DOI:
10.1039/C5RA07901B
(Communication)
RSC Adv., 2015,
5, 51834-51840
Synthesis, photophysical, electrochemical and laser properties of anthracene conjugated glycodendrimers with triazole as a bridging unit†
Received
29th April 2015
, Accepted 28th May 2015
First published on 28th May 2015
Abstract
Anthracene conjugated blue light emitting glycodendrimers 1, 2 and 3 were synthesized using Cu(I) catalyzed click chemistry. All of the anthracene conjugated blue light emitting glycodendrimers have enhanced photophysical, electrochemical and laser properties, from the lower to the higher generation dendrimers. Blue light emitting higher generation glycodendrimer 3 shows a longer life time (11.2 ns) than the lower generation glycodendrimers 1 and 2 due to the presence of a larger number of triazole bridging units and multivalent glucose units.
Introduction
The insertion of biologically important carbohydrate residues into dendritic scaffolds is widely used for biomedical applications.1 Glycodendrimers are supramolecular architectures having several carbohydrate residues on the surface accessible for multiple binding interactions, such as carbohydrate protein interactions which are essential for all living organisms, and they play a crucial role in many biological processes.2 Glycodendrimers have many more applications in the field of biology, because of their carbohydrate functionality, monodimensional size, multi-valance, biocompatibility, reduced toxicity, chiral surface for asymmetric induction,3 cell recognition,4 cell–protein interactions,5 targeting of hormones, antibodies and toxins,6 carbohydrate binding with proteins or lectins7 and as non-steroidal anti-inflammatory8 and antioxidant drugs.9
Anthracene based hybrids show strong luminescent behavior and have been widely used as blue-emitting materials in Organic Light Emitting Diodes (OLEDs) due to their excellent photoluminescence (PL) and electroluminescence (EL) properties, as well as excellent stability.10 Anthracene is an important building block for constructing blue luminescent dyes due to its merits, such as thermal and electrochemical stability.11 Anthracene hybrids with sugar molecules are widely used in biological and material applications, such as deoxyribonucleic acid (DNA) cleavage,12 artificial light activatable protein cleaving agents,13 carbohydrate selectors,14 and the recognition of various metal anions such as F−, Cl− and, Br− as well as cations such as alkali, alkaline-earth and heavy-metal ions.15 Anthracene attached dendrimers exhibit a blue fluorescence and hence will have potential applications as light emitting diodes and fuel cells, biomimetic catalysts, sensors and efficient light harvesting antenna devices.
The development of new materials containing photoactive units in a dendritic fashion has gained much interest in the last two decades due to their attractive physical properties for molecular electronics.16 Developing photoactive units containing carbohydrate molecules, which are promising candidates for various applications like bioimaging, cancer cell metastasis, cellular trafficking, inflammation by bacteria and viruses and the immune response and material properties, has been the focal point of recent interest. With such an interesting idea in mind, we report herein the synthesis of anthracene conjugated glycodendrimers 1, 2 and 3 with triazole as a bridging unit (Fig. 1), and their photophysical, electrochemical and laser properties.
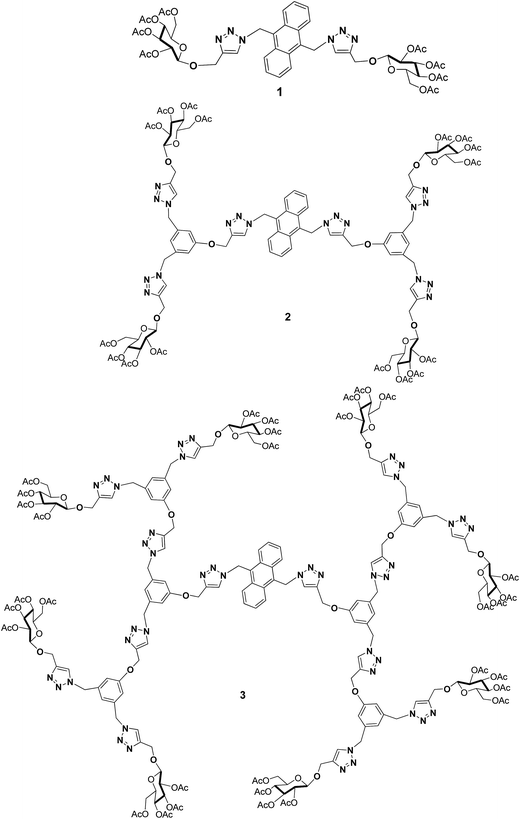 |
| Fig. 1 Structures of the glycodendrimers 1, 2 and 3. | |
The anthracene cored glycodendrimers are synthesized using a click chemistry technique17 by the ligation of an azide to an alkyne in the presence of a Cu(I) salt to form triazole bridges. The triazole linkers present in the dendritic systems have biological and material properties, which enhance the applications of the glycodendrimers.
