DOI:
10.1039/C5RA07400B
(Paper)
RSC Adv., 2015,
5, 63175-63185
Modeling of spherical silver nanoparticles in silicone-based nanocomposites for marine antifouling†
Received
23rd April 2015
, Accepted 9th July 2015
First published on 9th July 2015
Abstract
Since the use of organotin antifouling paints was prohibited in 2003, researchers have endeavored to develop novel environment-friendly marine antifouling coatings. We report the successful fabrication of model silicone foul-release (FR) coatings with elastomeric polydimethylsiloxane (PDMS)/spherical silver (Ag) nanocomposites. This design integrates two inhibition modes of (1) chemical inertness and (2) the physical repelling force of microfouling. The antifouling nanocomposite models were successfully synthesized via the solution casting technique. In this approach, a series of filler concentrations of Ag nanoparticles (NPs) with a particle size of <10 nm and spherical morphology facet dominantly controlled on the {111} lattice plane was used to control the antifouling models. The surface hydrophobicity, roughness, and free energy properties of the nanocomposites were systematically studied as fouling non-stick factors. The physicomechanical properties were also assessed. Selected bacterial strains were used as microfoulants for a laboratory assay investigation for 30 days. Our findings provide important insights into how subtle structural changes in polymer nanocomposites can considerably improve biological activity and simplify surface cleaning. Hydrophobicity, surface inertness, fouling resistance, and surface easy-cleaning properties significantly improved in the nanocomposite design models fabricated with nanofiller loadings of up to 0.1% spherical Ag NPs without changes in the bulk mechanical properties. The fabricated models were subjected to a rigorous test in a field trial in Red Sea water. The results show the potential of our models based on Ag nanofillers up to 0.1% for ecologically friendly antifouling coatings as an alternative to traditional systems. The PDMS/Ag composite models have a long-term durability and antifouling performance, which are important factors for developing effective, stable, and eco-friendly nanocomposites.
1. Introduction
In recent years, the physical, chemical and mechanical properties of nanomaterials have considerably improved their potential applications ranging from environment and energy to healthcare compared with those of bulk materials.1 International ecological restrictions have directed research toward developing environment-friendly nanomaterials for biofield applications.2 The environmental exposure of nanomaterials is inevitable because of their widespread use in many technological and biological fields.3,4 As various nanomaterials have been developed for marine applications, marine fouling represents a major economic and ecological problem worldwide.
Biofouling is a complex issue in marine fields, particularly in marine transportation.5,6 Marine biofouling increases drag resistance and decreases sailing speed to a minimum, thereby increasing fuel consumption and maintenance leading to poisonous gas emissions to the atmosphere.7,8 Antifouling (AF) paints have been widely used to prevent and control the biofouling of marine structures. AF coatings based on organotin compounds pose a threat to the marine environment.9 As the use of biocidal AF technologies has been restricted because of their bioaccumulative properties and high toxicity to various marine organisms, less or even nontoxic AF paints must be developed.10 Many researchers have focused on TBT-free AF paints, but these materials are also deleterious to the environment.11,12 Toxicity and concerns about the application of AF biocidal paints have impelled the development of novel environment-friendly materials based on non-stick physical anti-adhesion mechanisms.13 Non-stick FR coatings, which are a viable, commercial, and nontoxic alternative, function by providing many factors including low surface free energy, low glass transition temperature, low microroughness and high mobility of the polymer chains; hence, fouling species cannot securely attach on the surface and can be easily removed hydrodynamically.14,15
Silicone FR coatings based on polydimethylsiloxane (PDMS) feature non-toxicity, low microroughness, low surface tension, low glass transition temperature (Tg), high molecular mobility, water repellant and non-leachant properties, stability in marine water, and excellent adhesion properties onto various hull surfaces; these characteristics inhibit adhesion of fouling organisms onto the surface.16,17 Although PDMS coatings exhibit good FR effect, nanofillers are added to improve FR performance. As a trade-off exists between FR and durability, the challenge is to improve the durability of FR coatings without affecting their mechanical properties. Thus, this research introduces novel FR coating models.
