DOI:
10.1039/C5RA07812A
(Paper)
RSC Adv., 2015,
5, 61623-61630
Synthesis, characterization and photocatalytic activity of magnetically separable γ-Fe2O3/N,Fe codoped TiO2 heterojunction for degradation of Reactive Blue 4 dye
Received
29th April 2015
, Accepted 23rd June 2015
First published on 23rd June 2015
Abstract
In the present work, nanocrystalline undoped, N-doped and N,Fe codoped TiO2 have been synthesized using a sol–gel method for the photocatalytic degradation of Reactive Blue 4 dye under visible light, with N,Fe codoped TiO2 exhibiting the best activity. Furthermore, to counter the problem of separation of the N,Fe codoped TiO2 photocatalyst, magnetically separable γ-Fe2O3/N,Fe codoped TiO2 heterojunctions were fabricated and found to exhibit enhanced photoactivity with 100% dye degradation, compared to 85% with N,Fe codoped TiO2. This behavior has been attributed to the facilitated electron–hole transfer in the γ-Fe2O3/N,Fe codoped TiO2 heterojunctions, low electron–hole recombination rate, existence of a pure anatase phase and narrow band gap of 1.5 eV. The recycled γ-Fe2O3/N,Fe codoped TiO2 also demonstrated good repeatability of photoactivity. The mechanism involved in the degradation of dye by γ-Fe2O3/N,Fe codoped TiO2 has also been discussed. In conclusion, the synthesis of magnetically separable and visible light active titania by the coupling of γ-Fe2O3 and N,Fe codoped TiO2 to overcome the limitations of a narrow band gap and non-recyclability, with the additional advantage of enhanced photocatalytic activity for the degradation of Reactive Blue 4 dye, has been achieved.
1. Introduction
Semiconductor photocatalysts, mainly TiO2, have attracted much attention in the last decade for the removal of organic and inorganic pollutants1–4 because of their high activity, chemical stability, commercial availability and non-toxicity. However, the use of TiO2 inevitably leads to two obstacles when applied in practice, which are its narrow light response and difficult separation.5 Therefore, the development of a visible light active (VLA) and magnetically separable photocatalyst has become an important topic of research in this field.
The doping of non-metals or metals into TiO2 (ref. 6–11) has resulted in efficient photoactivity in the visible region. Recently, TiO2 codoped with non-metals and metals has been investigated, and the products have been found to be even more promising for making TiO2 visible light responsive.12,13 Among the various combinations of non-metal and metal codoping, nitrogen and iron codoping has received considerable attention because of their interesting synergistic effect in depressing the electron–hole recombination rate.14,15
An approach to overcome the separation problem is the synthesis of a photocatalyst that incorporates magnetic nanoparticles, due to the ease of their removal by the application of an external magnetic field.16 Unfortunately, sometimes photo-dissolution of the catalyst may occur, leading to the separation of TiO2 from the magnetic iron oxide core, which not only changes the properties of the magnetic iron oxide but also decreases the photocatalytic activity.17,18 Several papers in the literature have reported the synthesis of TiO2-based magnetic photocatalysts19,20 but research on the synthesis of magnetically separable VLA titania is still in its infancy and very few papers with magnetically separable non-metal or metal doped TiO2 have been reported.21–23 Yu et al. recently reported magnetically separable N,La codoped TiO2, which is the first report on the synthesis of non-metal and metal codoped TiO2.24
In this paper, a visible light induced photodegradation study has been carried out on Reactive Blue 4 dye (RB 4) with undoped, N-doped and N,Fe codoped TiO2. The best activity was found for N,Fe codoped TiO2 which was further modified to improve the separation process of the photocatalyst as magnetically separable γ-Fe2O3/N,Fe codoped TiO2.25,26 The enhanced photoactivity of γ-Fe2O3/N,Fe codoped TiO2 has been accounted for based on the suggested mechanism and its physico-chemical properties.
2. Experimental
2.1. Reagents and chemicals
Tetrabutyl titanate (TBT), Fe(NO3)3·9H2O, FeSO4, urea and RB 4 dye (M.W. = 637.43 g mol−1, C.I. 61
205) were purchased from Sigma-Aldrich, USA. All other chemicals, ammonium hydroxide (NH3·H2O, 25–28%), acetic acid, and absolute ethanol were of analytical grade and used as received without any further purification. Double distilled water (DDW) was used throughout the study.
