DOI:
10.1039/C5RA07652H
(Paper)
RSC Adv., 2015,
5, 51318-51329
Numerical investigation of the interaction between homogeneous and heterogeneous reactions of fuel-lean hydrogen–air mixtures over platinum in planar micro-channels
Received
27th April 2015
, Accepted 4th June 2015
First published on 4th June 2015
Abstract
The interaction between homogeneous and heterogeneous reactions of a fuel-lean hydrogen–air mixture over platinum in a catalytic planar micro-channel was investigated numerically to assess the relative importance and respective contributions of homogeneous and heterogeneous reactions for different channel gap distances, inlet mass fluxes and equivalence ratios. Simulations were carried out with a two-dimensional computation that included detailed chemical kinetic mechanisms for the hetero-/homogeneous reaction. The results indicated that channel size can significantly affect the homogeneous reaction because of the effective radical and heat losses to the wall, despite the fact that the heterogeneous reaction remains basically unchanged. The catalytic wall helps in sustaining the homogeneous reaction in smaller channels by decreasing heat losses to the wall as a result of the exothermic heterogeneous reaction, while it also inhibits the homogeneous reaction because of wall radical quenching caused by typically higher absorption of OH radicals on the wall. Product species contribute even further to inhibiting homogeneous reaction because of the stronger diffusive mass flux in the smaller channel. In the present work, the main parameter effects on the interaction between homogeneous and heterogeneous reactions are explored. The limiting values of these parameters beyond which the homogeneous reaction becomes negligible as compared with the heterogeneous reaction are identified.
1. Introduction
In recent years, micro-combustion has attracted increased attention due to the growing interest in developing micro-power generation systems.1 Although pure homogeneous combustion can be stabilized both in micro-scale2 and meso-scale3 channels, which are defined respectively as channels smaller or larger than 1.0 mm, increasing the surface-to-volume ratio renders heterogeneous combustion the preferred route compared with pure homogeneous combustion.4 Catalytic reaction broadens the classical flammability limits and leads to stable combustion even in the presence of high heat losses.5 Pizza et al.6,7 have further demonstrated the potential of a catalytic wall in inhibiting intrinsic flame instability in micro-scale and meso-scale channels.
Hydrogen–air flames are easily quenched at the micro-scale because of two primary mechanisms, i.e. thermal and radical quenching.8 Thermal quenching occurs when sufficient heat is removed through the wall, accordingly combustion cannot be self-sustained. Radical quenching occurs via radical adsorption on the wall and subsequent recombination and desorption, which results in lack of homogeneous reaction. The micro-scale enhances the heat transfer from the homogeneous flame to the wall and increases the radical mass transfer.9 Therefore, mass and heat transfer on the wall play a crucial role in defining the combustion characteristics as the characteristic size decreases, especially in catalytic micro-combustors. Chen et al.10 proposed that catalytic reaction generally have lower activation energy than homogeneous reaction, and catalytic wall can enhance reaction and reduce heat loss through the wall and radical quenching on the wall.
There are two effects of heterogeneous reaction on homogeneous reaction in catalytic micro-channels. On one hand, heterogeneous reaction will consume part of the fuel in upstream zone, and the reduced fuel and the increased product will weaken the homogeneous reaction.10 Wang et al.11 have verified that in alumina ceramic micro-combustors with catalytic Pt layer. On the other hand, heterogeneous reaction causes high temperature and radical quenching on the catalytic wall, helping to enhance homogeneous reaction and overcome heat losses from the homogeneous flame to the wall. Therefore, the homogeneous flame can be sustained in catalytic micro-channels smaller than the flame quench distance. These two competing effects determine whether homogeneous flame can occur in catalytic conditions.
Several numerical simulations as well as experimental studies have been performed to explore the role of catalytic wall and wall radical quenching on homogeneous reaction. Goralski and Schmidt12 performed a two-dimensional plug-flow reactor model with detailed homogeneous and heterogeneous reaction mechanisms of methane–air mixtures in high-temperature and short-contact-time catalytic combustor to gain insight into the interaction between homogeneous and heterogeneous reactions. They found that the homogeneous reaction in all cases (variety of temperatures, pressures, compositions, and catalyst pore sizes) is significantly inhibited by the heterogeneous reaction; this inhibition is a result of the adsorption of radical species from the gas phase to the catalyst surface, which prevents the initiation of free-radical chain reactions in the gas phase. Aghalayam et al.13 studied the role of wall radical quenching in ignition, extinction and auto-thermal behaviors of premixed hydrogen–air flame impinging on a flat wall surface. They proposed that the radical quenching on the wall inhibits the homogeneous reaction especially at ignition due solely to the surface reaction kinetics, while the heat release due to surface catalytic reaction can reduce this chemical effect. Ghermay et al.14 experimentally and numerically investigated the effects of hydrogen preconversion on the homogeneous ignition characteristics of H2/O2/N2/CO2 mixtures over platinum at moderate pressures. They found that homogeneous ignition was largely inhibited because of the combined effects of intrinsic homogeneous reaction kinetics and the competition between the homogeneous and heterogeneous reaction pathways for fuel consumption. Moreover, the catalytically-produced water inhibits homogeneous reaction and this kinetic inhibition is exacerbated by the diffusional imbalance of hydrogen. Finally, radical adsorption/desorption reactions hinder the onset of homogeneous ignition and this effect is more pronounced at atmospheric pressure; opposite this, over the post-ignition reactor length, radical adsorption/desorption reactions significantly inhibit homogeneous reaction at higher pressure while their impact is weaker at atmospheric pressure. Karagiannidis et al.15 studied the hetero-/homogeneous reaction and the stability limits of methane–air in catalytic micro-reactors (Pt-coated) using detail homogeneous and heterogeneous chemical reaction mechanisms. The result shown that the presence of catalytic wall can expands the stability limits of homogeneous flame by the heat release due to heterogeneous reaction. Chen et al.10 performed an axisymmetric CFD (computational fluid dynamics) model using detailed heterogeneous and homogeneous kinetics schemes to investigate the effect of catalytic wall on homogeneous reaction inside a catalytic micro-tube. This work shows the homogeneous flame and heterogeneous reaction are governed at the downstream and upstream zone, respectively; the zone dominated by heterogeneous reaction expands to downstream with increasing the inlet flow velocity, and finally occupied the whole channel; homogeneous reaction is inhibited by heterogeneous reaction along with it is ignited and sustained by the heat from heterogeneous reaction. They also proposed that the effect of heterogeneous reaction on homogeneous reaction can be divided into three main types: in first type, homogeneous flame is weakened by heterogeneous reaction but it can be sustained in a large range of inlet flow velocity; in second type, homogeneous reaction only have a significant effect on the amounts of intermediate species; in third type, homogeneous reaction can be wholly neglected as compared with heterogeneous reaction; increasing inlet flow velocity, decreasing fuel concentration or channel size will make their interaction shift from the first type to the third type. Fanaee and Esfahani16 analyzed the effect of main parameters such as maximum temperature, flame quenching distance, and reaction zone thickness on homogeneous flame in micro-combustors in catalytic (Pt-coated) and non-catalytic conditions, the results showed that the effect of catalytic wall on expanding homogeneous flame limits in a lean mixture is larger than the rich case. Appel et al.17 experimentally and numerically investigated the homogeneous ignition of hydrogen–air mixtures over platinum, and the results shown that the understanding of homogeneous and heterogeneous kinetics is crucial to the development of such catalytically stabilized combustion technology. Choi et al.18 experimentally investigated the effect of catalytic wall properties on combustion characteristics of hydrogen–air mixtures over supported platinum catalyst in a sub-millimeter catalytic burner, and proposed that catalyst concentration on the solid wall has no effect on the total homogeneous and heterogeneous reactions and this micro-combustor performance.