Results and discussion
The synthesis of the first generation propargyloxy dendritic wedge 7 and the second generation dendritic wedge 9 is shown in Scheme 1. The reaction of 2.1 equiv. of propargyloxy glucose acetate 418 with 1.0 equiv. of 3,5-bis(azidomethyl)phenol 519 under click chemistry reaction conditions afforded the hydroxy dendron 6 in 86% yield, which was further treated with 1.25 equiv. of propargyl bromide in the presence of K2CO3 in DMF at room temperature to give the first generation propargyloxy dendron 7 in 72% yield. The 1H NMR spectrum of propargyloxy dendron 7 showed four singlets at δ 1.93, 1.99, 2.03 and 2.08 for four different glyco-acetoxy protons, a sharp singlet at δ 5.49 for the benzylic protons, and a sharp singlet at δ 7.52 for the triazolyl protons. The 13C NMR spectrum of 7 displayed a benzylic carbon peak at δ 53.6 and a triazolyl carbon peak at δ 144.8. The appearance of the molecular ion peak at m/z 1014 [M+] in the mass spectrum confirmed the structure of the first generation propargyloxy dendron 7. The structure of the first generation propargyloxy dendron 7 was also confirmed from elemental analysis.
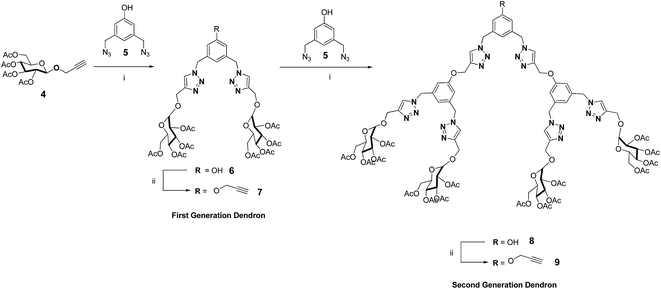 |
| Scheme 1 Reagents and conditions: (i) CuSO4 (5 mol%), sodium ascorbate (10 mol%), THF–H2O (1 : 1), r.t., 12 h, 6 (86%) and 7 (82%); (ii) 1.25 equiv. propargyl bromide, K2CO3, DMF, r.t., 48 h, 8 (72%) and 9 (79%). | |
The second generation propargyloxy dendron 9 was obtained from the hydroxyl dendron 8. The reaction of 2.1 equiv. of the propargyloxy dendron 7 with 1.0 equiv. of 3,5-bis(azidomethyl)phenol 5 under Cu(I)-catalyzed click reaction conditions gave the hydroxy dendron 8 in 82% yield. Reaction of the hydroxy dendron 8 with 1.25 equiv. of propargyl bromide in the presence of K2CO3 in DMF at room temperature gave the second generation propargyloxy dendron 9 in 79% yield. The 1H NMR spectrum of propargyloxy dendron 9 showed four singlets at δ 1.83, 1.91, 1.95 and 1.99 for four different glyco-acetoxy protons, and a sharp singlet at δ 7.46 for triazolyl protons. The 13C NMR spectrum of 9 displayed the triazolyl carbon peak at δ 143.7 and four different glyco-acetoxy carbonyl carbon peaks at 168.3, 168.4, 169.1 and 169.6 respectively. The appearance of the molecular ion peak at m/z 2270 [M+] in the mass spectrum confirmed the structure of the second generation propargyloxy dendron 9. The structure of the glycodendrimer 9 was also confirmed from elemental analysis.
The blue light emitting glycodendrimers 1, 2 and 3 with anthracene as a core unit from the corresponding dendritic wedges were synthesized as shown in Scheme 2.
