DOI:
10.1039/C5RA07966G
(Paper)
RSC Adv., 2015,
5, 48835-48841
Ultra sensitive and wide-range pH sensor based on the BSA-capped Cu nanoclusters fabricated by fast synthesis through the use of hydrogen peroxide additive†
Received
30th April 2015
, Accepted 27th May 2015
First published on 27th May 2015
Abstract
The accurate estimation of pH in the environment and on the cellular level is important in environmental, biomedical and bioprocess applications. Herein, we report an ultra-sensitive and wide-range pH sensor based on BSA-capped Cu nanoclusters (CuNCs), fabricated using a fast synthesis method through the use of hydrogen peroxide as an additive. Owing to its strong oxidation capacity, hydrogen peroxide partially destroys the peptide and disulfide bonds in the BSA molecule and results in an increased exposure of hydrophilic groups capable of protonation, which greatly accelerate the formation of CuNCs and improve the response of the CuNCs towards pH fluctuation in the surrounding environment. The results demonstrate that the decrease in repulsion and conformational change of BSA with decreasing pH values induce the aggregation of CuNCs, leading to a color change and fluorescence quenching of the CuNCs at low pH values. The fluorescence intensity exhibits a linear relationship over the pH range of 2–14 and increases by around 20-fold with greater fluorescence at higher pH values. The sensitivity and pH range are much better than those of other metal nanocluster pH sensors reported in the literature. The pH probe is also sensitive to different buffer solutions, except for those containing ions that could react with the CuNCs. The ionic strength of the buffers has little influence on the pH-responsive behaviour. The proposed pH sensor has been successfully applied for measuring the pH value of natural water and the intracellular pH of RBL-2H3 cells. The study also provides a promising candidate for application in biological, medical and pharmaceutical fields.
1 Introduction
Very small variations in pH value can have a devastating effect on plants and animals. In our surroundings, acid rain, excessive human activities and untreated sewage may cause changes in environmental pH value and the acidification of soils, streams, lakes and seawater, which will pollute our drinking water.1 In life science, intracellular pH is an essential parameter for cell, enzyme and tissue activities, and plays a vital role in cell metabolism processes, including proliferation and apoptosis.2 As an abnormal intracellular pH value is related to improper cell function and growth, it may affect human physiology, such as in cancer and neurological disorders. Therefore, measuring pH distribution and fluctuation with high temporal–spatial resolution in living cells is essential for advancing our understanding of cell biology.3 Thus, the accurate estimation of pH in the environment and on the cellular level is important in environmental, biomedical and bioprocess applications.4
The unique properties of metal nanoclusters attract great attention due to their molecule-like behaviour, including discrete electronic states and size-dependent fluorescence. The intensity and wavelength of fluorescence are highly sensitive to the surrounding environment, which has made nanoclusters attractive as functional materials in the development of versatile sensors.5 To date, studies have been carried out into the pH-responsive optical properties of metal nanoclusters, such as silver nanoclusters,6 gold nanoclusters,7 copper nanoclusters,8 silicon nanoclusters,9 cerium/gold nanoclusters10 and gold/copper nanoclusters.11 Proteins at high concentrations are often applied as the template and reducing agent for building stable and biocompatible metal nanoclusters. Bovine serum albumin (BSA) is the most popular. There are 28 cysteine and 20 tyrosine residues in each BSA molecule, leading to greater capping and reducing capabilities. Many investigations have demonstrated that the pH response of metal nanoclusters strongly depends on the features of the template, including its charge density and protonation level.12 However, BSA-capped nanoclusters often possess pH-insensitive fluorescence properties because of the low degree of exposed hydrophilic groups capable of protonation.13
This study reports a new fluorescent pH sensor based on BSA-capped copper nanoclusters (CuNCs), fabricated using a fast synthesis method through the use of hydrogen peroxide as an additive. The addition of hydrogen peroxide partially destroys the peptide and disulfide bonds in the BSA molecule, which increases the exposure of hydrophilic groups capable of protonation. This greatly accelerates the formation of CuNCs and improves the response of the CuNCs towards pH fluctuation in the surrounding environment. The as-prepared pH sensor provides advantages of high sensitivity, wide pH range and excellent reversibility compared with other metal nanoclusters.