The use of noble metal nanoparticles (NPs), particularly nano-Ag, has received great attention in the fields of physics, chemistry, materials, and sensors.18 Although nano-Ag is less expensive and presents excellent optical properties compared with nanogold, it has rarely been studied and applied. Nano-Ag particles have received great attention for many industrial applications, particularly in the paint industry, because of their superior physical and biological properties, such as antimicrobial characteristics.19 Among various antibacterial agents, Ag NPs are highly favorable because of their high toxicity to a broad spectrum of microorganisms but low cytotoxicity to higher animals.20,21 The high surface-area-to-volume ratio of NPs contributes to their unique physical, chemical, mechanical, and quantum size effect properties. The anti-fungal,22 antiviral,23 antiangiogenisis,24 and AF activities of Ag NPs have been highlighted in several studies.25 Spherical Ag NPs with an average particle size of 20 ± 0.21 nm have been shown to exert strong antimicrobial and antioxidant activities in biological applications against bacteria, such as Staphylococcus aureus and Escherichia coli.26 Spherical Ag NPs that mainly contain {111} facets exhibit the most significant antibacterial activity over other Ag nanostructures (cubic, wire, and triangular), which contain few {111} planes, against E. coli and Bacillus microorganisms.27 The polar properties of edged Ag spheres with densely packed {111} lattice planes, which exhibit the lowest surface energy per unit area and stability over the {100} and {110} facets of other morphologies, contribute to the FR and antibacterial properties.28 Small-sized particles also exhibit antibacterial properties against bacteria. The smallest Ag NPs with dimensions less than 10 nm exert the highest antibacterial activity because they contain numerous {111} facets.29 The stability and dispersion of Ag NPs could be enhanced through functionalization of the hydrophobic hydrocarbon chain of dodecylamine, thereby preventing aggregation.30 Ag NPs also enhance the hydrophobicity of films.31 Thus, the use of modified nano-Ag films to develop hydrophobic surfaces poses a challenge for the current field of study.
In this study, we fabricated low-cost, non-stick, and nontoxic PDMS/spherical nanostructured surfaces through solution casting to be used as novel FR coating surfaces. This study is the first to use solution casting to fabricate such composites. Stable spherical Ag NPs with an average particle size of <10 nm were synthesized via sol–gel technique. Various concentrations of Ag NPs were prepared by directly blending the desired amount in the PDMS matrix to compare and identify the most effective concentration. The bulk mechanical properties were evaluated using tensile modulus measurements. The surface properties were also quantitatively determined through measurements of contact angle, roughness values, and surface free energy. Spherical Ag plays a novel role of increasing FR properties in marine AF paints by improving the super-hydrophobicity and producing an inert silicone nanocomposite surface with low energy. Given these properties, fouling organisms cannot settle on the surface without being killed. The well-dispersed nanofillers in the polymer matrix enhance the surface property and stability of the bulk mechanical properties, thereby producing smooth surfaces, which can prevent fouling adhesion through a failure adhesion mechanism. Several microorganisms were used to investigate the FR performance of the prepared nanocomposites. A field trial was also conducted to examine the AF performance in natural sea water for 12 months. The proposed nanocomposite system could be potentially used to completely control fouling via a nontoxic method through a physical anti-adhesion mechanism.
2. Experimental details
2.1. Chemicals
Octamethylcyclotetrasiloxane (D4, 98%), tetramethyldivinyldisiloxane (C8H18OSi2, 97%), polyhydromethylsiloxane (PMHS; Mn = 1700–3200), Karstedt catalyst (platinum (0), divinyltetramethyldisiloxane in solution; Pt content: 8–11%), silver acetate (99%), phenyl hydrazine (97%), and dodecylamine (DDA, 98%) were purchased from Sigma-Aldrich Chemical Co. Ltd, United States. Trichloroethylene, KOH, methylbenzene, ethanol, acetone, and methanol were obtained from Merck Co., Mumbai, India and used without further purification.