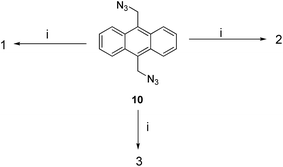 |
| Scheme 2 Reagents and conditions: (i) 2.1 equiv. of 4, 7 and 9, CuSO4 (5 mol%), sodium ascorbate (10 mol%), THF–H2O (1 : 1), r.t., 12 h, 1 (89%), 2 (80%) and 3 (79%). | |
Reaction of 9,10-bis(chloromethyl)anthracene20 with NaN3 in a mixture of acetone and water at 60 °C for 8 h gave 9,10-(bisazidomethyl)anthracene 10 in 76% yield. The reaction of 1.0 equiv. of 9,10-(bisazidomethyl)anthracene 10 with 2.1 equiv. of the propargylated glucose acetate 4 in the presence of Cu(I)-catalyzed click reaction conditions afforded glycodendrimer 1 in 89% yield (Scheme 2). The functionalization of 1.0 equiv. of 9,10-(bisazidomethyl)anthracene 10 with 2.1 equiv. of propargylated glucose dendron 7 under click reaction conditions gave glycodendrimer 2 in 80% yield. The 1H NMR spectrum of first generation glycodendrimer 2 displayed four singlets at δ 1.88, 1.98, 2.02 and 2.04 for four different glyco-acetoxy protons, a sharp singlet at δ 5.41 for Ph-CH2 protons, and sharp singlets at δ 7.37 and δ 7.52 for two different triazolyl protons. The 13C NMR spectrum of 2 displayed triazolyl carbon peaks at δ 143.3 and δ 144.7 and four different glyco-acetoxy carbonyl carbon peaks at δ 169.4, 169.5, 170.1 and 170.6. The appearance of the molecular ion peak at m/z 2318 [M+] in the mass spectrum confirmed the structure of the first generation glycodendrimer 2. The structure of glycodendrimer 2 was also confirmed from elemental analysis. Similarly, the functionalization of 1.0 equiv. of 9,10-(bisazidomethyl)anthracene 10 with 2.1 equiv. of propargylated glucose dendron 9 under click reaction conditions gave glycodendrimer 3 in 79% yield. The 1H NMR spectrum of second generation glycodendrimer 3 displayed four singlets at δ 1.89, 1.97, 2.01 and 2.04 for four different glyco-acetoxy protons, and sharp singlets at δ 7.46 and δ 7.54 for two different triazolyl protons. The 13C NMR spectrum of 3 displayed the triazolyl carbon peak at δ 144.6 and three different glyco-acetoxy carbonyl carbon peaks at δ 169.5, 170.1 and 170.6. The appearance of the molecular ion peak at m/z 4232 [M+] in the mass spectrum confirmed the structure of the first generation glycodendrimer 3. The structure of glycodendrimer 3 was also confirmed from elemental analysis.
Optical, photophysical and laser properties of the anthracene cored blue light emitting glycodendrimers 1, 2 and 3
The photophysical and optical properties of the anthracene cored blue light emitting glycodendrimers 1, 2 and 3 are presented in Table 1. The absorption spectra (1 × 10−4 M) and fluorescence spectra (1 × 10−4 M) of the anthracene cored glycodendrimers 1, 2 and 3 in DCM are shown in Fig. 2. The electronic absorption spectra of glycodendrimers 1, 2 and 3 show three intense absorption bands between 350–398 nm due to the π–π* transitions of the anthracene core. Fluorescein cored glycodendrimers 1, 2 and 3 when excited at 378 nm gave an emission band at 391–438 nm due to the presence of a greater number of triazolyl units and anthracene fluorophore unit. The fluorescence intensity of the blue light emitting glycodendrimers increases as the dendrimer generation increases, which is consistent with the increased number of bridging triazolyl units along with a greater number of anthracene fluorophore unit, which is otherwise known as the valency effect in dendrimer chemistry.21 The triazole and glucose units in the dendrimer shows a cumulative effect on the anthracene chromophore unit of the dendrimer and this probably increases the intensity of the signal.
Table 1 Optical, electrochemical and laser parameters for the dendrimers 1, 2 and 3 in concentrations of 1 × 10−4 M in DCM
Entry no. |
λabsmax (nm) |
λemmax (nm) |
Cyclic voltammetry |
Life time |
Epc |
Epa |
(τ) |
χ2 |
1 |
350, 378 & 398 |
391, 412 & 437 |
1.12, 1.70 |
−0.96, −1.30 |
9.29 |
1.15 |
2 |
356, 375 & 396 |
392, 413 & 438 |
1.13, 1.79 |
−1.32 |
11.04 |
1.16 |
3 |
354, 376 & 397 |
391, 412 & 437 |
1.21, 1.80 |
−1.38 |
11.28 |
1.19 |
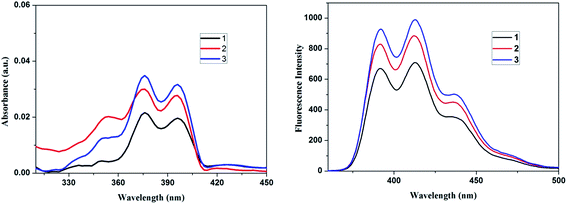 |
| Fig. 2 Absorption and emission spectra of the anthracene cored glycodendrimers 1, 2 and 3. | |
Life time decay analysis was carried out on all of the blue light emitting glycodendrimers 1, 2 and 3, using Iglesia Bautista Horeb (IBH), a Time-Correlated Single Photon Counting (TCSPC) technique, on excitation at 375 nm in dichloromethane (DCM) solvent. The fluorescence decay fits as mono-exponential with life time τ1. The life time decay values of the anthracene cored glycodendrimers 1, 2 and 3 are τ1 9.29, τ1 11.04 and τ1 11.18, respectively (Table 1). The fluorescence decay values (Fig. 3) of dendrimers 2 and 3 show a longer relaxation time τ1 than that of dendrimer 1. However, the fluorescence life time increases as the generation growth of the dendrimer increases which could be due to the greater number of triazolyl, anthracene and glucose units in the higher generation dendrimers.