2.2. Preparation of the modified photocatalysts
2.2.1. Synthesis of γ-Fe2O3. A mixture of 0.18 M Fe(NO3)3·9H2O (50 ml) and 0.12 M FeSO4 (50 ml) solutions were mechanically stirred at 40 °C, followed by the addition of NH3·H2O (5 ml). The mixture was stirred for 15 min and heated at 60 °C for 10 min. The black precipitate formed was separated with a magnet and washed with double distilled water (4 × 20 ml). The as-obtained precipitate of iron oxide was finally heated at 100 °C to form stable γ-Fe2O3 as a dry powder.
2.2.2. Preparation of undoped, N-doped and N,Fe codoped TiO2. Firstly, TBT (5 ml) and acetic acid (5 ml) were added to 25 ml of absolute ethanol (solution A). Secondly, acetic acid (2.5 ml) and distilled water (3 ml) were added to 12.5 ml of absolute ethanol (solution B). Solution B was added drop-wise into solution A with vigorous magnetic agitation. The obtained mixture was stirred for 3 h, followed by drying at 100 °C in an oven, it was then porphyrized into a powder and annealed at 500 °C at a rate of 3 °C per minute in a programmable furnace for 3 h. The final powder obtained was undoped TiO2. N-doped and N,Fe codoped TiO2 were prepared with the same procedure by using dopant sources, urea (1.8 g, 30 mmol) and ferric nitrate (0.17 g, 0.43 mmol) for nitrogen and iron respectively, added into solution B.
2.2.3. Preparation of γ-Fe2O3/N,Fe codoped TiO2. γ-Fe2O3 (0.77 g, 4.82 mmol) was added into solution A from the above procedure to obtain the γ-Fe2O3/N,Fe codoped TiO2 catalyst. The schematic process for the synthesis of the modified TiO2 has been shown in Fig. 1.
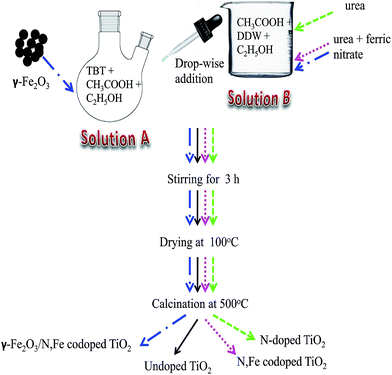 |
| Fig. 1 Schematic process for the synthesis of the modified TiO2. | |
The as-synthesized materials were characterized using X-ray diffraction (XRD), transmission electron microscopy (TEM), Brunauer–Emmett–Teller (BET) measurements, Fourier transform infra-red (FTIR) spectroscopy, UV-vis diffuse reflectance spectroscopy (UV-vis DRS), X-ray photoelectron spectroscopy (XPS) and energy dispersive X-ray spectroscopy (EDS) techniques.
2.3. Characterization of nanocrystalline TiO2
XRD measurements were performed using an X-ray powder diffractometer (XPERT-PRO) operated at 45 kV and 40 mA with Cu-Kα radiation (λ = 0.15406 nm) at a scan angle (2θ) of 20–100°. TEM images of the samples were studied using a transmission electron microscope from Hitachi (H- 7500) with an accelerating voltage of 120 kV. EDS was performed with an EDX-INCAx-act, Oxford instrument, with TEM capability attached. The hydrodynamic particle sizes were determined using a Malvern Zetasizer ZS90. BET surface area measurements were performed using a Quantachrome Nova Win version 10.01 and the samples were previously outgassed at 180 °C for 2 h. FTIR spectra in the range 4000–400 cm−1 were obtained using a Perkin Elmer-Spectrum RX-I. UV-vis DRS of the samples was performed on a Perkin Elmer-Lambda 35 UV-vis spectrophotometer, with a double-beam optical arrangement, equipped with an integrating sphere attachment. BaSO4 was used as a reference.27 XPS was recorded on a Shimadzu ESCA-3200 spectrometer. The shift in binding energy due to relative surface charging was corrected using the C 1s level at 284.6 eV as an internal standard.
2.4. Photocatalytic experiments
The photocatalytic experiments were performed with 200 ml of dye solution in an immersion well type reactor. The experiments were conducted using a 100 W halogen lamp with the circulation of 2 M NaNO2 solution for the absorption of any irradiation below 400 nm and with vigorous bubbling of oxygen into the solution to increase the dissolved oxygen concentration. An aliquot of 5 ml was taken from the reactor at regular intervals of time using a syringe. The suspension was centrifuged for 5 min at 4000 rpm and subsequently filtered through a Millipore filter (pore size 0.45 μm) to remove the catalyst and the filtrate was analyzed using a UV-vis spectrophotometer.