2 Experimental
2.1 Materials
Bovine serum albumin (BSA), hydrogen peroxide (H2O2), copper sulphate (CuSO4) and sodium hydroxide (NaOH) were purchased from Sigma-Aldrich (Mainland, China). Britton–Robinson buffer solution (BR buffer, H3PO4–HAc–H3BO3, 0.04 M) was prepared and its pH value was adjusted using 0.2 M NaOH solution. Phosphate-buffered saline (PBS, pH 7.0, Na2HPO4–KH2PO4–NaCl–KCl, 0.01 M) was prepared in the laboratory. Other reagents were of analytical reagent grade and purchased from the Shanghai Chemical Company (Shanghai, China). Ultrapure water (18.2 Ω cm), purified using a Milli-Q purification system, was used throughout the experiment.
2.2 Apparatus
Transmission electron microscopy (TEM) imaging was conducted on a JEOL 2010 FEG microscope at 200 keV. The sample was prepared by dispensing a small amount of dry powder in ultrapure water. Then, one drop of the suspension was dropped on 300 mesh copper TEM grids covered with thin amorphous carbon films. The pH values of samples were measured using a PHS-3D pH meter (Shanghai Precision Scientific Instruments Co., Ltd., China). Fluorescence spectra and intensities were recorded on a Cary Eclipse fluorescence spectrophotometer (Agilent, Japan). Circular dichroism (CD) measurements were performed on a MOS-450 circular dichroism spectrometer using a 0.01 cm quartz cell. The percentages of various conformations have been determined for both CuNCs and CuNCs-c by using the SELCON3 analysis programme. The zeta potential measurements were carried out using a ZETASIZER2000 Zeta Potential Analyzer.
2.3 Synthesis of CuNCs
A CuSO4 solution (10 ml, 20 mM) was added to a BSA solution (50 ml, 15 mg ml−1). After stirring for 5 min, its pH was slowly adjusted to 12 using the NaOH solution (1 M). Then, a H2O2 solution (10 ml, 0.4 M) was injected into the above solution under vigorous agitation. After that, the mixed solution was heated at 55 °C for 1 h to obtain the CuNC solution. For comparison, a control sample (termed as CuNCs-c) was also prepared using the same procedure, but with no addition of H2O2.
2.4 Procedure for measuring pH value
The CuNC solution (200 μl) was mixed with BR buffer (600 μl). After 5 min of incubation, the mixture was subjected to fluorescence measurements on the fluorescence spectrophotometer with an excitation wavelength of 320 nm and emission wavelength of 420 nm. The fluorescence signal was monitored using a photomultiplier tube on the fluorescence spectrophotometer and was recorded by a computer. For each pH detection, the fluorescence measurement was repeated thrice, and the average fluorescence signal was determined.
2.5 Method for studying the interference of ionic strength
The CuNC solution (200 μl) and different concentrations of NaCl solution (200 μl) were mixed with BR buffer (pH 10.1, 400 μl). After 5 min, the fluorescence was measured and recorded on the fluorescence spectrophotometer with an excitation wavelength of 320 nm and emission wavelength of 420 nm.
2.6 Measurement of zeta potentials
The zeta (ζ) potential of the CuNCs was examined as a function of pH value using dynamic light scattering (DLS). The DLS samples were prepared as follows: the CuNC solution (100 μl) was diluted in BR buffer (pH 2–14, 2.9 ml). Then, the mixed solutions were passed through millipore filters with a pore size of 0.22 μm to remove dust. The zeta potential of the solution was determined using the Zeta Potential Analyzer.
2.7 The pH-dependent intracellular fluorescence imaging
RBL-2H3 cells, in Roswell Park Memorial Institute 1640 (RPMI 1640) medium supplemented with 10% fetal BSA, were added to each well of a 48-well plate (200 μl per well). The cells were cultured first for 24 h in an incubator (37 °C, 5% CO2), then for another 24 h after the culture medium was replaced with 300 μl of RPMI 1640 containing 30 μl of the CuNCs. Before imaging, the CuNC-loaded cells were rinsed three times and incubated with 1.0 ml of BR buffer at various pH values for 10 min. The cells were imaged using a DSU live-cell confocal microscope (Olympus, Japan) system with laser excitation of DAPI, and the images were analyzed using image-Pro Plus software.