2.2. One-step fabrication of spherical Ag NPs
Spherical Ag NPs were synthesized through a one-step chemical reduction-stabilizing technique by using phenyl hydrazine (substituted hydrazine) and 1-dodecylamine as suitable reducing and stabilizing agents, respectively, at a relatively low temperature range of 50–60 °C.32–35 In this approach, unique and spherical Ag NPs with an average particle size of <10 nm were obtained under specific synthesis conditions with controlled concentrations of the silver precursor, reducing and stabilizing agents, and pH solution as follows: phenyl hydrazine (1 mmol) solution in 10 mL of methylbenzene was added dropwise to a mixture of silver acetate (1 mmol) solution in 40 mL of methylbenzene and DDA (10 mmol) with continuous stirring at 50 °C to 60 °C (pH 10.5) for 1 h to prepare spherical Ag NPs with particle sizes lower than 10 nm. After the addition of methanol and acetone (50
:
50 in volume) to the mixture, black–silver DDA NPs precipitated. The precipitates were filtered, washed with a mixture of acetone and ethanol, and then dried in air (Scheme 1).
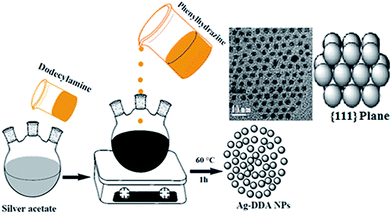 |
| Scheme 1 Preparation of spherical Ag NPs through a one-step chemical reduction-stabilizing technique by using phenyl hydrazine and 1-dodecylamine as reducing and stabilizing agents, respectively, at a relatively low temperature range of 50–60 °C. | |
2.3. Fabrication of FR model based on novel spherical PDMS/Ag nanocomposites
Linear PDMS with vinyl terminal groups (VTPDMS) was prepared to fabricate the novel spherical PDMS/Ag nanocomposites. The preparation was performed through the anionic ring-opening polymerization of octamethylcyclotetrasiloxane, as we reported recently.16 Ag NPs with different concentrations (0.01%, 0.05%, 0.1%, 0.5%, 1%, 3%, and 5%) were sonicated in methylbenzene using a VCX-750 (Sonics and Materials Co., USA) with an ice bath for 15 min. A solution of the VTPDMS resin in methylbenzene was slowly added, stirred for 20 min, and then sonicated for a further 15 min. A solution containing VTPDMS (15 g in 50 mL of methylbenzene) and Ag NPs was subjected to hydrosilation crosslinking using polyfunctional silicon hydride PMHS (curing agent, 0.5 g in 10 mL of methylbenzene) in the presence of Karstedt catalyst (0.55 g in 20 mL of trichloroethylene) through the hydrosilation pathway of curing.16,36
A fabricated coating formulation (VTPDMS 79.5%, 0.1% Ag NPS, 10% ferric oxide pigment (95%, SDFCl, India), and 0.5% surfactant) was applied over the epoxy primer first coat and tie coat layer after drying. Free-standing films with a thickness of 150 μm were applied. The top-coat spherical Ag/silicone nanocomposite film was left for 24 h at RT for complete curing.
2.4. Characterization of the Ag NPs and FR model based on PDMS/Ag NP nanocomposites
Various characterization techniques were performed on the fabricated Ag NPs and FR model based on PDMS/Ag NP nanocomposites.
The crystalline nature of the prepared Ag NPs was determined through XRD, and the data were collected using a PANalytical instrument (X’Pert PRO, Netherlands) equipped with monochromated CuKα radiation (λ = 0.15406 nm) at an accelerating voltage of 30 kV. Scanning was performed in 2 theta angle that ranged from 10° to 70° with d-spacing values between 0.144 and 0.235 nm, and the data obtained were identified in accordance with the ICDD database.
Samples for high-resolution TEM were prepared by placing a drop of the sonicated Ag NPs (in ethyl alcohol) on a carbon-coated copper grid (300 mesh) to evaluate the morphology and size. TEM images were collected with an electron microscope (JEM2100 LaB6, Japan) at 200 kV accelerated voltage with 1.4 Å point resolution. The Ag/silicone nanocomposite films were cut into ultra-thin sections of 100–150 nm thickness using a Leica Ultracut UCT ultracryomicrotome (Leica Microsystems, Austria). These sections were cut with a 45° sharp diamond cryoknife at −160 °C. The cryoslices were collected serially onto a copper-mesh TEM grid and analyzed at 160 kV acceleration voltage.
The elemental composition of the Ag NPs was evaluated using an EDS instrument (Oxford X-Max, Birth) directly connected to an electron microscope (JEM2100 LaB6) at an acceleration voltage of 30 kV.