3. Characterization
Fig. 2 shows the XRD pattern of all the synthesized photocatalysts. The XRD patterns shown in Fig. 2a–ccorrespond to undoped, N-doped and N,Fe codoped TiO2 respectively, indicating the presence of an anatase phase (JCPDS no. of pure anatase 01-071-1167) for only the undoped and N-doped TiO2, while in the case of N,Fe codoped TiO2, an anatase phase with a trace amount of rutile phase was observed (JCPDS no. of pure rutile 01-078-1510), i.e. 95.55% anatase and 4.45% rutile phase,28 as calculated by the following equation:
where IA is the intensity of the 101 peak of anatase and IR is the intensity of the 110 peak of rutile.
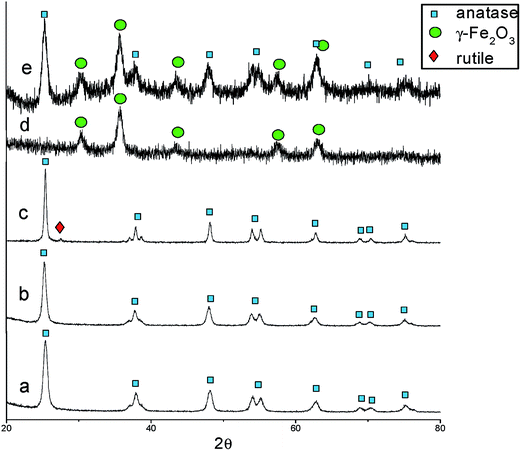 |
| Fig. 2 The XRD patterns of the synthesized photocatalysts calcined at 500 °C: (a) undoped TiO2, (b) N-doped TiO2, (c) N,Fe codoped TiO2, (d) γ–Fe2O3 and (e) γ-Fe2O3/N,Fe codoped TiO2. | |
The XRD diffraction peaks for γ-Fe2O3 in Fig. 2d at 2θ = 30.29°, 35.72°, 43.40°, 57.45°, 63.07° and 74.6° confirm its crystal lattice structure.21 The diffraction peaks in Fig. 2e for γ-Fe2O3/N,Fe codoped TiO2 show the anatase peaks of TiO2 and the peaks of γ-Fe2O3 which remain unchanged, and reveal that the magnetic properties are not lost after high temperature treatment. The absence of a rutile phase in Fig. 2e when compared to Fig. 2c suggests that there is some interaction between γ-Fe2O3 and N,Fe codoped TiO2.
The crystallite sizes of all the photocatalysts were calculated using the Debye–Scherrer equation as shown in Table 1.
Table 1 Physico-chemical properties of the synthesized photocatalysts
|
Undoped TiO2 |
N-doped TiO2 |
N,Fe codoped TiO2 |
γ-Fe2O3/N,Fe codoped TiO2 |
Crystallite size (nm) from XRD |
14.2 |
13.6 |
21.3 |
12.0 |
Range of particle size (nm) from TEM |
8–15 |
12–18 |
9–12 |
7–14 |
Hydrodynamic size (Å) |
238.8 |
132.8 |
25.2 |
42.0 |
Band gap (eV) |
3.0 |
2.6 |
2.4 |
1.5 |
BET surface area (m2 g−1) |
103.8 |
83.4 |
98.0 |
131.5 |
Fig. 3 shows the TEM images of all the synthesized samples. The particle size from TEM was found to be in agreement with the crystallite size calculated from XRD analysis. The undoped and N,Fe codoped TiO2 (Fig. 3a and c) have a cubic shape, while the N-doped TiO2 (Fig. 3b) showed a rectangular shape with low aggregation of particles. Fig. 3d of γ-Fe2O3/N,Fe codoped TiO2 shows the heterogeneous dispersion of ultrafine γ-Fe2O3 magnetic nanoparticles in the N,Fe codoped TiO2 matrix.29 The heterojunction formed between γ-Fe2O3 and N,Fe codoped TiO2 has been shown in the enlarged view of Fig. 3d. The dark region corresponds to γ-Fe2O3 and the light region corresponds to N,Fe codoped TiO2. The presence of a well defined SAED ring pattern in the HRTEM image of γ-Fe2O3/N,Fe codoped TiO2 confirmed the crystallinity of titania. Furthermore, the calculated d-spacings of 0.35 nm and 0.25 nm, corresponding to the (101) plane of anatase TiO2 and the (110) plane of Fe2O3 respectively, confirm the presence of a heterojunction, as shown in Fig. 3e.