3 Results and discussion
3.1 Fluorescent characteristics
A new strategy has been successfully developed for the fast synthesis of CuNCs through the use of H2O2 as an additive. The results verify that the addition of H2O2 greatly accelerates the formation of CuNCs. The synthesis of CuNCs is reduced to less than 1 hour. The time is much less than with the conventional method in the absence of H2O2 (about 30 days). In this study, we focus on the fluorescence characteristics of the resulting CuNCs. Fig. 1 presents the typical fluorescence spectra of the CuNCs and CuNCs-c. Two kinds of CuNC products give very similar fluorescence spectra with the same shape and maximum emission wavelength, verifying that the two kinds of CuNCs have analogous nanostructures and similar particle size distributions. Interestingly, the CuNCs give a higher fluorescence intensity than the CuNCs-c. Our study reveals that in the CuNCs-c exists a high concentration of Cu2+ due to the weak reducing ability of BSA. The residual Cu2+ will not only result in a low content of CuNCs but also partially quench the fluorescence, so that the CuNCs-c exhibit a relatively low fluorescence intensity. The results demonstrate that the addition of H2O2 during the synthesis of CuNCs results in an enhanced fluorescence intensity.
 |
| Fig. 1 Excitation spectrum (a) of the CuNCs and fluorescence spectra of the CuNCs (b) and CuNCs-c (c). | |
The solvation dynamics of the as-prepared CuNCs were studied by measuring the fluorescence spectra at different excitation wavelengths. Fig. 2 indicates that the fluorescence emission peak changes with the change in excitation wavelength. When the excitation wavelength was changed from 320 to 420 nm, the emission spectrum was wavelength-tunable, with the emission maximum shifting from 410 nm to 480 nm (as shown in Fig. S1†). The fluorescence intensity decreases with the increase in excitation wavelength. This strong excitation wavelength-dependent fluorescence of the CuNCs originates from the “giant red-edge effect”.14 When the CuNCs are present in a polar solvent, the solvation dynamics slow down to the same time scale as the fluorescence due to the local environment of the CuNCs. The giant red-edge effect of the CuNCs disappears in a nonpolar solvent, thus leading to a narrow fluorescence peak that is independent of the excitation wavelength. The discovery of an underlying strong excitation wavelength-dependent fluorescence mechanism offers guidelines for the development of CuNCs-based optical devices. The results also demonstrate that the fluorescence of the as-prepared CuNCs is sensitive to environment polarity.
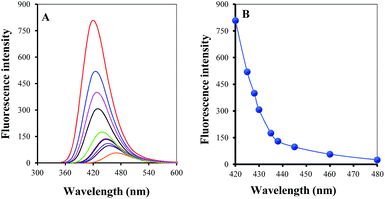 |
| Fig. 2 Fluorescence spectra (A) of the CuNCs with excitation wavelengths of 320, 335, 340, 345, 355, 360, 380, 400 and 420 nm (from top to bottom) and relationship (B) between the emission wavelength and the fluorescence intensity. | |
3.2 Response to pH value
The sensing performance of the as-prepared CuNCs is characterized using fluorescence measurements in BR buffers from pH 2 to 14. Fig. 3 shows that the CuNCs are highly sensitive to the pH value, adjusted using the BR buffer. The fluorescence intensity will rapidly increase with an increase in the pH value in the surrounding environment. The fluorescence signal reaches its maximum value at pH 14, which is more than 20-fold that at pH 2. Further, Fig. 3B indicates that the fluorescence intensity changes in a linear fashion over the pH range from 2 to 14. The linear equation is F = 92.56pH − 221.25 and the corresponding correlation coefficient is about 0.9971. For comparison, the analytical characteristics of other metal nanocluster pH sensors reported in the literature are listed in Table 1. From Table 1, we can observe that the novel CuNC pH sensor provides the best sensitivity and the widest ranging pH response. Thus, the as-prepared CuNCs can be widely applied for measuring the pH value of environmental and living samples. Moreover, Fig. 3B also shows that the sensitivity and pH range of the CuNCs are much better than those of the CuNCs-c. This is because the low fluorescence intensity of the CuNCs-c leads to a relatively low sensitivity pH response compared with the CuNCs. In addition, a relatively high isoelectric point and low exposure of hydrophilic groups capable of protonation result in the narrow pH-sensitive range of the CuNCs-c. The results also confirm that the introduction of H2O2 in the synthesis obviously improves the pH-dependent optical properties.