Homogeneous reaction is often neglected in catalytic combustion model even though its effect, especially at elevated pressure and on steady-state reactor operation, can be significant.19 As discussed in previous studies, these works mainly focus on the effect of heterogeneous reaction or catalytic wall on homogeneous reaction. However, the interaction between homogeneous and heterogeneous reactions, especially the relative importance or respective contribution of homogeneous reaction to total hydrogen conversion as compared with heterogeneous reaction, is still poorly understood. In this work, the relative importance and respective contribution between homogeneous and heterogeneous reactions associated with fuel-lean hydrogen–air combustion over platinum under different operating conditions (channel gap distance, inlet mass flux, and equivalence ratio) are assessed in planar micro-channels. Detailed hetero-/homogeneous reaction mechanisms are employed to fully consider effect of the minor species in determining the hetero-/homogeneous reaction pathways.
2. Numerical models and simulation approach
2.1. Model geometry
Fig. 1 shows a schematic view of planar micro-channel (2D parallel plates) in which steady-state combustion of fuel-lean hydrogen–air mixture takes place. FLUENT20 coupled with CHEMKIN21 was used to simulate the fluid flow in the catalytic planar channel of gap distance D = 0.8 mm, length L = 8.0 mm, and wall thickness δ = 0.1 mm. The catalytic micro-combustor consisted of two horizontal non-porous refractory ceramic plates made of SiC. Inner horizontal surfaces contained platinum catalyst washcoat, and its properties are shown in Table 1. The incoming hydrogen–air mixture was fully premixed with an equivalence ratio of φ = 0.4. The fuel–air equivalence ratio φ of a system is defined as the ratio of the fuel-to-oxidizer ratio to the stoichiometric fuel-to-oxidizer ratio. In addition, x depicts the downstream distance and y represents the radial distance, respectively. Based on this physical model, a 2-D axial symmetric computational domain is established.
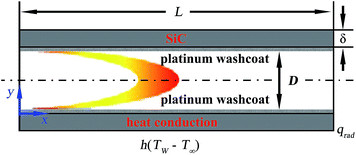 |
| Fig. 1 Schematic diagram of the planar micro-channel geometry. | |
Table 1 The properties of platinum catalyst washcoat
Property |
Value |
Reference |
Catalyst surface site density Γ (mol cm−2) |
2.7 × 10−9 |
22 |
Catalyst particle diameter dp (m) |
1.0 × 10−4 |
23 and 24 |
Permeability of the catalyst washcoat (m2) |
1.0 × 10−8 |
25 and 26 |
Average pore diameter dpore (m) |
2.61 × 10−8 |
23 and 27 |
Catalyst porosity εcat |
0.4 |
25 and 28 |
Catalyst tortuosity τcat |
8.1 |
23 and 29 |
Thickness δs (μm) |
2.0 |
17 and 26 |
2.2. Mathematical model
Recently, transient two-dimensional simulations of fuel-lean hydrogen–air combustion were reported by Brambilla et al.2 in a 2 mm-height planar channel coated with platinum, using detailed hetero-/homogeneous chemistry and transport along with heat conduction in the solid wall. Subsequently, fundamental hetero-/homogeneous combustion studies of fast processes such as light-off, homogeneous ignition and hetero-/homogeneous combustion interactions during dynamic operation, with time scales ranging from fractions of a millisecond to a few milliseconds, have also been carried out. They have given the model's capacity for the first time to resolve all relevant time scales. In the present work, main objective is to examine the interaction between homogeneous and heterogeneous reactions in the steady-state premixed combustion process, while the above fast processes have not been taken into consideration. Therefore, simulations were performed with a two-dimensional steady-state model. The governing equations for steady, laminar, reactive gas flow with heterogeneous reaction are as follows:30–34
Continuity equation:
|
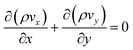 | (1) |
Momentum equation:
|
 | (2) |
|
 | (3) |
Energy equation:
|
 | (4) |
Species equation:
|
 | (5) |
where
ρ (kg m
−3) is density;
vx (m s
−1) and
vy (m s
−1) are the velocity components in the
x and
y directions, respectively;
p (Pa) is pressure;
τ (Pa) is shear stress;
hi (J kg
−1) is the enthalpy of species
i;
kf (W m
−1 K
−1) is the thermal conductivity of fluid;
T (K) is temperature;
Yi (kg kg
−1) denotes the mass fraction of species
i;
Ri (kg s
−1 m
−3) is the generation or consumption rate of species
i;
Di,m (m
2 s
−1) is the mass diffusivity of species
i.
Both gas phase and surface species can be produced and depleted by surface reactions. The production or consumption rate Ri for each species is written as
|
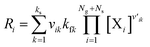 | (6) |
where
ks is the total number of elementary gas phase and surface reactions including adsorption and desorption,
vik and
v′
ik are stoichiometric coefficients (right minus left side of reaction equation and left side, respectively),
kfk is the forward rate coefficient, [X
i] is the concentration of species
i,
Ng is the number of gas phase species and
Ns is the number of surface species. The rate coefficients of adsorption, desorption, and surface reactions can also depend on surface coverage
Θi resulting in the following expression for the rate coefficient of the forward reaction
k:
|
 | (7) |
where
Ak is pre-exponential factor,
βk is temperature exponent, and
Eak is the activation energy of reaction
k. Coefficients
μik and
εik describe the dependence of the rate coefficients on the surface coverage
Θi of species
i.
In the case of gas phase species adsorption reactions on the wall, the forward rate coefficient kfk of the reaction k is calculated using the sticking coefficients as
|
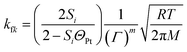 | (8) |
where
Si is adsorption coefficient of species
i,
ΘPt is the free surface coverage,
Γ is the surface site density of the catalyst,
m is the sum of surface species stoichiometric coefficients on the left hand side of adsorption reactions, and
M is molar mass of species
i.