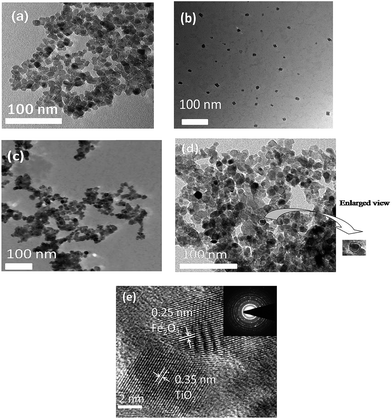 |
| Fig. 3 TEM images of: (a) undoped TiO2, (b) N-doped TiO2, (c) N,Fe codoped TiO2, (d) γ-Fe2O3/N,Fe codoped TiO2 and (e) the HRTEM of γ-Fe2O3/N,Fe codoped TiO2. | |
The hydrodynamic particle size of all the synthesized TiO2 samples has also been determined, as given in Table 1. The hydrodynamic sizes of the photocatalysts were found to be larger than the crystallite sizes, calculated using the Debye–Scherrer equation, due to the aggregation of the powders in aqueous media.
The BET surface areas of the synthesized samples are shown in Table 1. Among all the samples, γ-Fe2O3/N,Fe codoped TiO2 has the highest surface area which can be accounted for based on the different nucleation and growth of the nanocrystallites, resulting in the formation of particles with smaller sizes and correspondingly larger surface areas.30
The FTIR spectra have been analyzed for all of the samples to confirm the composition and structure of the nanocomposites (Fig. 4). In all the spectra, the peaks at 1620 and 3400 cm−1 are attributed to absorption by water and hydroxyl groups31 and the peaks at 500–900 cm−1 originate from the Ti–O–Ti bond.32 The small peaks at around 1450, 1230 and 1090 cm−1 can be assigned to the nitrogen atoms embedded in the TiO2 network in the doped TiO2 samples. In the spectrum of γ-Fe2O3/N,Fe codoped TiO2, a peak at around 570 cm−1 is observed which is due to the vibration of the Fe–O bond.
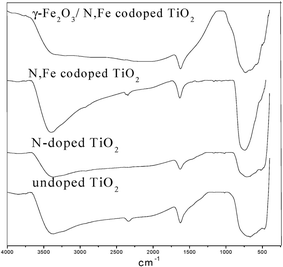 |
| Fig. 4 The FTIR spectra of the synthesized photocatalysts. | |
Fig. 5 shows the UV-vis DRS spectra of all the synthesized catalysts and their band gaps were calculated using Tauc plots.33 The undoped TiO2 has a band gap of 3.0 eV indicating that it can absorb only UV light. In the N-doped TiO2, the band gap shifts from 3.0 eV to 2.6 eV because of the hybridization of the N 2p orbitals with the O 2p orbitals. In N,Fe codoped TiO2, the incorporation of iron further decreases the band gap to 2.4 eV.34 The doping of nitrogen and iron into TiO2 forms an impurity band just above the valence band (VB) and below the conduction band (CB) respectively in TiO2.35 The reduction in the band gap confirms that both nitrogen and iron weave efficiently into the crystal lattice structure. In γ-Fe2O3/N,Fe codoped TiO2, the band gap further lowers to 1.5 eV which justifies its increased absorption in the visible region.36 The shift in the band gap might be due to the formation of a heterojunction between γ-Fe2O3 and N,Fe codoped TiO2, which was further confirmed by the HRTEM analysis. This is further supported by literature reports where heterojunction formation leads to a reduction in the band gap.37–39
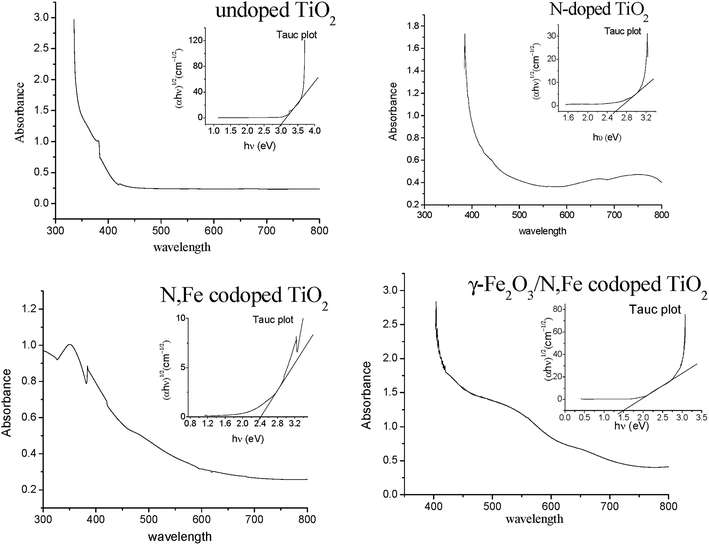 |
| Fig. 5 UV-vis DRS analysis of the synthesized photocatalysts. | |
The XPS spectra of the doped samples, as represented in Fig. 6, show a clear N 1s binding energy around 400 eV which has been assigned to the interstitial nitrogen and residual non-influent N species at the surface.40–42 The N,Fe codoped TiO2 sample shows peaks for the binding energies of Fe 2p located at 722.6 and 709.7 eV indicating the existence of Fe–O bonds on the surface of the sample.