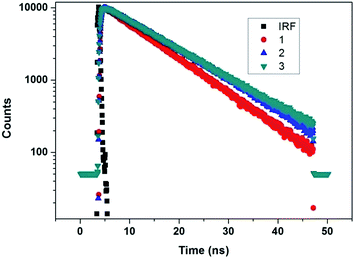 |
| Fig. 3 Fluorescence decay of glycodendrimers 1, 2 and 3 (IRF = instrument response function). | |
Electrochemical properties of the anthracene cored blue light emitting glycodendrimers 1, 2 and 3
The electrochemical behaviour of the anthracene cored blue light emitting glycodendrimers 1, 2 and 3 was studied by cyclic voltammetry in 0.1 M of tetrabutylammonium perchlorate (TBAP) as a supporting electrolyte in dry DCM, as shown in Fig. 4. According to the cyclic voltammograms, anodic and cathodic peak potential responses are observed for all of the glycodendrimers, corresponding to electrochemical oxidation and reduction processes. Glycodendrimer 1 shows two oxidation potentials at 1.12 and 1.70, as well as two reduction peaks exhibiting negative potentials at −0.96 and −1.30. Similarly, glycodendrimers 2 and 3 exhibit oxidation peaks at 1.13, 1.79 and 1.21, 1.80, and reduction peaks with negative potentials at −1.32 and −1.38, respectively. From this study, the electrochemical properties are shown to alter upon increasing the dendrimer generation from a lower to a higher. Glycodendrimers 2 and 3 show only one reduction wave compared to glycodendrimer 1. Oxidation is fast and reduction is slow in higher generation dendrimers because of the excessive crowding due to the greater number of triazolyl and glucose units.
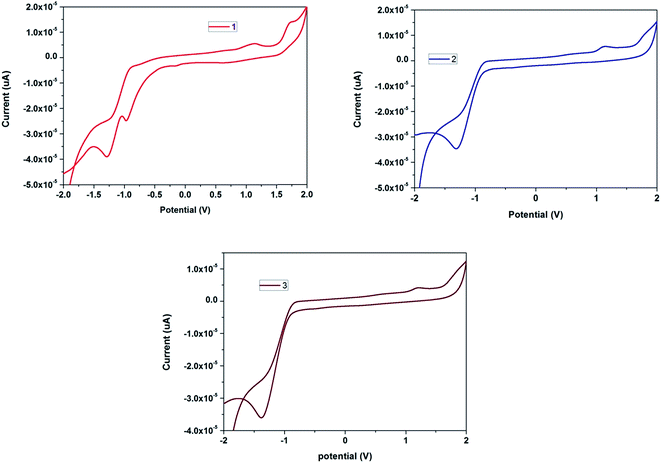 |
| Fig. 4 Cyclic voltammograms of the blue light emitting glycodendrimers 1, 2 and 3 in DCM at room temperature (scan rate at 50 mV s−1) using 0.1 M TBAP as a supporting electrolyte in dry DCM. | |
Experimental
All reagents were commercially available and used as such unless otherwise stated. Analytical TLC was performed on commercial Merck plates coated with Silica Gel GF254. Analytical samples were obtained from silica gel chromatography, using silica gel of 100–200 mesh and elution with the solvent system as mentioned under each experiment section. The melting points were determined using a Metler Toledo melting point apparatus by open capillary tube method and were uncorrected. 1H and 13C NMR spectra were recorded on a 300 MHz BRUKER AVANCE (75 MHz for 13C NMR) spectrometer. Chemical shift values are reported in δ ppm relative to the internal standard tetramethylsilane (TMS, δ 0.00). 13C chemical shifts (ppm) are reported in δ relative to CDCl3 (center of triplet, δ 77.23). The spin multiplicities are indicated by the symbols s (singlet), d (doublet), t (triplet), q (quartet), m (multiplet) and br (broad), and dd (doublet of doublets). UV-vis absorption spectra were measured using a Perkin-Elmer Lambda 35 UV-vis spectrometer. All of the electrochemical measurements were performed using a PGSTAT-12 electrochemical work station, (AUTOLAB, The Netherlands BV) controlled by a general purpose electrochemical system (GPES). The measurements were based on a three electrode system, with a glassy carbon (GC) electrode (of geometric area 0.07 cm2) used as the working electrode, a Pt wire in the form of a spiral (with a high geometrical surface area of 20 cm2) used as the auxiliary electrode and Ag/AgCl as the reference electrode with a scan rate of 50 mV s−1 and a supporting electrolyte of 0.1 M TBAP.