As heat conduction in the wall can affect significantly the combustion characteristics,35 heat transfer in the wall is considered in the present simulation. The energy equation for the solid phase is given as follow:
|
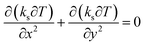 | (9) |
where
ks (W m
−1 K
−1) is the thermal conductivity of solid wall.
2.3. Chemical kinetics
Hetero-/homogeneous combustion of hydrogen–air mixtures at the fuel-lean stoichiometry has been reported in many past studies with the detailed kinetics schemes, including the heterogeneous and homogeneous reaction mechanisms. For example, the fuel-lean hydrogen–air hetero-/homogeneous combustion over platinum was investigated experimentally/numerically in Appel et al.17 and numerically in Brambilla et al.,2 where the detailed transport, gas-phase and surface chemistry were used together with 2-D heat conduction in the solid wall.
In the present work, homogeneous chemical kinetics of hydrogen–air combustion was described by the detailed mechanism of Konnov et al.36 containing 9 gaseous species participating in 21 reversible elementary reactions, it was updated according to the latest kinetic data from Alekseev et al.37 This homogeneous mechanism was tested against shock tube, rapid compression machines, flow reactors, jet-stirred reactors, and flame velocity measurements in Olm et al.38 The heterogeneous reaction of hydrogen oxidation over platinum was described by the detailed scheme of Deutschmann et al.22,39 without any modification. The capacity of this heterogeneous reaction scheme to reproduce measured hetero-/homogeneous ignition characteristics under both fuel-rich and fuel-lean mixtures has been demonstrated in.40–42 Homogeneous and heterogeneous reactions rates were evaluated with CHEMKIN21 and surface-CHEMKIN,43 respectively. While the transport properties along with the mixture-average diffusion (including thermal diffusion) were calculated from the CHEMKIN's transport database.44,45
2.4. Boundary conditions
The incoming hydrogen–air mixture with uniform velocity and concentration profiles at 300 K and pressure of 0.1 MPa were specified at the inlet. Zero-Neumann boundary condition was given at the exit. Non-slip boundary condition was considered for the inner wall. The inlet mass flow rate was set 0.4 kg m−2 s−1. In order to sustain combustion of fuel-lean hydrogen–air over platinum in the range of operating conditions, initial temperature of 1400 K was adapted to initialize the combustion reaction. The heat flux between fluid and solid wall is computed using the Fourier's law, and the continuity in temperature links the fluid and solid phases. Moreover, the radiation between the inner surfaces was considered using the discrete ordinates model. At the outer walls, heat losses to the surroundings are calculated through eqn (10), in which both thermal radiation and natural convection are considered. |
q = h(Tw,o − T∞) + εsσ(Tw,o4 − T∞4)
| (10) |
where h is the natural convection heat transfer coefficient (20 W m−2 K−1),30,31 Tw,o is the outer wall temperature, T∞ is the ambient temperature (300 K), εs is the surface emissivity (0.92), and σ is the Stephan–Boltzmann constant (5.67 × 10−8 W m−2 K−4).
2.5. Computation scheme
CFD software FLUENT20 coupled with CHEMKIN21 was employed for a steady-state reactive flow to solve the mass, energy, momentum and species conservation equations and the conjugated heat conduction in solid walls. The second-order upwind scheme was used to discretize this model, and the SIMPLE algorithm was employed for the pressure–velocity coupling. The uniform grid size of 0.5 μm was used to mesh the numerical models for all scenarios analyzed, and grid-independence of the results has been checked. The convergence of CFD simulation was judged based on the residuals of all governing equations less than 1.0 × 10−6.
3. Results and discussion
3.1. Model validation
The numerical model is validated by comparing the results with the experimental data. A fuel-lean hydrogen–air mixture with an equivalence ratio of φ = 0.28 is considered corresponding to the experimental data of Appel et al.17 The micro-combustor consists of two horizontal ceramic plates. The channel gap distance and length are 7 mm and 300 mm, respectively. The inlet velocity is 2.0 m s−1 leading to an inlet Reynolds number of Re = 1389. The inlet temperature Tin is assumed to be 310 K. The details of the experimental system and method are given in Appel et al.17 Comparisons between predicted and measured temperature distributions across the channel at the streamwise distance x = 25 mm are illustrated in Fig. 2. Differences between predicted and measured temperature ranged from 0.6% to 5.8%. The temperature field prediction is in good agreement with the experimental results, which confirms the reasonable accuracy of the numerical model employed in the present work.
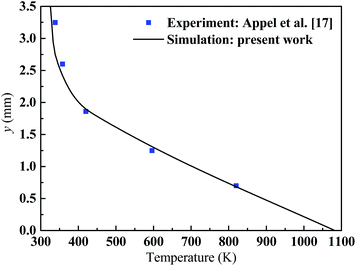 |
| Fig. 2 Comparisons between predicted and measured temperature distributions across the channel at the streamwise distance x = 25 mm. | |
3.2. Effect of channel gap distance
Hydrogen conversion rate profiles as a function of channel gap distance between 0.4 and 1.2 mm in the streamwise direction is shown in Fig. 3. The predicted gaseous conversion rate was computed by integrating the volumetric gaseous hydrogen conversion over the channel height, whereas the catalytic conversion rate accounted for catalytic hydrogen conversion on both channel surfaces. Gaseous conversion rate of hydrogen decreases with decreasing the channel gap distance. Initially, as the channel gap distance is decreased from 1.2 to 0.6 mm, gaseous conversion rate gradually decreases mainly because of the reduced micro-channel volume. In this range, the catalytic conversion rate remains essentially unchanged. However, there is a precipitous decline in gaseous conversion rate as the channel gap distance is further decreased. The catalytic conversion rate also increase for the channel gap distance below 0.6 mm because of the decreasing gaseous conversion rate of hydrogen.