 |
| Fig. 3 Fluorescence spectra (A) of the CuNCs at pH 2–14 and the relationship (B) between the fluorescence intensity of the CuNCs (upper) and CuNCs-c (lower) at 420 nm and the pH value. | |
Table 1 Analytical characteristics of metal nanocluster pH sensors
Nanocluster |
Stabilizer |
Sample |
pH range |
Sensitivity |
Ref. |
Ce/AuNC |
BSA |
HeLa cells |
6–9 |
2 |
10 |
AgNC |
Poly-ethylenimine |
|
5.02–7.96 |
10 |
12 |
AuNC |
Glutathione and citrate |
Cells |
4.1–8.6 |
|
7 |
CuNC |
BSA + hydrazine hydrate |
CAL-27 cells |
6–12 |
|
15 |
The as-prepared CuNCs also display a distinct color variation with decreasing pH (shown in Fig. 4), namely from red (basic medium) to orange color (neutral medium) and finally to yellow color (acidic medium). At the same time, its fluorescence intensity decreases with decreasing pH value. This imparts an excellent characteristic to this probe as a color indicator in pH detection. From Fig. 4, we observe that the CuNC solution is transparent and no precipitation can be seen when the pH value is more than 4.01, indicating excellent water-solubility. When the pH value decreases to 4.01, BSA begins to precipitate from the CuNC solution. The precipitation reaches its maximum at pH 2.36, indicating an isoelectric point of about 2.36 for the BSA in the CuNCs system. The isoelectric point is lower than that of BSA in the CuNCs-c system (about 3.26) (shown in Fig. S2†). The results demonstrate that the introduction of H2O2 during the synthesis decreases the isoelectric point of BSA. This is mainly because the use of H2O2 partially destroys the peptide and disulfide bonds due to its strong oxidation capacity, which enhances the water-solubility and leads to a decrease in the isoelectric point. Interestingly, the precipitate formed in the acidic medium can be dissolved in the basic medium and the solution color becomes red and transparent again.
 |
| Fig. 4 Photographs showing the color changes of the CuNCs in BR buffer at different pH values under visible light (upper) and UV light (lower). | |
To test the response of the as-prepared CuNCs to pH change, CuNC solution was well mixed with BR buffer (pH 7 or 10) and then its fluorescence signal was immediately measured using the fluorophotometer. The results show that the fluorescence intensity reaches its maximum value within 20 s, indicating a very fast response to pH change. Further, the effect of the standing time on the fluorescence intensity was investigated in the laboratory. Fig. S3† indicates that the fluorescence intensity can remain almost unchanged over the course of at least 60 min, indicating an excellent stability for pH measurement.
To investigate the reversibility of the CuNCs, the pH value was changed from 11.13 to 3.01 and back to 11.13 using acid and base as the modulators. The fluorescence intensity was measured at each pH value and results are shown in Fig. 5. It can be seen that the cycles can be repeated 7 times without obvious fatigue. A small decrease in fluorescence intensity was observed for the reason that the volume of the solution became larger after each cycle. The reversibility of the CuNCs in strong acid (1 M HCl) and strong base (5 M NaOH) media was tested in this study. The results show that the fluorescence in strong acid and strong base media can rapidly be restored by adjusting the pH value using strong acid or strong base solution, indicating an excellent reversibility. Based on this excellent reversibility, we think that the aggregation at low pH values does not influence the crystal structure and particle size of the cores in the CuNCs. The formed soft agglomerate is easy to dissociate into the pristine CuNCs. Thus, the fluorescence intensity can rapidly increase at high pH. All of these results afforded significant evidence for the advantages of the CuNCs for application in various environments.