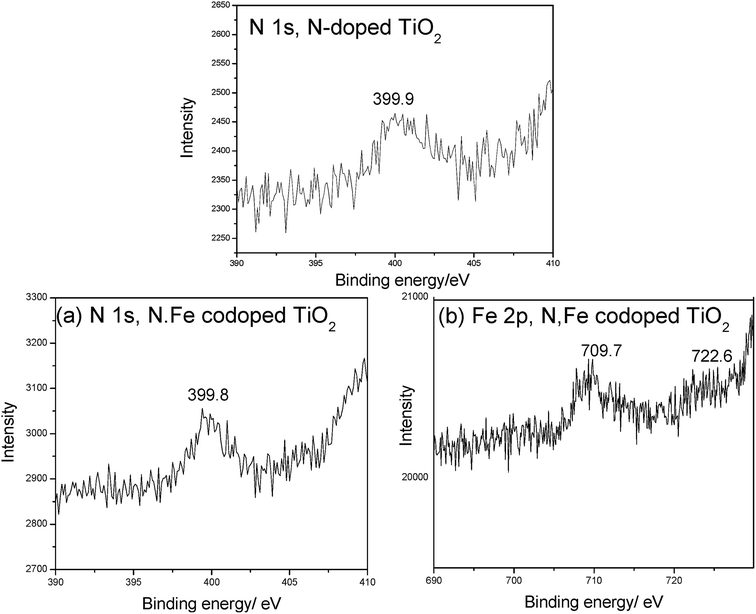 |
| Fig. 6 XPS spectra of N-doped TiO2 and N,Fe codoped TiO2. | |
EDS analysis has been used for the elemental analysis of γ-Fe2O3/N,Fe codoped TiO2 and the energy peaks corresponding to the elements N, Fe, O and Ti were observed as shown in Fig. 7.43
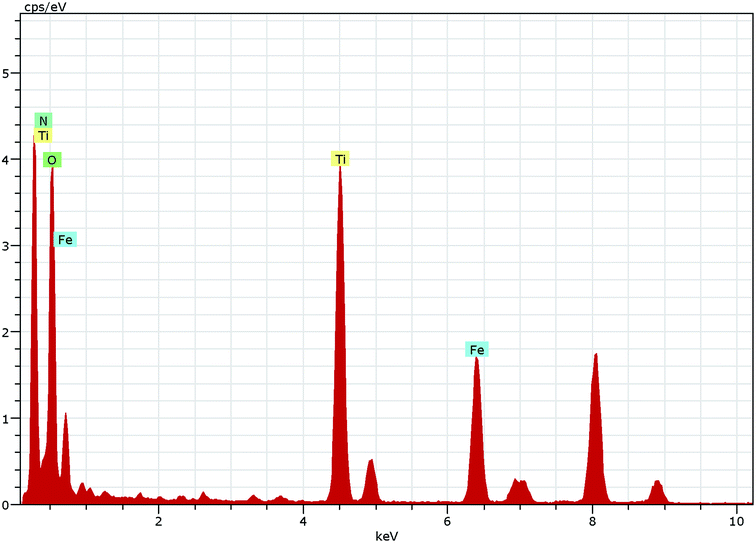 |
| Fig. 7 The EDS data for γ-Fe2O3/N,Fe codoped TiO2. | |
4. Results and discussion
4.1. Results of the photoactivity test
RB 4, a textile azo dye, was chosen as the target compound to test the photocatalytic activity of the prepared photocatalysts. The degradation efficiency of the synthesized catalysts was defined as C/Co, where C and Co stand for the remnant and initial concentrations of the dye respectively. The order of degradation efficiency with respect to the initial rate of reaction for the photocatalytic degradation of the dye under visible light was found to be γ-Fe2O3/N,Fe codoped TiO2 > N,Fe codoped TiO2 > N-doped TiO2 > undoped TiO2, as shown in Fig. 8.
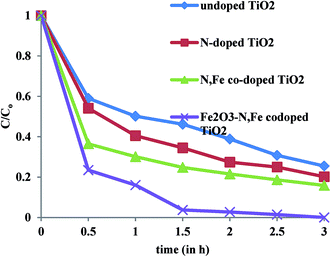 |
| Fig. 8 Photocatalytic degradation of RB 4 dye using the synthesized catalysts; Co = 10 ppm, catalyst amount = 0.24 g/200 ml. | |
4.2. Discussion on the mechanism of degradation
In γ-Fe2O3/undoped TiO2, the CB and VB of γ-Fe2O3 are located between the CB and VB of TiO2 as shown in Fig. 9.44 The electron gets excited from the VB to the CB of γ-Fe2O3 by the absorption of visible light, but neither the electrons in the CB nor the holes in the VB of γ-Fe2O3 can be transferred to the CB or VB of TiO2 by following pathways A and B, due to unfavorable energy band matching.