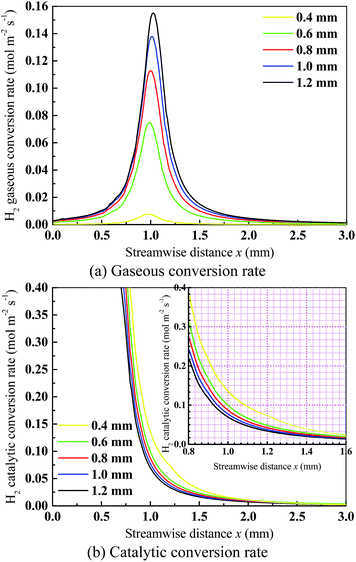 |
| Fig. 3 Conversion rate of hydrogen at five different channel gap distances. | |
In Fig. 4, chemical effect of wall OH radical quenching on homogeneous reaction also exhibits some very interesting trends. As the channel gap distance is decreased from 1.2 to 0.6 mm, the OH radical quenching rate on the wall remains almost unchanged despite the decreasing OH radical concentrations in the gas phase. OH radical adsorption/desorption reactions are removed from the heterogeneous reaction mechanism of Deutschmann et al.22,39 to assess the chemical effect of wall radical quenching on homogeneous reaction. Three different reaction modes by different chemical kinetic schemes are employed. They are Case A: normal simulation, Case B: lower H2O effect in homogeneous reaction mechanism (artificial reduction of H2O enhanced third-body efficiencies), and Case C: OH adsorption/desorption reactions are removed from the heterogeneous reaction mechanism. In Fig. 5, the gaseous conversion rate as a function of channel gap distance for three reaction modes is presented. The simulation results indicate that wall OH radical quenching significantly inhibits the homogeneous reaction. For example, the maximum gaseous conversion rate in 0.6 mm channel increases by about 48% when the OH adsorption/desorption reactions are removed. The chemical effect of wall OH radical quenching will be even more pronounced in 0.4 mm channel. However, this chemical effect becomes less important with the increasing of channel gap distance. When the OH adsorption/desorption reactions are removed, the maximum gaseous conversion rate increases respectively by about 38%, 30% and 24% in 0.8, 1.0 and 1.2 mm channel because of the chemical effect of wall OH radical quenching. Therefore, OH adsorption/desorption reactions become extremely important in smaller channel, and this chemical effect gradually decreases with the increasing of channel gap distance. However, in contrast to the chemical effect of wall OH radical quenching on homogeneous reaction, the axial position where the maximum gaseous conversion rate occurs is not sensitive to the OH adsorption/desorption reactions and only slightly shifted upstream by thermal effect, such as the heat conduction in the wall provides a convenient route for heat transfer from the post-combustion zone to preheat the unburned mixture in the upstream zone. Therefore, chemical effect of wall OH radical quenching on homogeneous reaction for catalytic combustion of hydrogen become extremely important in smaller channel and gradually decreases with the increasing of channel gap distance, while its thermal effect on homogeneous flame almost can be neglected.
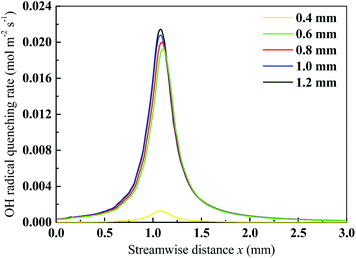 |
| Fig. 4 OH radical quenching rate on the wall at five different channel gap distances. | |
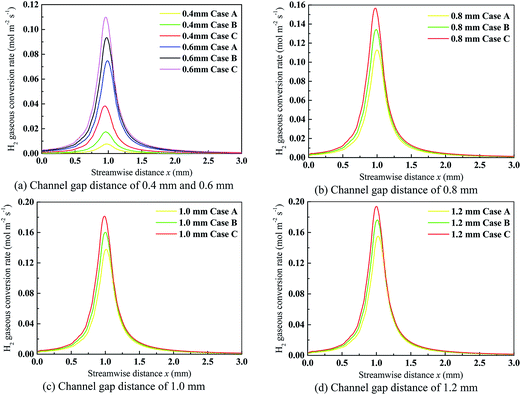 |
| Fig. 5 Gaseous conversion rate of hydrogen at five different channel gap distances; Case A: normal simulation, Case B: lower H2O effect in homogeneous reaction mechanism (artificial reduction of H2O enhanced third-body efficiencies), and Case C: OH adsorption/desorption reactions are removed from the heterogeneous reaction mechanism. | |
The inhibition of homogeneous reaction can be further examined by observing the inlet flow composition. The inlet mass flux of each species is comprised of the convective mass flux and the diffusion mass flux. The diffusive mass transfer in smaller channel generally becomes more significant because of the fuel and air in the bulk gas need less diffusive time to reach the surface of catalytic wall. H2 and H2O concentrations across the channel at the inlet are shown in Fig. 6. The average concentration of hydrogen at the inlet generally decreases with decreasing the channel gap distance. This behavior is because of the stronger diffusive mass flux especially in the wall vicinity in smaller channel, while the average concentration of H2O at the inlet increases because of the stronger diffusion effect. However, H2O concentration close to the wall at the inlet is higher in larger channel, which is dissimilar the monotonic trend of hydrogen. This behavior is because of the relatively smaller diffusion coefficient D of H2O as compared with hydrogen. The diffusive flux JH2O is responsible for the removal of H2O from the wall. The diffusive flux JH2O is not strong enough to wash away H2O from the wall in larger channel. Therefore, a tremendous difference of H2O concentration right at the inlet exists between wall and mid-plane. The decrease in the channel gap distance enhances the diffusive transfer of H2O (surface reaction products) from the wall to the gas phase, which in turn decreases the difference of H2O concentration between them. This decrease in fuel levels and increase in the reaction products at the inlet lead to a change in the reaction rate.
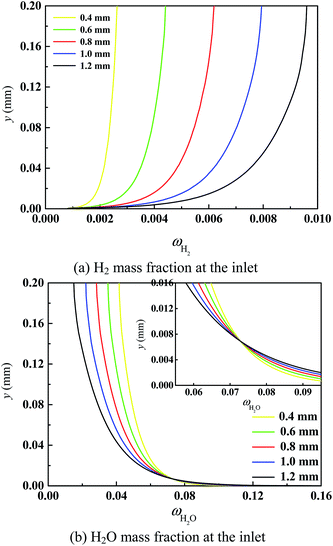 |
| Fig. 6 Concentration profiles of hydrogen and water across the channel at five different channel gap distances. | |
The presence of catalytically-produced water inhibits homogeneous reaction have been demonstrated by Ghermay et al.,14,19 Appel et al.17 and Bui et al.46 As a chain terminating reaction, H + O2 + H2O = HO2 + H2O* is a crucial elementary reaction step in ignition process, which has been discussed in.14,17,19,46–48 As a third-body species, H2O promotes this elementary reaction where active H radical reacts with O2 molecules and produces the relatively inert HO2 radical along with loses some energy. Third body efficiency βH2O of gaseous species H2O in above reaction relative to O2 is decreased from 16.25 to 1.0 by decreasing βH2O from 6.5 to 0.4 to assess the inhibition effect of H2O-induced on homogeneous reaction. The gaseous conversion rate with (Case A) and without (Case B) the addition of above reaction are shown in Fig. 5. The presence of H2O inhibits homogeneous reaction. For example, in 0.6 mm channel, the peak value of gaseous conversion rate is about 24.8% greater in the artificially adjusted βH2O case (Case B) as compared with the result of the normal simulation (Case A). However, in the smallest channel of 0.4 mm, the inhibition effect of H2O-induced on homogeneous reaction is much smaller than the chemical effect of wall OH radical quenching.