 |
| Fig. 5 The fluorescence intensity of the CuNCs reversibly went up and down when alternating the pH value. One cycle means that the fluorescence intensity was measured as the value changed from pH 11.13 to 3.01 and then from pH 3.01 to 11.13. | |
The proposed pH sensor was also sensitive to other commonly used buffer solutions such as tris(hydroxymethyl)aminomethane–HCl buffer (Tris–HCl), acetic acid–sodium acetate buffer (HAc–NaAc), phosphate buffer (PB) and borax–HCl buffer (as shown in Fig. S4†). Good corresponding linear relationships were obtained between the pH values measured using the pH meter and the fluorescence intensity of the CuNCs, revealing that the fluorescence intensity changes were primarily due to the variation of the pH values rather than the reaction between the CuNCs and the ions contained in the buffer solutions. More importantly, the CuNCs could react with anions contained in the buffer solutions, resulting in deviations in the pH determination. In spite of this, the pH-response was not affected by the ionic strength. The fluorescence intensity of the CuNCs remained almost constant when exposed to 0.0–1.0 M NaCl solutions (as shown in Fig. 6), indicating that ionic strength had no significant effect on the pH sensor.
 |
| Fig. 6 Fluorescence peak intensity of the CuNCs in BR buffer solution of pH 10.1 containing different concentrations of NaCl. | |
3.3 Mechanism of pH response
Resonance light scattering (RLS), zeta potential, transmission electron microscopy (TEM) and circular dichroism (CD) of the as-prepared CuNCs at different pH values were investigated to understand the mechanism of response to pH value. Fig. 7A displays the RLS spectra of the CuNCs at different pH values. It can be seen that the RLS spectra of the CuNCs at different pH values have a similar shape. However, the RLS intensity is highly dependent on the pH value. When the pH value is close to the isoelectric point of BSA, the RLS intensity rapidly increases with decreasing pH value. This is because the loss of charge results in an obvious aggregation process, indicating a strong RLS signal. The BSA on the CuNC surface is negatively charged in the basic aqueous solution with increasing pH value, and is stabilized against aggregation due to the negative electrostatic repulsion. Thus, the BSA on the CuNC surface gives a relatively weak RLS intensity at pH 8 and 12.
 |
| Fig. 7 (A) Resonance light scattering spectra of the CuNCs at pH 3, 5, 8 and 12 (from top to bottom). Condition: λex = λem (290–800 nm). (B) The relationship between the zeta potential of the CuNCs and the pH value. | |
Fig. 7B presents the relationship between the zeta potential of the CuNCs and the pH value. The zeta potential of the as-prepared CuNCs is sensitive to the pH value of the solution. The CuNCs exhibit a positive zeta potential when the pH value is less than 2.36, indicating a positive charge on the surface of the CuNCs. The zeta potential is equal to zero at pH 2.36, indicating an isoelectric point of 2.36. The isoelectric point is lower than that of BSA (4.01). This is attributed to the use of H2O2 during the synthesis of the CuNCs, which partially destroys the chemical bonds in BSA and results in an enhanced exposure of hydrophilic groups capable of protonation. When the pH value is more than 2.36, the zeta potential of the CuNCs becomes negative, indicating a negative charge on the CuNC surface. The negative charge is beneficial for improving the stability of the CuNC solution due to electrostatic repulsion. Interestingly, the zeta potential (ζ) linearly increases with increasing pH value of the solution, in the range from 2 to 14. The linear equation is ζ (mV) = −2.513pH + 8.23 and the corresponding correlation coefficient is 0.9918. Thus, the pH value can be determined by measuring the zeta potential.
Fig. 8 presents the typical TEM images of the as-prepared CuNCs at different pH values. From Fig. 8, we can observe that the CuNCs have a narrow particle size distribution with an average diameter of 1.6 ± 0.5 nm at pH 12. However, the particle size of the CuNCs increases with decreasing pH value. When the pH value is close to 5, an obvious aggregate appears in the CuNCs. Interestingly, the aggregate will rapidly disappear if the pH value is adjusted to basic, verifying that the aggregation is a reversible process.
 |
| Fig. 8 TEM images of the CuNCs at pH 12 (a), 8 (b), 5 (c) and 3 (d). | |
Fig. 9 presents the ratio of secondary structure in the BSA in the CuNCs and CuNCs-c. The BSA in the CuNCs exhibits an obvious reduction in the amount of α-helix and an increase in unordered chains. This can be attributed to the use of H2O2 during the synthesis of the CuNCs. Many investigations have confirmed that H2O2 can be changed into ˙OH radicals under the catalysis of metal ions such as Cu2+.16 Due to its better oxidation ability compared with H2O2, ˙OH could partially destroy the peptide and disulfide bonds in BSA, resulting in a greater exposure of hydrophilic groups capable of protonation.17 During the formation of the CuNCs, the hydrophobic groups in BSA are embedded in the center of the structure and the hydrophilic groups are distributed at the surface of the CuNCs, which will improve the response of the CuNCs towards pH fluctuation in the surrounding environment.