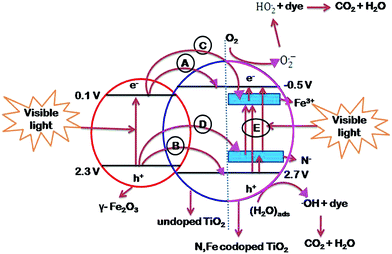 |
| Fig. 9 Schematic representation of mechanism involved for degradation of dye in γ-Fe2O3/N,Fe codoped TiO2 heterojunction. | |
In γ-Fe2O3/N,Fe codoped TiO2, this mismatch of energy bands does not limit the photoactivity of catalyst because codoping with N and Fe induces the formation of new states close to the VB and CB respectively. Due to these newly formed states, the electron and hole mobilities from γ-Fe2O3 to the impurity band of N,Fe codoped TiO2 become feasible by pathways C and D respectively as shown in Fig. 9.45–50 The probable reactions of the electrons and holes by pathways C and D, which lead to the degradation of RB 4 dye, are as below.
Pathway C.
HO2˙ + dye → → → CO2 + H2O |
Pathway D.
˙OH + dye → → → CO2 + H2O |
The narrow band gap of γ-Fe2O3/N,Fe codoped TiO2 (1.5 eV) participates in increasing the quantity of photoinduced electrons and holes via pathway E, as shown in Fig. 7, which leads to an enhanced, more efficient utilization of visible light for the degradation of dye.51 The high photoactivity of γ-Fe2O3/N,Fe codoped TiO2 is further facilitated by the large surface area and pure anatase phase because the fast migration of photogenerated electrons and holes from the bulk to the surface occurs more easily in the anatase phase, thus resulting in a low recombination rate of the photogenerated charge carriers, as reported in the literature.52–54
4.3. Photocatalyst reuse
The magnetic γ-Fe2O3 nanoparticles in TiO2 facilitate the separation of the photocatalyst, which improves the recyclability of the catalyst. The recycling runs were carried out by separating the γ-Fe2O3/N,Fe codoped TiO2 catalyst under an external magnetic field of intensity 365 gauss. A mass recovery of ∼98% was achieved with a minor loss. This loss was expected owing to the small size. As shown in Fig. 10, the photocatalytic activity of catalyst remains almost constant up to 4 runs.
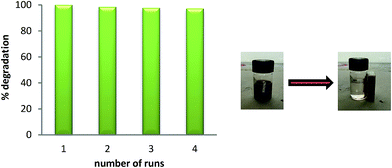 |
| Fig. 10 Recycling runs for the degradation of RB 4 dye by γ-Fe2O3/N,Fe codoped TiO2; the inset is a photograph showing the separation of the catalyst under a magnetic field. | |
5. Conclusion
In this paper, we have successfully synthesized γ-Fe2O3/N,Fe codoped TiO2 which resulted in the formation of a heterojunction and was well characterized. The synthesized γ-Fe2O3/N,Fe codoped TiO2 exhibits enhanced photocatalytic activity in comparison to the non-magnetic N,Fe codoped TiO2 and has been well accounted for based on the coupling effect between γ-Fe2O3 and N,Fe codoped TiO2, shift in the band gap, low electron–hole recombination rate, large surface area and presence of the anatase phase. Moreover, the fast magnetic separation and efficient photoactivity of the catalyst are advantageous for using the synthesized catalyst in industries to address environmental issues.
Acknowledgements
The generous financial support from the PEC University of Technology, Chandigarh and TEQIP (World Bank Project) is gratefully acknowledged. We also thank RSIC, Chandigarh, Dr Prakash and Dr R.V. Jasra from Reliance Industries, Gujarat for analysis.
References
- Y. Zhang, J. C. Crittenden, D. W. Hand and D. L. Perram, Environ. Sci. Technol., 1991, 28, 435–442 CrossRef PubMed.
- M. R. Hoffmann, S. T. Martin, W. Choi and D. W. Bahnemann, Chem. Rev., 1995, 95, 69–96 CrossRef CAS.
- J. Zhao, T. Wu, K. Wu, K. Oikawa, H. Hidaka and N. Serpone, Environ. Sci. Technol., 1998, 32, 2394–2400 CrossRef CAS.