The non-dimensional mid-plane temperature profiles as a function of channel gap distance in the streamwise direction are shown in Fig. 7. Mid-plane temperature increases with decreasing the channel size because of stronger diffusion effect in smaller channel. Elevated temperature promotes the homogeneous reaction because it is a strong function of temperature. However, the temperature variation as a result of the change in channel size does not seem to have a significant effect on homogeneous reaction as previously observed. In other words, the rise in gas temperature as a result of smaller channel is not strong enough to compete with the other inhibiting effects.
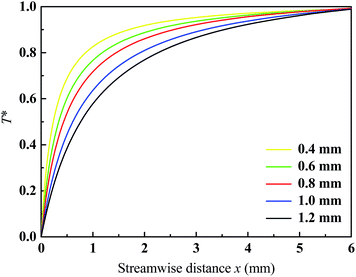 |
| Fig. 7 Non-dimensional mid-plane temperature T* [T* = (T − Tin)/(Tw − Tin)] at five different channel gap distances. | |
3.3. Effect of mass flux
The variation in the inlet mass flux alters the relative significant of the diffusive and convective mass fluxes, which can affect the reactive flow behavior. The gaseous and catalytic conversion rates at different inlet mass flow fluxes are shown in Fig. 8. As observed, the gaseous conversion rate initially increases as the inlet mass flow flux is increased and then decreases as it is further increased. However, the catalytic conversion rate increases with increasing the inlet mass flow flux. The H(s) and H2O(s) surface coverage (ΘH(s) and ΘH2O(s)) as a function of inlet mass flux are shown in Fig. 9. As the inlet mass flow flux is increased, there is increased finite-rate chemistry (i.e. slightly higher concentration of hydrogen just above the catalyst surface), which in turn leads to higher levels of ΘH(s) and ΘH2O(s) on the catalytic wall. The relative contribution of homogeneous and heterogeneous reactions to the total hydrogen conversion are shown in Table 2. It is apparent in all cases that the heterogeneous reaction achieved complete dominance in catalytic micro-channels. The supply of hydrogen increases linearly with increasing the inlet mass flux, while the relative importance of homogeneous reaction rapidly declines to the negligible levels as compared with heterogeneous reaction. Initially, the gaseous conversion rate of hydrogen increases as the inlet mass flux is increased to 0.4 kg m−2 s−1 and then decreases as it is further increased. This behavior is because of the highly non-linear interactions between the convection and diffusion fluxes, transport properties along with homogeneous and heterogeneous reaction kinetics.
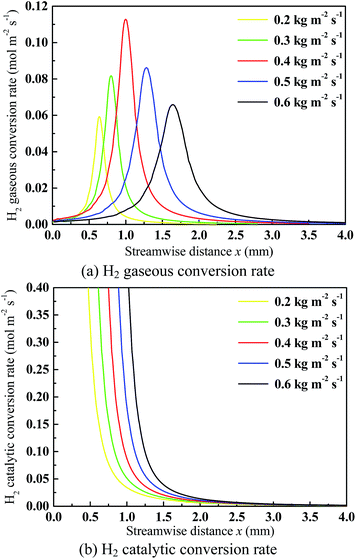 |
| Fig. 8 Conversion rate of hydrogen at five different inlet mass flow fluxes. | |
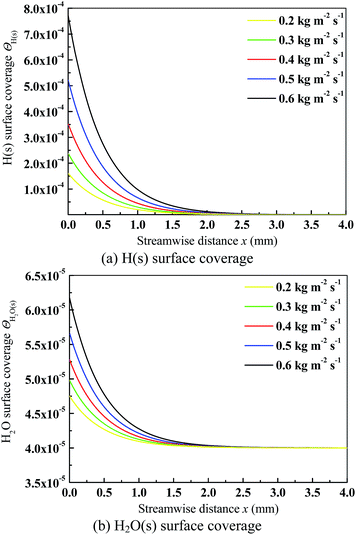 |
| Fig. 9 Surface coverage of H(s) and H2O(s) at five different inlet mass fluxes. | |
Table 2 H2 gaseous and catalytic conversion rates at different inlet mass fluxes
J (kg m−2 s−1) |
0.2 |
0.3 |
0.4 |
0.5 |
0.6 |
Gaseous conversion rate (×106 kg s−1) |
0.047 |
0.084 |
0.098 |
0.088 |
0.062 |
Catalytic conversion rate (×106 kg s−1) |
0.744 |
1.208 |
1.722 |
2.448 |
3.346 |
Percentage of gaseous contribution (%) |
9.8 |
8.2 |
6.4 |
4.4 |
2.2 |
Percentage of catalytic contribution (%) |
90.2 |
91.8 |
93.6 |
95.6 |
97.8 |
As the inlet mass flux is lower, homogeneous reaction is affected by heterogeneous reaction and it can still be sustained in catalytic micro-channels; the heterogeneous reaction rate obviously surpasses the homogeneous reaction rate. As the inlet mass flux is increased, the heterogeneous reaction is continued to enhance and becomes the dominant reaction. Homogeneous flame cannot be sustained in catalytic micro-channels. However, Chen et al.10 and Appel et al.17 found that, even in this situation, homogeneous reaction still affect the expression of intermediate species. As the inlet mass flux is further increased, the homogeneous reaction is completely inhibited to have almost no effects.
The cross-stream mass fraction profiles of hydrogen at axial locations where the gaseous conversion rates peak for the aforementioned inlet mass fluxes (Fig. 8(a)) are shown in Fig. 10. This trend observed in the available hydrogen for homogeneous reaction is consistent with the results of homogeneous reaction contribution as observed in Table 2 and Fig. 8. Initially, as the inlet mass flux is increased from 0.2 to 0.4 kg m−2 s−1, the available hydrogen for homogeneous reaction also increases. However, as it is further increased from 0.4 to 0.6 kg m−2 s−1, the enhanced heterogeneous reaction depletes the available hydrogen and seriously inhibits homogeneous reaction. As observed (Table 2, red lines in Fig. 8(a) and 10), the available hydrogen for homogeneous reaction is the largest for 0.4 kg m−2 s−1 at the axial location of peak gaseous conversion rate, although its supply of hydrogen at the inlet is lower than 0.6 kg m−2 s−1.
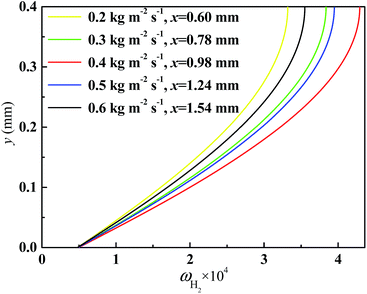 |
| Fig. 10 Cross-stream concentration profiles of hydrogen at axial location of maximum gaseous conversion. | |
The variety of inlet mass flux also affects the temperature distribution, which can inhibit homogeneous reaction in turn. As the inlet mass flux increases, the temperature at every axial location decreases as observed in Fig. 11 where the non-dimensional mid-plane temperature profiles are shown. Therefore, besides the decline in the available hydrogen for homogeneous reaction, the lower temperature levels also inhibit homogeneous reaction as the inlet mass flux is increased.