The morphology of the as-synthesized Ag NPs was captured using a scanning electron microscope (JEOL JSM530) at an accelerating voltage of 20 keV.
The optical micrographs of the immersed coated glass samples were obtained using a polarized microscope (Olympus BH-2, Japan).
2.5. Surface and release performance of the PDMS/Ag nanocomposites
A Tantec line of contact angle goniometer (Germany) was used to measure the static contact angles of liquids on the as-synthesized cured polymer and Ag/silicone nanocomposites. Measurements were performed on coated microscopic slides through the sessile drop technique. The solid surface free energy of the coated surfaces was determined based on the Fox–Zisman theory using static contact angle measurements; the Owens, Wendt, Rabel, and Kaelble (OWRK) method, also called the geometric mean method, was employed for measurement.37 The liquids used were DI water and diiodomethane. The total surface tension γtotalS measurements of the cured polymer films and nanocomposite surfaces were calculated using the OWRK method with the following (eqn (1) and (2)): |
 | (1) |
where θL and γL are the static contact angle and surface tension values of the testing liquids, respectively; γD and γP are the dispersion and polar free energy of the testing liquids, respectively; and γDS and γPS are the dispersion and polar free energy of the testing surfaces, respectively.
A profilometer (Mitutoyo Surftest SJ-301) with a standard stylus device was used to determine the average surface roughness (Ra), which is the average distance from the profile to the mean line over the length of assessment of the fabricated polymer nanocomposites. Measurements were performed using a stylus tip with a 4 μm diameter, 10 mm min−1 tracing speed, and 90° tip angle of the tool and repeated at random points several times during the tests.
2.6. Biodegradability and field exposure testing
Different bacterial strains, including S. aureus, Bacillus subtilis, Pseudomonas aeruginosa, and E. coli, were obtained from the Faculty of Agriculture in Mircen, Cairo, Egypt. The strains were grown in nutrient broth for the maintenance and cultivation of the bacterial strains at 30 °C for 30 days.38 Biodegradation tests were carried out in 100 mL batch flasks (containing 30 mL of basal salts medium and at a pH of 7).39 The incubation was performed in a (150 rpm) shaking incubator. Then the specimens were taken away, subject to distilled water washing and then dried. Biodegradability was evaluated under aerobic conditions through weight loss determination.39
The exposure of painted panels to natural conditions is the oldest and one of the most effective techniques to study AF marine paints.40 A field trial was applied at the Suez Canal Marine shipyard, Suez, Egypt for the as-synthesized PDMS/Ag nanocomposites. To ensure the visual assessments, the FR evaluation in the field test was conducted based on a screening process and image analysis for 12 months in Red Sea water.40a,b The Suez Canal was selected as the test field because it is a tropical area where fouling occurs throughout the year. The painted panels were tested in water at temperatures of 23 °C to 30 °C, pH of 8.5–9.1, and salinity of 40%. The test steel panels (300 mm × 200 mm × 1.5 mm) were cleaned and removed of rust. The panels were then painted on two sides with anticorrosive coating followed by a tie coat. After drying, the developed nontoxic FR nanocomposite coatings were applied on the two sides of the panels. The panels were allowed to cure at RT, and the thickness of the top coat dry film was 100–150 μm.
2.7. Mechanical tests of the FR model design
The viscoelastic behavior of the designed spherical Ag/silicone nanocomposites was studied. The rectangular samples were investigated in tension mode using a TTDMA instrument (UK) at RT and 1 Hz with a 2 N preload through the ASTM D412 protocol.
Three different tests, namely, cross cut, T-bending, and impact, were performed to evaluate the mechanical properties of the prepared silicone/Ag nanocomposite coatings. The tests determined the adhesion strength, flexibility, and elasticity of the painted films.
A 170 mm × 90 m × 1 mm steel panel was degreased and coated with a primer coat of two-component epoxy resin. A tie coat of silicon/epoxy was applied as a second coat. After complete drying, a top coat of the PDMS/Ag nanocomposite coating was applied with a dry film thickness of 150 μm. The adhesion degree of the coating was examined by a cross-cut test. The coated panels were scribed with a sharp steel cutter (Sheen 750, UK), and the resulting grid size was 2 mm × 2 mm. Adhesive tape was used, and the adhesive strength was rated according to ASTM D 3359. The T-bending test was performed on the test panels (Ref 809 type model, Sheen Instruments Ltd, Kingston, UK) through ISO 6272 to check the formability of the prepared nanocomposites. The measurements were based on the spindle diameter. Impact tests were carried out using an impact tester (Ref BG5546, Sheen Instruments. Ltd, UK) according to ISO 6272 to determine the height of sudden falling load (1 kg weight) on the painted PDMS and resistance to damage of the nanocomposite films.