General procedure for the Cu-catalyzed Huisgen ‘click reaction’
A mixture of azide (1.0 mmol, 1.0 equiv.), propargyl glucopyranoside (2.1 mmol, 2.1 equiv.), CuSO4·5H2O (5 mol%) and NaAsc. (10 mol%) in a tetrahydrofuran–water (THF–H2O) (1
:
1, 20 mL) mixture was stirred for 12 h at room temperature. The residue obtained after evaporation of the solvent was dissolved in CHCl3 (150 mL) and washed with NH4Cl solution (50 mL) and brine solution (50 mL), dried over Na2SO4 and then concentrated to give a residue, which was purified by column chromatography (SiO2), using the eluent as mentioned under each compound.
First generation OH dendron 6
The reaction of 1,3-bis(azidomethyl)phenol 5 (1.0 mmol, 1 equiv.) with propargylated D-glucose 4 (2.0 mmol, 2.1 equiv.) using a general procedure for click chemistry afforded the first generation phenolic dendron 6 as a white solid; yield: 82%; 1H NMR (300 MHz, CDCl3) δH 1.92, 1.99, 2.03, 2.07 (4 × s, 24H), 3.73–3.76 (m, 2H), 4.12–4.26 (m, 4H), 4.67–4.81 (m, 4H), 4.89–4.99 (m, 4H), 5.05–5.11 (m, 2H), 5.27–5.23 (m, 2H), 5.43 (s, 4H), 6.65 (s, 3H), 7.67 (s, 2H); 13C NMR (75 MHz, CDCl3) δC 20.6, 20.7, 53.8, 61.8, 62.9, 68.3, 71.3, 71.9, 72.7, 100.1, 115.3, 118.3, 123.4, 137.1, 144.6, 158.6, 169.5, 169.6, 170.2, 170.8 ppm. MS(EI): m/z 976 (M+). Elemental anal. calcd for C42H52N6O21: C, 51.64; H, 5.37; N, 8.60; O, 34.39%. Found: C, 51.34; H, 5.47; N, 8.70; O, 34.19%.
First generation alkyne dendron 7
The first generation phenolic dendron 6 (1.0 mmol, 1.0 equiv.) was reacted with propargyl bromide (1.2 mmol, 1.2 equiv.) in the presence of K2CO3 in dry DMF for 12 h to afford the alkyne dendron 7 as a white powder in 86% yield. 1H NMR (300 MHz, CDCl3) δH 1.93, 1.99, 2.03, 2.08 (4 × s, 24H), 2.56 (s, 1H), 3.73–3.76 (m, 2H), 4.12–4.23 (m, 2H), 4.21–4.28 (m, 2H), 4.66–4.69 (m, 4H), 4.79–4.84 (m, 2H), 4.91–5.01 (m, 4H), 5.05–5.17 (m, 2H), 5.20–5.23 (m, 2H), 5.49 (s, 4H), 6.82 (s, 3H), 7.52 (s, 2H); 13C NMR (75 MHz, CDCl3) δC 20.6, 20.7, 53.6, 55.9, 61.8, 63.4, 68.4, 71.3, 72.74, 76.6, 77.6, 100.0, 114.8, 120.2, 122.9, 137.2, 144.8, 158.5, 169.3, 169.4, 170.2, 170.6 ppm. MS(EI): m/z 1014 (M+). Elemental anal. calcd for C45H54N6O21: C, 53.25; H, 5.36; N, 8.28; O, 33.10%. Found: C, 53.45; H, 5.66; N, 8.18; O, 33.30%.
Second generation OH dendron 8
The reaction of 1,3-bis(azidomethyl)phenol 5 (1.0 mmol, 1 equiv.) with first generation alkyne dendron 7 (2.0 mmol, 2.1 equiv.) using a general procedure for click chemistry afforded the second generation phenolic dendron 8 as a semi-solid; yield: 72%; 1H NMR (300 MHz, CDCl3): δH 1.92, 1.99, 2.03, 2.07 (4 × s, 48H), 3.74–3.77 (m, 4H), 4.14–4.29 (m, 6H), 4.69–4.71 (m, 4H), 4.81–4.85 (m, 4H), 4.91 (s, 4H), 4.96–5.02 (m, 6H), 5.06–5.12 (m, 4H), 5.19–5.25 (m, 8H), 5.44 (s, 12H), 6.76 (s, 3H), 6.79 (s, 6H), 7.46 (s, 2H) 7.58 (s, 4H), 8.68 (s, 1H); 13C NMR (75 MHz, CDCl3): δC 19.6, 19.7, 28.7, 52.6, 60.8, 61.0, 61.9, 67.4, 70.3, 70.9, 71.8, 99.0, 113.8, 115.9, 117.5, 119.0, 122.3, 135.4, 135.9, 136.2, 142.6, 143.6, 157.2, 157.5, 158.1, 168.5, 169.2, 169.7 ppm. MS (Q-TOF): m/z 2233 (M+). Elemental anal. calcd for C98H116N18O43: C, 52.69; H, 5.23; N, 11.29%. Found: C, 52.63; H, 5.19; N, 11.24%.