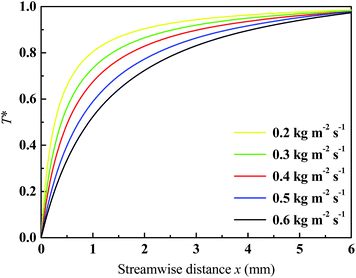 |
| Fig. 11 Non-dimensional mid-plane temperature T* [T* = (T − Tin)/(Tw − Tin)] at five different inlet mass fluxes. | |
OH concentration profiles at different inlet mass fluxes are shown in Fig. 12. Initially, OH concentration increases with increasing the inlet mass flux from 0.2 to 0.4 kg m−2 s−1. However, its level drops to a considerable degree as the inlet mass flux further increases from 0.4 to 0.6 kg m−2 s−1. This behavior is consistent with the homogeneous reaction levels at different inlet mass fluxes as observed earlier in Fig. 8(a), 10 and Table 2. The higher contribution of homogeneous reaction to total hydrogen conversion can produce more OH radical in the gas phase. As the inlet mass flux is increased, the location of the highest OH concentration slightly shifts downstream, as well as the homogeneous reaction zone gradually stretched and expanded in the streamwise direction.
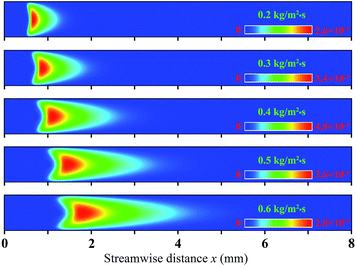 |
| Fig. 12 Contours of OH mass fraction at five different inlet mass fluxes. | |
3.4. Effect of equivalence ratio
In the case of lower equivalence ratio, both homogeneous and heterogeneous reaction rates are generally lower as the result of the absence of enough fuel, although oxygen is oversupply. This result can be confirmed in Fig. 13 where the gaseous and catalytic conversion rates of hydrogen are illustrated in the streamwise direction at different inlet equivalence ratios. In the case of relatively richer mixture (φ = 0.6) but still below the stoichiometric ratio, the gaseous and catalytic conversion rates are higher as expected. The increase in the relative contribution of homogeneous reaction to total hydrogen conversion can be distinctly detected at φ = 0.6 by observing the sharply decline in the catalytic conversion rate in Fig. 13. Since the hydrogen mainly gets depleted by the heterogeneous reaction, leaner hydrogen–air mixture exhibits the monotonic decrease in the catalytic conversion rate in the streamwise direction. However, as the homogeneous reaction at φ = 0.6 initiates, the sharp decline occurs in the catalytic conversion rate because of the consumption of homogeneous reaction becomes significant. The comparison of simulations with and without homogeneous reaction is carried out to examine the relative contribution of homogeneous reaction to total hydrogen conversion at different equivalence ratios. The distribution of hydrogen concentration across the channel with and without considering homogeneous reaction at x = 0.98 mm are shown in Fig. 14. The error associated with neglecting homogeneous reaction is much higher in the case of relatively richer mixture (φ = 0.6). This effect can also be confirmed by observing the total behavior in hydrogen conversion. The hydrogen consumption in the case of relatively richer mixture (φ = 0.6) with and without considering homogeneous reaction are shown in Fig. 15. Close to the inlet section, the two simulations predict almost identical results because fuel is mostly consumed by heterogeneous reaction and homogeneous reaction almost can be neglected. However, as the homogeneous reaction initiates, the difference in fuel consumption predictions becomes apparent. In this case, the largest difference in fuel consumption is about 3.8% at axial location x = 0.94 mm. Therefore, in this case, the homogeneous reaction of hydrogen can be ignored in catalytic micro-channels at the less precision levels.
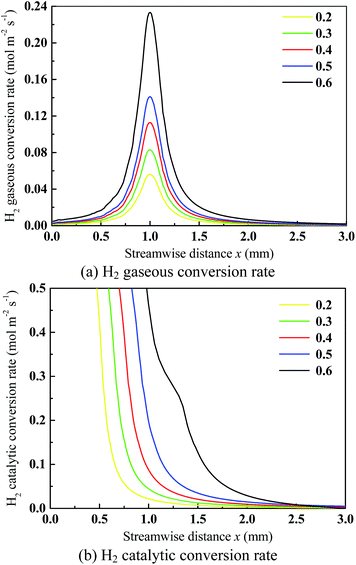 |
| Fig. 13 Gaseous and catalytic conversion rate profiles of hydrogen at different inlet equivalence ratios. | |
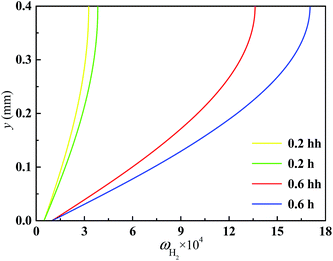 |
| Fig. 14 Mass fraction profiles of hydrogen at initial φ = 0.2 and 0.6, axial location x = 0.98 mm; hh: both homogeneous and heterogeneous reactions, h: heterogeneous reaction alone. | |
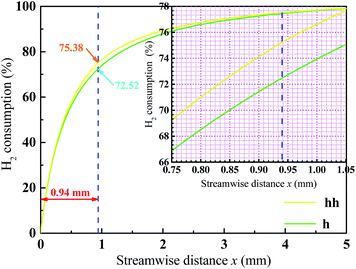 |
| Fig. 15 H2 consumption profiles at initial φ = 0.6; hh: both homogeneous and heterogeneous reactions, h: heterogeneous reaction alone. | |
4. Conclusions
Interaction between homogeneous and heterogeneous reactions of fuel-lean hydrogen–air mixture over platinum in a catalytic planar micro-channel was investigated numerically to assess the relative importance and respective contribution between homogeneous and heterogeneous reactions, employing detailed homogeneous and heterogeneous reaction mechanisms. The main achieved results of this work abbreviate as follows:
• Homogeneous reaction contribution to total hydrogen conversion reduces with decreasing the channel size. As the channel size is decreased from 1.2 to 0.6 mm, the decline in homogeneous reaction is gradual because of the decreased channel volume and the increased adsorption of OH radical on the wall, while heterogeneous reaction remains basically unchanged.
• Chemical effect of wall OH radical quenching on homogeneous reaction become extremely important in smaller channel and gradually decreases with increasing the channel size, while its thermal effect on homogeneous flame almost can be neglected.
• As the channel size is decreased, the upstream diffusion of product species becomes significant as a result of the stronger diffusive mass flux in smaller channel. This effect leads to the existence of product species, which contributes even further to inhibit homogeneous reaction.