3. Results and discussion
3.1. Controlled particle and distribution sizes, morphology, and stability of the Ag NPs
A facile and inexpensive synthesis of spherical Ag NPs was successfully conducted, and the size of the particles could be controlled at low temperatures within short reaction times. The stability and particle size control of the Ag NPs are strongly dependent on the pH of the prepared solution.41 Increasing the molar ratio of the Ag source may inevitably result in small particle sizes and a narrow particle size distribution.42 Considerable changes in particle and distribution sizes, morphology, and stability may occur during the synthesis of Ag NPs even with minimal variations in the reaction parameters.
AF activity can be maximized by increasing the number of particles per unit area at the nanoscale level. The mechanism of Ag NP formation is discussed and illustrated in Scheme 1. The crystalline information and morphological homogeneity of the as-synthesized Ag NPs were obtained using XRD (Fig. 1). The distinct peaks at the 2θ values of 38.18, 44.34, and 64.54 correspond to the {1 1 1}, {2 0 0}, and {2 2 0} inter-planar reflections of the spherical crystal system; this finding showed that the resulting Ag NPs were well crystallized. The {111} lattice plane is more intense because of its predominant orientation compared to the other peaks. The average crystallite size (D) of <10 nm was determined by substituting the parameters in the Debye–Scherrer equation.41,43
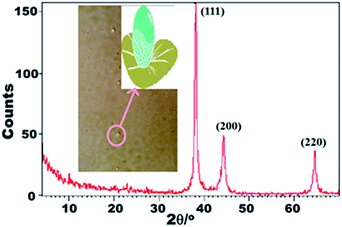 |
| Fig. 1 XRD pattern of the prepared Ag NP spheres. Inset is the schematic shape of a liquid droplet with super-hydrophobic character on the surface of coated steel panels. | |
The TEM images of the as-synthesized Ag NPs are presented in Fig. 2A–C. Overall, the prepared NPs presented a mean particle size of <10 nm, a spherical shape, and single crystals without agglomeration. The crystalline nature of the prepared Ag NPs was further confirmed via selected-area (electron) diffraction (SA-ED) (Fig. 2D). Distinct ring patterns were observed, and the {111}, {200}, and {220} crystal planes were indexed to confirm the polycrystalline nature of the prepared NPs.41 The findings indicated that the main facet is the {111} crystal plane, which represents the desired FR properties, such as low surface energy, antibacterial activity, and nearest neighbour atoms per unit area. Fig. 2E shows the fringes of the {111} lattice of the Ag NPs with 0.23 nm d-spacing. The reported values are compatible with the XRD results. Fig. 2F illustrates the elemental map of the synthesized NPs via the EDS spectrum. The results reveal that the prepared NPs demonstrated clear Ag peaks without impurities or contaminations. The peaks of Ag NPs around 3.0 refer to the Ag Lω binding energies, whereas the peaks around 3.2 and 3.4 keV belong to that of Ag Lβ and Ag Lβ2 energy, respectively. The other peaks of C and Cu elements could be attributed to the use of the carbon-coated copper grid in the analysis. The SEM images of Ag NPs (ESI, Fig. S1A and B†) reflect the well-dispersed Ag nanospheres with a smooth surface nature, which explains the good surface properties of these particles.