Second generation alkyne dendron 9
The second generation phenolic dendron 8 (1.0 mmol, 1.0 equiv.) was reacted with propargyl bromide (1.2 mmol, 1.2 equiv.) in the presence of K2CO3 in dry DMF for 12 h to afford the alkyne dendron 9 as a semi-solid, 79% yield. 1H NMR (300 MHz, CDCl3): δH 1.83, 1.91, 1.95, 1.99 (4 × s, 48H), 2.44 (s, 1H) 3.65–3.69 (m, 4H), 4.00–4.19 (m, 8H), 4.56–4.75 (m, 10H), 4.82–5.16 (m, 20H), 5.39 (s, 12H), 6.70 (s, 3H), 6.76 (s, 6H), 7.46 (s, 4H) 7.53 (s, 2H); 13C NMR (75 MHz, CDCl3): δC 19.6, 19.7, 21.7, 28.7, 52.5, 55.0, 60.8, 62.0, 67.4, 70.3, 71.8, 75.6, 76.4, 76.7, 99.1, 113.1, 113.7, 118.9, 122.1, 122.3, 127.8, 129.9, 136.2, 138.3, 143.7, 157.5, 158.1, 168.3, 168.4, 169.1, 169.6 ppm. MS (Q-TOF): m/z 2270 (M+). Elemental anal. calcd for C101H118N18O43: C, 53.39; H, 5.23; N, 11.10%. Found: C, 53.34; H, 5.19; N, 11.03%.
9,10-Diazidomethylanthracene 10
Yield: 76%; m.p. 150–152 °C; 1H NMR (300 MHz, CDCl3): δH 5.36 (s, 4H), 7.67–7.62 (m, 4H), 8.40–8.35 (m, 4H); 13C NMR (75 MHz, CDCl3): δC 46.3, 124.6, 126.7, 128.6, 130.2 ppm. MS (ESI): m/z 288 (M+). Elemental anal. calcd for C16H12N6: C, 66.66; H, 4.20; N, 29.15%. Found: C, 66.62; H, 4.17; N, 29.08%.
Glycodendrimer 1
Glycodendrimer 1 was synthesized as a light brown solid from 9,10-diazidomethylanthracene 10 (1.0 mmol, 1.0 equiv.) with propyn-1-yl 2,3,4,6-tetra-O-acetyl-b-D-glucopyranoside 4 (2.0 mmol, 2.1 equiv.) using click chemistry; yield: 89%; m.p. 94–96 °C; Rf = 0.46 (CHCl3
:
methanol, 49
:
1); 1H NMR (300 MHz, CDCl3): δH 1.96, 1.99, 2.00, (3 × s, 24H), 3.58–3.63 (m, 2H) 3.98–4.18 (m, 4H), 4.54–4.56 (m, 2H), 4.65–4.77 (m, 4H), 4.82–4.87 (m, 2H), 4.96–4.99 (m, 2H), 5.02–5.13 (m, 2H), 6.59 (s, 4H), 7.18 (s, 2H), 7.67–7.78 (m, 4H), 8.44–8.47 (m, 4H); 13C NMR (75 MHz, CDCl3): δC 20.6, 20.7, 22.7, 29.7, 53.6, 61.9, 63.0, 68.4, 71.3, 71.9, 72.8, 76.6, 77.0, 77.5, 100.0, 114.7, 119.9, 123.3, 130.7, 137.3, 144.6, 159.0, 169.5, 170.1, 170 ppm. MS (MALDI-TOF): m/z 1060 (M+). Elemental anal. calcd for C50H56N6O20: C, 56.60; H, 5.32; N, 7.92%. Found: C, 56.53; H, 5.28; N, 7.86%.
Glycodendrimer 2
Glycodendrimer 2 was synthesized as a light yellowish solid from 9,10-diazidomethylanthracene 10 (1.0 mmol, 1.0 equiv.), and first generation alkyne dendron 7 (2.1 mmol, 2.1 equiv.) using click chemistry; yield: 80%; m.p. 118–120 °C; Rf = 0.54 (CHCl3
:
methanol, 19
:
1); 1H NMR (300 MHz, CDCl3): δH 1.88, 1.98, 2.02, 2.04, (4 × s, 48H), 3.74–3.77 (m, 4H), 4.11–4.27 (m, 8H), 4.67–4.70 (m, 4H), 4.77–4.81 (m, 4H), 4.88–5.23 (m, 20H), 5.41 (s, 8H), 6.61 (s, 4H), 6.73 (s, 2H), 6.77 (s, 4H), 7.37 (s, 2H), 7.52 (s, 4H), 7.67–7.69 (m, 4H) 8.47–8.49 (m, 4H); 13C NMR (75 MHz, CDCl3): δC 20.6, 20.7, 29.7 46.6, 53.5, 61.8, 63.0, 71.3, 71.9, 72.8, 76.7, 77.1, 77.6, 100.0, 114.6, 119.9, 12.8, 123.1, 124.3, 127.7, 130.7, 143.3, 144.7, 159.1, 169.4, 169.5, 170.1, 170.6 ppm. MS (MALDI-TOF): m/z 2318 (M+). Elemental anal. calcd for C106H120N18O42: C, 54.92; H, 5.22; N, 10.88%. Found: C, 54.87; H, 5.18; N, 10.82%.