 |
| Fig. 9 The secondary structure of CuNCs and CuNCs-c. | |
Based on the above analysis, we suggest a mechanism for the pH response. The pH response of the CuNCs originates from their reversible aggregation. At low pH values, the aggregation develops. This helps to transfer energy between the CuNCs due to the very small distance between them and results in a decrease in the fluorescence intensity. In addition, the protonation of BSA on the CuNC surface at low pH leads to partially quenched fluorescence due to the electron withdrawing effect. At high pH values, the aggregation is greatly suppressed due to electrostatic repulsion. More negatively charged ions such as OH− are adsorbed on the surface of the CuNCs. Their electron donating effect enhances the fluorescence intensity. More importantly, the use of H2O2 during the synthesis breaks up some of the peptide and disulfide bonds in BSA and results in increasing exposure of hydrophobic groups capable of protonation. This action creates an elaborate protonation/deprotonation system in the CuNCs, which improves the range and sensitivity of the response of the pH sensor towards pH value.
3.4 Detection of pH value in water samples
The pH-sensitive properties of the fluorescence emission of the CuNCs could be applied in the pH detection of bodies of water to check if the water is polluted or if it is safe to drink. Comparing the results from our method and the pH meter, as shown in Table 2, we can see that the results are reliable and the CuNCs can be utilized as an excellent fluorescent pH indicator for real water samples.
Table 2 The results for the detection of pH value in water samples (N = 5)
Sample |
The results obtained using the proposed method |
The results obtained using the pH meter |
River water |
7.33 ± 0.12 |
7.29 ± 0.18 |
Lake water |
6.90 ± 0.09 |
6.84 ± 0.13 |
Tap water |
6.82 ± 0.15 |
6.90 ± 0.22 |
Well water |
7.13 ± 0.05 |
7.09 ± 0.14 |
Rain water |
6.81 ± 0.08 |
6.92 ± 0.05 |
Distilled water |
7.04 ± 0.10 |
7.06 ± 0.16 |
3.5 Intracellular pH sensing via confocal microscopy
To further demonstrate the application of the probe in live cells, an intracellular calibration experiment was carried out in CuNC-loaded RBL-2H3 cells. In the process of intracellular pH sensing, the CuNCs have two roles: the signal molecule for pH sensing and the carrier for carrying itself as a single molecule into the cells. This would avoid any complicated modification processes. Fig. 10 shows the confocal images of RBL-2H3 cells treated with BR buffer. The CuNCs are well-distributed in the live cells, indicating excellent membrane permeability due to their small size. The CuNC-loaded cells exhibit a weak fluorescence in pH 5.7 BR buffer for the DAPI channel; nevertheless, the RBL-2H3 cells become brighter as the pH increases. The fluorescence intensity increases with pH from 5.1, 6.6, 7.2 to 8.0. All of these results establish that the obtained CuNCs are desirable pH probes for monitoring the pH changes in live cells.
 |
| Fig. 10 Epifluorescence microscopic images of RBL-2H3 cells treated with the CuNCs (20 μg ml−1) at pH 5.1 (a), 6.6 (b), 7.2 (c) and 8.0 (d) using a UV excitation of 365 nm. | |
4 Conclusions
We have successfully developed an ultra sensitive and wide-range pH sensor based on BSA-capped copper nanoclusters fabricated using a fast synthesis method through the use of hydrogen peroxide as an additive. Owing to the strong oxidizing capacity of hydrogen peroxide in the presence of Cu2+, a portion of the peptide and disulfide bonds in the BSA molecule were broken during the synthesis. This leads to an increased exposure of hydrophilic groups in the BSA molecule that are capable of protonation, helping to create a better protonation/deprotonation system for the copper nanoclusters compared with the synthesis in the absence of hydrogen peroxide. The proposed pH sensor exhibits an enhanced response towards pH fluctuation in the surrounding environment. The applications of the CuNCs for the pH detection in natural water and imaging in live RBL-2H3 cells were successfully achieved, proving that they can reveal intracellular pH fluctuations via a change in the fluorescence intensity response. This study also provides a promising candidate for application in biological, medical and pharmaceutical fields.