• Homogeneous reaction rate increases with increasing the mass flux even though its contribution to total hydrogen conversion is decreased. However, this behavior reverses after a certain mass flux, which results in further decline in homogeneous reaction contribution to the negligible levels. Meanwhile, heterogeneous reaction contribution increases steadily different from homogeneous reaction.
• Equivalence ratio significantly affect the relative contribution between homogeneous and heterogeneous reactions.
• Even though the increase of homogeneous reaction is more vigorous, both homogeneous and heterogeneous reactions increase as the equivalence ratio is increased but below the stoichiometric ratio. For hydrogen fuel at φ = 0.6, the deviation caused by ignoring the homogeneous reaction is not more than 3.8%. Therefore, in this case, the homogeneous reaction of hydrogen can be ignored in catalytic micro-channels at the less precision levels.
References
- Y. Ju and K. Maruta, Microscale combustion: technology development and fundamental research, Prog. Energy Combust. Sci., 2011, 37, 669–715 CrossRef CAS PubMed.
- A. Brambilla, C. E. Frouzakis, J. Mantzaras, A. Tomboulides, S. Kerkemeier and K. Boulouchos, Detailed transient numerical simulation of H2/air hetero-/homogeneous combustion in platinum-coated channels with conjugate heat transfer, Combust. Flame, 2014, 161, 2692–2707 CrossRef CAS PubMed.
- C. Miesse, R. Masel, M. Short and M. Shannon, Experimental observations of methane–oxygen diffusion flame structure in a sub-millimetre microburner, Combust. Theory Modell., 2005, 9, 77–92 CrossRef CAS PubMed.
- J. Ahn, C. Eastwood, L. Sitzki and P. D. Ronney, Gas-phase and catalytic combustion in heat-recirculating burners, Proc. Combust. Inst., 2005, 30, 2463–2472 CrossRef PubMed.
- S. Karagiannidis, J. Mantzaras and K. Boulouchos, Stability of hetero-/homogeneous combustion in propane- and methane-fueled catalytic microreactors: channel confinement and molecular transport effects, Proc. Combust. Inst., 2011, 33, 3241–3249 CrossRef CAS PubMed.
- G. Pizza, J. Mantzaras, C. E. Frouzakis, A. G. Tomboulides and K. Boulouchos, Suppression of combustion instabilities of premixed hydrogen/air flames in microchannels using heterogeneous reactions, Proc. Combust. Inst., 2009, 32, 3051–3058 CrossRef CAS PubMed.
- G. Pizza, J. Mantzaras and C. E. Frouzakis, Flame dynamics in catalytic and non-catalytic mesoscale microreactors, Catal. Today, 2010, 155, 123–130 CrossRef CAS PubMed.
- D. G. Norton and D. G. Vlachos, Combustion characteristics and flame stability at the microscale: a CFD study of premixed methane/air mixtures, Chem. Eng. Sci., 2003, 58, 4871–4882 CrossRef CAS PubMed.
- E. Miyata, N. Fukushima, Y. Naka, M. Shimura, M. Tanahashi and T. Miyauchi, Direct numerical simulation of micro combustion in a narrow circular channel with a detailed kinetic mechanism, Proc. Combust. Inst., 2015, 35, 3421–3427 CrossRef CAS PubMed.
- G. B. Chen, C. P. Chen, C. Y. Wu and Y. C. Chao, Effects of catalytic walls on hydrogen/air combustion inside a micro-tube, Appl. Catal., A, 2007, 332, 89–97 CrossRef CAS PubMed.
- Y. Wang, Z. Zhou, W. Yang, J. Zhou, J. Liu, Z. Wang and K. Cen, Combustion of hydrogen–air in micro combustors with catalytic Pt layer, Energy Convers. Manage., 2010, 51, 1127–1133 CrossRef CAS PubMed.
- C. T. Goralski Jr and L. D. Schmidt, Modeling heterogeneous and homogeneous reactions in the high-temperature catalytic combustion of methane, Chem. Eng. Sci., 1999, 54, 5791–5807 CrossRef.
- P. Aghalayam, P.-A. Bui and D. G. Vlachos, The role of radical wall quenching in flame stability and wall heat flux: hydrogen–air mixtures, Combust. Theory Modell., 1998, 2, 515–530 CrossRef CAS.
- Y. Ghermay, J. Mantzaras and R. Bombach, Effects of hydrogen preconversion on the homogeneous ignition of fuel-lean H2/O2/N2/CO2 mixtures over platinum at moderate pressures, Combust. Flame, 2010, 157, 1942–1958 CrossRef CAS PubMed.
- S. Karagiannidis, J. Mantzaras, G. Jackson and K. Boulouchos, Hetero-/homogeneous combustion and stability maps in methane-fueled catalytic microreactors, Proc. Combust. Inst., 2007, 31, 3309–3317 CrossRef PubMed.
- S. A. Fanaee and J. A. Esfahani, Two-dimensional analytical model of flame characteristic in catalytic micro-combustors for a hydrogen–air mixture, Int. J. Hydrogen Energy, 2014, 39, 4600–4610 CrossRef CAS PubMed.
- C. Appel, J. Mantzaras, R. Schaeren, R. Bombach, A. Inauen, B. Kaeppeli, B. Hemmerling and A. Stampanoni, An experimental and numerical investigation of homogeneous ignition in catalytically stabilized combustion of hydrogen/air mixtures over platinum, Combust. Flame, 2002, 128, 240–368 CrossRef.
- W. Y. Choi, S. J. Kwon and H. Shin, Combustion characteristics of hydrogen–air premixed gas in a sub-millimeter scale catalytic combustor, Int. J. Hydrogen Energy, 2008, 33, 2400–2408 CrossRef CAS PubMed.
- Y. Ghermay, J. Mantzaras, R. Bombach and K. Boulouchos, Homogeneous combustion of fuel-lean H2/O2/N2 mixtures over platinum at elevated pressures and preheats, Combust. Flame, 2011, 158, 1491–1506 CrossRef CAS PubMed.
- Fluent 6.3 user's guide, Fluent Inc., Lebanon, New Hampshire, 2006 Search PubMed.
- R. J. Kee, F. M. Rupley, E. Meeks and J. A. Miller, Chemkin: a Fortran chemical kinetics package for the analysis of gas-phase chemical kinetics, Report No. SAND96-8216, Technical Report, Sandia National Laboratories, 1996 Search PubMed.
- O. Deutschmann, L. I. Maier, U. Riedel, A. H. Stroemman and R. W. Dibble, Hydrogen assisted catalytic combustion of methane on platinum, Catal. Today, 2000, 59, 141–150 CrossRef CAS.
- I. Sen and A. K. Avci, Simulation of exhaust gas reforming of propane in a heat exchange integrated microchannel reactor, Int. J. Hydrogen Energy, 2014, 39, 844–852 CrossRef CAS PubMed.