Glycodendrimer 3
Glycodendrimer 3 was synthesized as a light brown solid from 9,10-diazidomethylanthracene 10 (1.0 mmol, 1.0 equiv.), and second generation alkyne dendron 9 (2.1 mmol, 2.1 equiv.) using click chemistry; yield: 79%; m.p. 131–133° C; 1H NMR (300 MHz, CDCl3): δH 1.89, 1.97, 2.01, 2.04, (4 × s, 96H), 3.74–3.76 (m, 8H), 4.08–4.15 (m, 8H), 4.22–4.27 (m, 8H), 4.67–4.70 (m, 12H), 4.75–4.79 (m, 6H), 4.83–4.90 (m, 6H), 4.93–4.98 (m, 12H), 5.06–5.09 (m, 12H), 5.17–5.23 (m, 12H), 5.40 (s, 16H) 5.45 (s, 8H), 6.62 (s, 4H), 6.75 (s, 6H), 6.81 (s, 12H), 7.40 (s, 2H), 7.54 (s, 8H), 7.60 (s, 4H), 7.61–7.64 (m, 4H), 8.42–8.49 (m, 4H). 13C NMR (75 MHz, CDCl3): δC 20.6, 20.7, 22.7, 29.7, 53.6, 61.9, 63.0, 68.4, 71.3, 71.6, 72.8, 76.6, 77.0, 77.5, 100.0, 114.7, 119.9, 123.3, 130.7, 144.6, 159.0, 169.5, 170.1, 170.6 ppm. MS (MALDI-TOF): m/z 4232 (M+). Elemental anal. calcd for C218H248N42O86: C, 54.18; H, 5.17; N, 12.17%. Found: C, 54.12; H, 5.12; N, 12.13%.
Conclusion
In conclusion, the blue light emitting glycodendrimers 1, 2 and 3 were successfully synthesized in excellent yield by a Cu(I) catalyzed click reaction and the higher generation glycodendrimers show enhanced optical, photophysical, laser and electrochemical properties than those of the lower generation dendrimers. The higher generation dendrimers also show longer life time decay and hence, the synthesis of such dendrimers and their applications for bioimaging in human live cell lines are under way.
Acknowledgements
The author thanks the Council of Scientific and Industrial Research (CSIR), New Delhi, India, for financial assistance and the Department of Science and Technology-Fund for the Improvement of Science and Technology Infrastructure (DST-FIST) for providing NMR facility to our department. AK thanks CSIR, New Delhi, for a fellowship and the author thanks the National Centre for Ultrafast Processes-Taramani for light amplification by stimulated emission of radiation (LASER) studies.
References
- R. Roy, T. C. Shiao and K. R. Olson, Braz. J. Med. Biol. Res., 2013, 49, 85 CAS.
-
(a) Y. Yang, M. Yu, T. T. Yan, Z. H. Zhao, Y. L. Sha and Z. J. Li, Bioorg. Med. Chem., 2010, 18, 5234 CrossRef CAS PubMed;
(b) N. Jayaraman, Chem. Soc. Rev., 2009, 38, 3463 RSC.
- N. Malik, R. Wiwattanapatapee, R. Klopsch, K. Lorenz, H. Frey, J. W. Weener, E. W. Meijer, W. Paulus and R. Duncan, J. Controlled Release, 2000, 65, 133 CrossRef CAS.
-
(a) K. J. Doores, D. P. Gamblin and B. G. Davis, Chem.–Eur. J., 2006, 12, 656 CrossRef CAS PubMed;
(b) G. A. Rabinovich, A. Cumashi, G. A. Bianco, D. Ciavardelli, I. Iurisci, M. Degidio, E. Piccolo, N. Tinari, N. Nifantiev and S. Iacobelli, Glycobiology, 2006, 16, 210 CrossRef CAS PubMed.
-
(a) T. Fukuda, S. Onogi and Y. Miura, Thin Solid Films, 2009, 518, 880 CrossRef CAS PubMed;
(b) N. Seah, P. V. Santacroce and A. Basu, Org. Lett., 2009, 11, 559 CrossRef CAS PubMed.
- M. G. Baek, K. R. Olson and R. Roy, Chem. Commun., 2001, 257 RSC.
-
(a) R. Kikkeri, F. Kamena, T. Gupta, L. H. Hossain, S. Boonyarattanakalin, G. Gorodyska, E. Beurer, G. Coullerez, M. Textor and P. H. Seeberger, Langmuir, 2010, 26, 1520 CrossRef CAS PubMed;
(b) I. Deguise, D. Lagnoux and R. Roy, New J. Chem., 2007, 31, 1321 RSC.