Acknowledgements
The authors acknowledge the financial support from the National Natural Science Foundation of China (no. 21176101), the Fundamental Research Funds for the Central Universities (no. JUSRP51314B), MOE & SAFEA for the 111 Project (B13025) and the country “12th Five-Year Plan” to support science and technology project (no. 2012BAK08B01).
Notes and references
- Z. Y. Yang, W. Qin, J. W. Y. Lam, S. Chen, H. H. Y. Sung, I. D. Williams and B. Z. Tang, Chem. Sci., 2013, 4, 3725 RSC
. - M. Weinlich, U. Heydasch, F. Mooren and M. Starlinger, Res. Exp. Med., 1998, 198, 73 CrossRef CAS
. - A. M. Dennis, W. J. Rhee, D. Sotto, S. N. Dublin and G. Bao, ACS Nano, 2012, 6, 2917 CrossRef CAS PubMed
. - H. R. Kermis, Y. Kostov, P. Harms and G. Rao, Biotechnol. Prog., 2002, 18, 1047 CrossRef CAS PubMed
. - X. F. Wu, R. Y. Li, Z. J. Li, J. K. Liu, G. L. Wang and Z. G. Gu, RSC Adv., 2014, 4, 24978 RSC
; X. F. Wu, R. Y. Li and Z. J. Li, RSC Adv., 2014, 4, 9935 RSC
. - Y. P. Zhong, C. Deng, Y. He, Y. L. Ge and G. W. Song, Anal. Methods, 2015, 7, 1558 RSC
; J. X. Dong, F. Qu, N. B. Li and H. Q. Luo, RSC Adv., 2015, 5, 6043 RSC
. - R. J. Stover, A. K. Murthy, G. D. Nie, S. Gourisankar, B. J. Dear, T. M. Truskett, K. V. Sokolov and K. P. Johnston, J. Phys. Chem. C, 2014, 118, 14291 Search PubMed
; W. C. Ding, Y. Liu, Y. J. Li, Q. R. Shi, H. S. Li, H. B. Xia, D. Y. Wang and X. T. Tao, RSC Adv., 2014, 4, 22651 RSC
. - W. Wang, F. Leng, L. Zhan, Y. Chang, X. X. Yang, J. Lan and C. Z. Huang, Analyst, 2014, 139, 2990 RSC
. - Y. L. Feng, Y. F. Liu, C. Su, X. H. Ji and Z. K. He, Sens. Actuators, B, 2014, 203, 795 CrossRef CAS PubMed
. - Y. N. Chen, P. C. Chen, C. W. Wang, Y. S. Lin, C. M. Ou, L. C. Ho and H. T. Chang, Chem. Commun., 2014, 50, 8571 RSC
. - P. C. Chen, J. Y. Ma, G. L. Lin, C. C. Shih, T. Y. Lin and H. T. Chang, Nanoscale, 2014, 6, 3503 RSC
. - F. Gu, N. B. Li and H. Q. Luo, Langmuir, 2013, 29, 1199 CrossRef PubMed
. - P.-C. Chen, C.-K. Chiang and H.-T. Chang, J. Nanopart. Res., 2013, 15, 1336 CrossRef
. - S. K. Cushing, M. Li, F. Q. Huang and N. Q. Wu, ACS Nano, 2014, 8, 1002 CrossRef CAS PubMed
. - C. Wang, C. X. Wang, L. Xu, H. Cheng, Q. Lin and C. Zhang, Nanoscale, 2014, 6, 1775 RSC
. - T. Sun, H. R. Guo, H. L. Xu and B. K. Zhou, Chem. J. Chin. Univ., 2007, 28, 856–858 CAS
. - M. M. Zou, Y. Li, J. Wang, J. Q. Gao, Q. Wang, B. X. Wang and P. Fan, Spectrochim. Acta, Part A, 2013, 112, 206–213 CrossRef CAS PubMed
.
Footnote |
† Electronic supplementary information (ESI) available. See DOI: 10.1039/c5ra07966g |
|
This journal is © The Royal Society of Chemistry 2015 |