- M. Karakaya, S. Keskin and A. K. Avci, Parametric study of methane steam reforming to syngas in a catalytic microchannel reactor, Appl. Catal., A, 2012, 411–412, 114–122 CrossRef CAS PubMed.
- A. K. Avci, D. L. Trimm and M. Karakaya, Microreactor catalytic combustion for chemicals processing, Catal. Today, 2010, 155, 66–74 CrossRef CAS PubMed.
- M. Karakaya and A. K. Avci, Microchannel reactor modeling for combustion driven reforming of iso-octane, Int. J. Hydrogen Energy, 2011, 36, 6569–6577 CrossRef CAS PubMed.
- X. Karatzas, J. Dawody, A. Grant, E. E. Svensson and L. J. Pettersson, Zone-coated Rh-based monolithic catalyst for autothermal reforming of diesel, Appl. Catal., B, 2011, 101, 226–238 CrossRef CAS PubMed.
- M. Lyubovsky, L. L. Smith, M. Castaldi, H. Karim, B. Nentwick, S. Etemad, R. LaPierre and W. C. Pfefferle, Catal. Today, 2003, 83, 71–84 CrossRef CAS.
- R. E. Hayes, S. T. Kolaczkowskib, P. K. C. Li and S. Awdry, Evaluating the effective diffusivity of methane in the washcoat of a honeycomb monolith, Appl. Catal., B, 2000, 25, 93–104 CrossRef CAS.
- J. Wan, A. Fan, H. Yao, W. Liu, X. Gou and D. Zhao, The impact of channel gap distance on flame splitting limit of H2/air mixture in microchannels with wall cavities, Int. J. Hydrogen Energy, 2014, 39, 11308–11315 CrossRef CAS PubMed.
- J. Wan, W. Yang, A. Fan, Y. Liu, H. Yao, W. Liu, Y. Du and D. Zhao, A numerical investigation on combustion characteristics of H2/air mixture in a micro-combustor with wall cavities, Int. J. Hydrogen Energy, 2014, 39, 8138–8146 CrossRef CAS PubMed.
- A. Brambilla, C. E. Frouzakis, J. Mantzaras, R. Bombach and K. Boulouchos, Flame dynamics in lean premixed CO/H2/air combustion in a mesoscale channel, Combust. Flame, 2014, 161, 1268–1281 CrossRef CAS PubMed.
- H.-Y. Shih and C.-R. Liu, A computational study on the combustion of hydrogen/methane blended fuels for a micro gas turbines, Int. J. Hydrogen Energy, 2014, 39, 15103–15115 CrossRef CAS PubMed.
- M. Baigmohammadi, S. Tabejamaat and J. Zarvandi, Numerical study of the behavior of methane–hydrogen/air pre-mixed flame in a micro reactor equipped with catalytic segmented bluff body, Energy, 2015, 85, 117–144 CrossRef CAS PubMed.
- D. G. Norton, E. D. Wetzel and D. G. Vlachos, Fabrication of single-channel catalytic microburners:
effect of confinement on the oxidation of hydrogen/air mixtures, Ind. Eng. Chem. Res., 2004, 43, 4833–4840 CrossRef CAS. - A. A. Konnov, Remaining uncertainties in the kinetic mechanism of hydrogen combustion, Combust. Flame, 2008, 152, 507–528 CrossRef CAS PubMed.
- V. A. Alekseev, M. Christensen and A. A. Konnov, The effect of temperature on the adiabatic burning velocities of diluted hydrogen flames: a kinetic study using an updated mechanism, Combust. Flame, 2015, 162, 1884–1898 CrossRef CAS PubMed.
- C. Olm, I. Gy. Zsély, R. Pálvölgyi, T. Varga, T. Nagy, H. J. Currac and T. Turányi, Comparison of the performance of several recent hydrogen combustion mechanisms, Combust. Flame, 2014, 161, 2219–2234 CrossRef CAS PubMed.
- O. Deutschmann, R. Schmidt, F. Behrendt and J. Warnatz, Numerical modeling of catalytic ignition, Symposium (International) on Combustion, 1996, 26, 1747–1754 CrossRef.
- X. Zheng, J. Mantzaras and R. Bombach, Kinetic interactions between hydrogen and carbon monoxide oxidation over platinum, Combust. Flame, 2014, 161, 332–346 CrossRef CAS PubMed.
- M. Schultze, J. Mantzaras, R. Bombach and K. Boulouchos, An experimental and numerical investigation of the hetero-/homogeneous combustion of fuel-rich hydrogen/air mixtures over platinum, Proc. Combust. Inst., 2013, 34, 2269–2277 CrossRef CAS PubMed.
- X. Zheng and J. Mantzaras, An analytical and numerical investigation of hetero-/homogeneous combustion with deficient reactants having larger than unity Lewis numbers, Combust. Flame, 2014, 161, 1911–1922 CrossRef CAS PubMed.
- M. E. Coltrin, R. J. Kee, F. M. Rupley and E. Meeks, Surface Chemkin: a Fortran package for analyzing heterogeneous chemical kinetics at a solid surface/gas-phase interface, Report No. SAND96-8217, Technical Report, Sandia National Laboratories, 1996.
- J. A. Manion, R. E. Huie, R. D. Levin, D. R. Burgess Jr, V. L. Orkin, W. Tsang, W. S. McGivern, J. W. Hudgens, V. D. Knyazev, D. B. Atkinson, E. Chai, A. M. Tereza, C.-Y. Lin, T. C. Allison, W. G. Mallard, F. Westley, J. T. Herron, R. F. Hampson and D. H. Frizzell, NIST Chemical Kinetics Database, NIST Standard Reference Database 17, Version 7.0 (Web Version), Release 1.6.8, Data version 2013.03, National Institute of Standards and Technology, Gaithersburg, Maryland, 20899-8320, web address: http://kinetics.nist.gov/ Search PubMed.
- R. J. Kee, G. Dixon-Lewis, J. Warnatz, M. E. Coltrin and J. A. Miller, A Fortran computer code package for the evaluation of gas-phase multicomponent transport properties, Report No. SAND86-8246, Technical Report, Sandia National Laboratories, 1996 Search PubMed.
- P.-A. Bui, D. G. Vlachos and P. R. Westmoreland, Catalytic ignition of methane/oxygen mixtures over platinum surfaces: comparison of detailed simulations and experiments, Surf. Sci., 1997, 385, 1029–1034 CrossRef.
- D. G. Vlachos, L. D. Schmidt and R. Aris, Ignition and extinction of flames near surfaces: combustion of H2 in air, Combust. Flame, 1993, 95, 313–335 CrossRef CAS.
- D. G. Norton and D. G. Vlachos, Hydrogen assisted self-ignition of propane/air mixtures in catalytic microburners, Proc. Combust. Inst., 2005, 30, 2473–2480 CrossRef PubMed.
|
This journal is © The Royal Society of Chemistry 2015 |