- P. Rajakumar and R. Anandhan, Eur. J. Med. Chem., 2011, 46, 4687 CrossRef CAS PubMed.
-
(a) A. R. Han and Y. S. J. Paik, J. Appl. Biol. Chem., 2012, 55, 195 CrossRef CAS;
(b) J. Wang, Y. D. Yue, F. Tang and J. Sun, Molecules, 2012, 17, 12297 CrossRef CAS PubMed.
-
(a) E. J. Na, K. H. Lee, B. Y. Kim, S. J. Lee, Y. K. Kim and S. S. Yoon, Mol. Cryst. Liq. Cryst., 2013, 584, 113 CrossRef CAS PubMed;
(b) M. Kato, A. Omura, A. Toshikawa, S. Kishi and Y. Sugumoto, Angew. Chem., 2002, 114, 3315 (Angew. Chem. Int. Ed., 2002, 41, 3183) CrossRef;
(c) Z. Fei, N. Kocher, C. J. Mohrschladt, H. Ihmels and D. Stalke, Angew. Chem., 2003, 115, 807 (Angew. Chem. Int. Ed., 2003, 42, 783) CrossRef PubMed;
(d) J. Seo, S. Kim and S. Y. Park, J. Am. Chem. Soc., 2004, 126, 11154 CrossRef CAS PubMed.
- S. H. Kim, I. Cho, M. K. Sim, S. Park and S. Y. Park, J. Mater. Chem., 2011, 21, 9139 RSC.
- K. Toshima, M. Hasegawa, J. Shimizu and S. Matsumura, ARKIVOC, 2004, 13, 28 CrossRef.
- A. Suzuki, M. Hasegawa, M. Ishii, S. Matsumura and K. Toshima, Bioorg. Med. Chem. Lett., 2005, 15, 4624 CrossRef CAS PubMed.
- M. Nishibu, D. Takahashi and K. Toshima, Org. Biomol. Chem., 2012, 10, 8393 CAS.
-
(a) P. Nanjappan and A. W. Czarnik, J. Am. Chem. Soc., 1987, 109, 1826 CrossRef CAS;
(b) M. E. Huston, K. W. Haider and A. W. Czarnik, J. Am. Chem. Soc., 1988, 110, 4460 CrossRef CAS;
(c) S. A. Van Arman and A. W. Czarnik, J. Am. Chem. Soc., 1990, 112, 5376 CrossRef CAS;
(d) L. Fabbrizzi, M. Licchelli, F. Mancin, M. Pizzeghello, G. Rabaioli, A. Taglietti, P. Tecilla and U. Tonellato, Chem.–Eur. J., 2002, 8, 94 CrossRef CAS.
-
(a) A. Adronov and J. M. J. Frechet, Chem. Commun., 2000, 1701 RSC;
(b) A. Mishra, C. Q. Ma, R. A. J. Janssen and P. Bauerle, Chem.–Eur. J., 2009, 15, 13521 CrossRef CAS PubMed;
(c) J. H. Huang, J. H. Su and H. Tian, J. Mater. Chem., 2012, 22, 10977 RSC;
(d) C. H. Wu, C. H. Chien, F. M. Hsu, P. I. Shih and C. F. Shu, J. Mater. Chem., 2009, 19, 1464 RSC.
-
(a) P. Rajakumar, A. Kannan and R. Anandhan, New J. Chem., 2014, 38, 1594 RSC;
(b) P. Rajakumar, R. Anandhan and A. Kannan, Aust. J. Chem., 2012, 65, 1457 CrossRef CAS;
(c) A. Thirunarayanan, S. Raja, G. Mohanraj and P. Rajakumar, RSC Adv., 2014, 4, 41778 RSC.
- L. F. Tietze and U. Bothe, Chem.–Eur. J., 1998, 4, 1179 CrossRef CAS.
- P. Rajakumar, S. Raja, C. Satheeshkumar, S. Ganesan, P. Maruthamuthu and S. A. Suthanthiraraj, New J. Chem., 2010, 34, 2247 RSC.
- W. Liu, Y. Wang, L. Bu, J. Li, M. Sun, D. Zhang, M. Zheng, C. Yang, S. Xue and W. Yang, J. Lumin., 2013, 143, 50 CrossRef CAS PubMed.
- T. H. Kwon, M. K. Kim, J. Kwon, T. Y. Shin, S. J. Park, C. L. Lee, J. J. Kim and J. I. Hong, Chem. Mater., 2007, 19, 3673 CrossRef CAS.
Footnote |
† Electronic supplementary information (ESI) available: The 1H NMR and 13C NMR spectra. See DOI: 10.1039/c5ra07901b |
|
This journal is © The Royal Society of Chemistry 2015 |