- H. Li, X. He, Z. Kang, H. Huang, Y. Liu, J. Liu, S. Lian, C. H. A. Tsang, X. Yang and S. T. Lee, Angew. Chem., Int. Ed., 2010, 49, 4430–4434 CrossRef CAS PubMed.
- X. Chen and S. S. Mao, Chem. Rev., 2007, 107, 2891–2959 CrossRef CAS PubMed.
- W. Q. Fang, Z. Huo, P. Liu, X. L. Wang, M. Zhang, Y. Jia, H. Zhang, H. Zhao, H. G. Yang and X. Yao, Chem.–Eur. J., 2014, 20, 11439–11444 CrossRef CAS PubMed.
- D. Qi, M. Xing and J. Zhang, J. Phys. Chem. C, 2014, 118, 7329–7336 CAS.
- S. Sridhar, A. Viswanathan, K. Venkateswarlu, N. Rameshbabu and N. L. Parthasarathi, J. Porous Mater., 2015, 22, 545–557 CrossRef CAS.
- Y. Wang, R. Zhang, J. Li, L. Li and S. Lin, Nanoscale Res. Lett., 2014, 9, 46–54 CrossRef PubMed.
- J. M. Valero, S. Obregon and G. Colon, ACS Catal., 2014, 4, 3320–3329 CrossRef CAS.
- N. Khakpash, A. Simchi and T. Jafari, J. Mater. Sci.: Mater. Electron., 2012, 23, 659–667 CrossRef CAS.
- L. C. Jia, C. C. Wu, S. Han, N. Yao, Y. Y. Li, Z. B. Li, B. Chi, J. Pu and L. Jian, J. Alloys Compd., 2011, 509, 6067–6071 CrossRef CAS PubMed.
- A. T. Kuvarega, R. W. M. Krause and B. B. Mamba, J. Phys. Chem. C, 2011, 115, 22110–22120 CAS.
- X. Li, Z. M. Chen, Y. C. Shi and Y. Y. Liu, Powder Technol., 2011, 207, 165–169 CrossRef CAS PubMed.
- K. S. Rane, R. Mhalsiker, S. Yin, T. Sato, K. Cho, E. Dunbar and P. Biswas, J. Solid State Chem., 2006, 179, 3033–3044 CrossRef CAS PubMed.
- H. M. Chen, C. H. Deng and X. M. Zhang, Angew. Chem., Int. Ed., 2010, 49, 607–611 CrossRef CAS PubMed.
- P. M. Rao and X. L. Zheng, Nano Lett., 2011, 11, 2390–2395 CrossRef CAS PubMed.
- V. Tyrpeki, J. P. Vejpravova and A. G. Roca, Appl. Surf. Sci., 2011, 257, 4844–4848 CrossRef PubMed.
- M. S. Lucas, P. B. Tavares, J. A. Peres, J. L. Faria, M. Rocha, C. Pereira and C. Freire, Catal. Today, 2013, 209, 116–121 CrossRef CAS PubMed.
- R. Chalasani and S. Vasudevan, ACS Nano, 2013, 7, 4093–4104 CrossRef CAS PubMed.
- B. Cui, H. Peng, H. Xia, X. Guo and H. Guo, Sep. Purif. Technol., 2013, 103, 251–257 CrossRef CAS PubMed.
- M. He, D. Li, D. Jiang and M. Chen, J. Solid State Chem., 2012, 192, 139–143 CrossRef CAS PubMed.
- M. Pelaez, B. Baruwati, R. S. Varma, R. Luque and D. D. Dionysiou, Chem. Commun., 2013, 49, 10118–10120 RSC.
- L. Yu, X. Yang, J. He, Y. He and D. Wang, Sep. Purif. Technol., 2015, 144, 107–113 CrossRef CAS PubMed.
- J. R. Jeong, S. J. Lee, J. D. Kim and S. C. Shin, Phys. Status Solidi B, 2004, 241, 1593–1596 CrossRef CAS PubMed.
- S. H. Xuan, Y. J. Wang, J. C. Yu and K. C. Leung, Chem. Mater., 2009, 21, 5079–5087 CrossRef CAS.
- E. M. Patterson, C. E. Shelden and B. H. Stockton, Appl. Opt., 1977, 13, 729–732 CrossRef PubMed.
- Y. Su, Y. Xiao, Y. Li, Y. Du and Y. Zhang, Mater. Chem. Phys., 2011, 126, 761–768 CrossRef CAS PubMed.
- M. Niyaifar, A. Hasanpour, H. Mohammadpour and J. Anighian, Phys. Status Solidi A, 2013, 210, 1190–1194 CrossRef CAS PubMed.