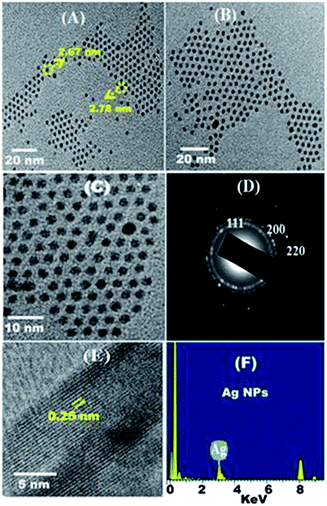 |
| Fig. 2 (A)–(C) are the TEM images of the synthesized spherical Ag NPs at different magnifications; (D) corresponding SA-ED patterns of the as-synthesized Ag NPs; (E) corresponding crystal lattice, which is consistent with the XRD results; and (F) corresponding EDX image of the as-synthesized Ag NPs. | |
3.2. Ag–polymer nanocomposite film formation and NP diffusion
With the promising features of PDMS in FR coatings16 and the advantages of applying the hydrosilation curing mechanism,32 VTPDMS was prepared successfully,16 and tailored toward developing novel solutions for non-stick FR coatings to deter fouling.
The spherical Ag NP film showed enhanced surface hydrophobicity and self-cleaning properties and exerted biological activity. The low surface energy (111) orientation of the spherical Ag NPs stabilized and enhanced the biological activity (see Section 3.4).8 Ag ions are particularly suitable for AF because of their oligodynamic effect at relatively low concentrations. The interactions of the microorganisms with the Ag NPs were considerably affected by the morphology and size distribution of the NPs.44 With this approach, novel PDMS/spherical Ag nanocomposites were fabricated (Scheme 2) and various concentrations of Ag nanospheres were embedded into the prepared VTPDMS matrix to investigate chemical inertness against fouling.
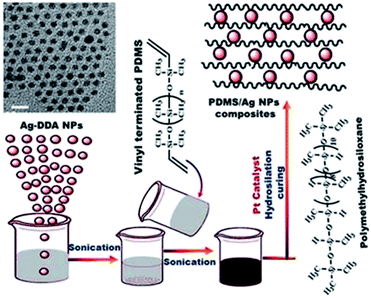 |
| Scheme 2 Preparation of a series PDMS/Ag nanocomposites with the addition of different concentrations of Ag NPs to vinyl terminal groups (VTPDMS) under sonication. | |
TEM observations showed that the Ag NPs appeared as dark spheres, and the uniformly bright surroundings were the cured PDMS. The TEM images (ESI, Fig. S1C–E†) of the prepared spherical Ag/silicone nanocomposites (0.1% Ag nanospheres concentration) showed well-dispersed spherical Ag NPs, which were separated from one another without any agglomeration at low concentrations. The retention of well-dispersed Ag NPs in homogeneous and spherical size domains without aggregation inside the PDMS matrices could significantly enhance the physical, surface and AF properties of the nanocomposites. By contrast, a different trend was observed at high concentrations (5% Ag nanosphere concentration); this finding demonstrated the high-degree of agglomeration and aggregation of the Ag NPs, as well as their condensation over one other (ESI, Fig. S1F–H†). Agglomeration and aggregation enhanced the surface roughness and reduced the surface hydrophobic properties, thereby facilitating the adhesion of fouling organisms.
3.3. Wetting behaviour and surface investigation of the fabricated nanocomposites
The main factor for the anti-adhesive behaviour of the silicone FR surfaces is the low surface tension. Fouling could be deterred by inhibiting the bonding ability on the FR surfaces and eliminated by hydrodynamic or mechanical methods. Surface hydrophobicity and free energy are the most important factors for designing FR coatings. The surface hydrophobicity of the hybrid nanocomposites was evaluated in the dry state and after submersion using static contact angle measurements, as illustrated in Fig. 3a. The unfilled PDMS showed a hydrophobic characteristic with a measured contact angle of 102° ± 1°. At various Ag nanofiller concentrations, the wetting disposal of the prepared Ag/silicone nanocomposites shifted toward higher hydrophobicity up to 0.1% Ag and presented a contact angle of 148° ± 1° in water. This finding indicated the superhydrophobic surface nature (Lotus effect) and could be attributed to the well-dispersed NPs that increased the surface area and smoothness and enhanced the chemical bonding between the NPs and the polymer matrix (Fig. 3a). The average surface roughness (Ra) measurements indicated higher microroughness for the unfilled PDMS (0.76 μm) than for the spherical Ag-filled PDMS nanocomposites (Fig. 3a). The microroughness value gradually decreased up to the Ag nanofiller (0.16 μm) concentration of 0.1%, resulting in an ultra-smooth surface with non-stick FR characteristics. As the concentration of Ag NPs increased in the matrix, hydrophobicity reduced until 5% nanofiller concentration because of the enhanced agglomeration and particle clustering of the Ag nanofillers. Consequently, the chemical bonding of the Ag nanospheres with the polymers was minimized, which is the main problem at high filling concentrations. The combination of the Ag NPs, the formation of strongly bonded aggregates, and the loss of high surface area could reduce the hydrophobic characteristics and enhance the surface roughness (0.96 μm), thereby reducing the FR efficiency. After submersion, the fabricated samples presented reduced contact angles compared with the dry samples. The hydrophobic values were restored afterward, thereby confirming the renewable properties of the designed PDMS/Ag nanocomposites.
