PubMed.
- M. C. Cuquerella, M. A. Miranda and F. Bosca, J. Phys. Chem. A, 2006, 110, 2607 CrossRef CAS PubMed.
- H. A. Benesi and J. H. Hildebrand, J. Am. Chem. Soc., 1949, 71, 2703 CrossRef CAS.
- M. Benderdour, T. B. Van, A. Dicko and F. Belleville, J. Trace Elem. Med. Biol., 1998, 2, 2 Search PubMed.
Footnote |
† Electronic supplementary information (ESI) available: For additional spectroscopic information. See DOI: 10.1039/c5ra08086j |
|
This journal is © The Royal Society of Chemistry 2015 |