- K. M. Parida and B. Naik, J. Colloid Interface Sci., 2009, 333, 269–276 CrossRef CAS PubMed.
- X. X. Yu, S. W. Liu and J. G. Yu, Appl. Catal., B, 2011, 104, 12–20 CrossRef CAS PubMed.
- A. Zielinska, E. Kowalska and J. W. Sobczak, Sep. Purif. Technol., 2010, 45, 155–162 Search PubMed.
- A. Ibrahim and S. K. J. A. Ani, Czech J. Phys., 1994, 44, 785–797 CrossRef CAS.
- Y. Cong, J. Zhang, F. Chen, M. Anpo and D. He, J. Phys. Chem. C, 2007, 111, 10618–10623 CAS.
- R. Asahi, T. Morikawa, T. Ohwaki, K. Aoki and Y. Taga, Science, 2001, 293, 269–271 CrossRef CAS PubMed.
- H. Liu, H. K. Shon, X. Sun, S. Vigneswaran and H. Nan, Appl. Surf. Sci., 2011, 257, 5813–5819 CrossRef CAS PubMed.
- R. Khan and T. J. Kim, J. Hazard. Mater., 2009, 163, 1179–1184 CrossRef CAS PubMed.
- J. Yang, X. Wang, J. Dai and J. Li, Ind. Eng. Chem. Res., 2014, 53, 12575–12586 CrossRef CAS.
- A. Banisharif, A. A. Khodadadi, Y. Mortazavi, A. A. Firooz, J. Beheshtian, S. Agah and S. Menbari, Appl. Catal., B, 2015, 165, 209–221 CrossRef CAS PubMed.
- S. Livraghi, M. R. Chierotti, E. Giamello, G. Magnacca, M. C. Paganini, G. Cappelletti and C. L. Bianchi, J. Phys. Chem. C, 2008, 112, 17244–17252 CAS.
- F. Napoli, M. Chiesa, S. Livraghi, E. Giamello, S. Agnoli, G. Granozzi, G. Pacchioni and C. Di Valentin, Chem. Phys. Lett., 2009, 477, 135–138 CrossRef CAS PubMed.
- S. Sato, R. Nakamura and S. Abe, Appl. Catal., A, 2005, 284, 131–137 CrossRef CAS PubMed.
- M. Montazer, A. Behzadnia and E. Pakdel, J. Photochem. Photobiol., B, 2011, 103, 637–640 CrossRef PubMed.
- S. B. Rawal, S. Bera, D. Lee, D. J. Jang and W. I. Lee, Catal. Sci. Technol., 2013, 3, 1822–1830 CAS.
- Y. Bessekhouad, N. Chaoui, M. Trzpit, N. Ghazzal, D. Robert and J. V. Weber, J. Photochem. Photobiol., A, 2006, 183, 218–224 CrossRef CAS PubMed.
- U. I. Gaya and A. H. Abdullah, J. Photochem. Photobiol., C, 2008, 9, 1–12 CrossRef CAS PubMed.
- S. B. Rawal, A. K. Chakraborty, Y. J. Kim, H. J. Kim and W. I. Lee, RSC Adv., 2012, 2, 622–630 RSC.
- Y. J. Kim, B. Gao, S. Y. Han, M. H. Jung, A. K. Chakraborty, T. Ko, C. Lee and W. I. Lee, J. Phys. Chem. C, 2009, 113, 19179–19184 CAS.
- S. Shamaila, A. K. L. Sajjad, F. Chen and J. Zhang, J. Colloid Interface Sci., 2011, 356, 465–472 CrossRef CAS PubMed.
- H. Gnaser, M. R. Savina, W. F. Chalaway, C. E. Tripa, I. V. Veryovkin and M. J. Pellin, Int. J. Mass Spectrom., 2005, 245, 61–67 CrossRef CAS PubMed.
- Y. Cong, J. Zhang, F. Chen, M. Anpo and D. He, J. Phys. Chem. C, 2007, 111, 10618–10623 CAS.
- J. Joo, J. Shim, H. Seo, N. Jung, U. Weisner, J. Lee and S. Jeon, Anal. Chem., 2010, 82, 3032–3037 CrossRef CAS PubMed.
- J. Joo, Y. Ye, D. Kim, J. Lee and S. Jeon, Mater. Lett., 2013, 93, 141–144 CrossRef CAS PubMed.
- J. Zhang, P. Zhou, J. Liu and J. Yu, Phys. Chem. Chem. Phys., 2014, 16, 20382–20386 RSC.
|
This journal is © The Royal Society of Chemistry 2015 |
Click here to see how this site uses Cookies. View our privacy policy here.