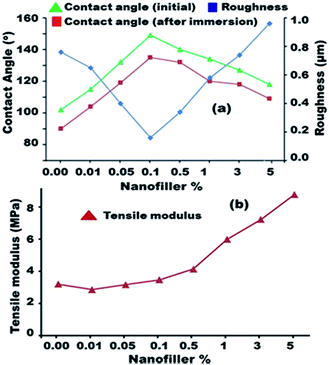 |
| Fig. 3 (a) Water contact angle measurements of the prepared unfilled and Ag-filled silicone nanocomposites before and after immersion (error bars represent ±1 standard deviation based on three determinations) and inside the roughness measurements (error bars represent ±0.01 standard deviation from three replications); (b) tensile modulus of the prepared Ag nanocomposites. | |
The surface tension values for the unfilled PDMS and Ag-filled silicone nanocomposites were calculated while dry and after submersion in water using the Zisman and OWRK equations as reported in Table 1. The data showed that γtotalS decreased at lower nanofiller concentrations (21.34 mN m−1 for unfilled PDMS) up to 0.1% Ag nanofiller, which exhibited the lowest surface free energy (10.93 mN m−1). By contrast, γtotalS gradually increased with increasing nanofiller loading up to 5% (17.49) because of the roughness caused by agglomeration and aggregation. The surface free energy of the dry samples with unfilled and filled PDMS showed lower surface free energy compared with those of the immersed PDMS and its nanocomposites. Our finding indicates that the low surface free energy was caused by the embedding of Ag in the nanocomposites (up to 0.1%). In addition, the well-dispersion of the Ag NPs is the key component factor for the inertness of the surface toward biological attachment. The chemical interaction between the polymer and the spherical Ag NPs was further analyzed by evaluating the bulk mechanical properties of the as-prepared unfilled and filled spherical Ag/silicone nanocomposites (Fig. 3b). The tensile modulus values presented a nearly steady state at lower concentrations because of the good dispersion and gradually suffered from increased stiffness with higher filling because of Ag NP agglomeration and clustering. The size of the prepared Ag NPs of < 10 nm is a major factor for the increased surface area and {111} lattice plane, resulting in pronounced surface properties. However, the surface area was reduced because of agglomeration and the {111} faces were reduced because of clustering.
Table 1 The measured dry and wet contact angles in diiodomethane (error bars represent the standard deviation from three measurements) and also the values of γtotalS calculated according to goniometer mean methods for the fabricated unfilled and filled silicone/Ag NPs (error bars represent the standard deviation from three replications)
Sample design |
(θ) Diiodomethane |
Goniometer mean method |
Dry |
Wet |
γDS |
γPS |
γtotalS (mN m−1) |
Dry |
Wet |
Dry |
Wet |
Dry |
Wet |
PDMS blank |
75° ± 1° |
70° ± 2° |
20.13 |
21.21 |
1.22 |
1.642 |
21.34 |
23.91 |
PDMS/Ag (0.01%) |
83° ± 2° |
78° ± 3° |
15.98 |
18.53 |
0.11 |
1.101 |
16.09 |
19.63 |
PDMS/Ag (0.05%) |
91° ± 2° |
84° ± 2° |
12.26 |
13.6 |
0.36 |
0.525 |
12.62 |
14.13 |
PDMS/Ag (0.1%) |
98° ± 1° |
92° ± 2° |
9.41 |
11.83 |
1.52 |
0.57 |
10.93 |
12.4 |
PDMS/Ag (0.5%) |
93° ± 1° |
88° ± 1° |
11.41 |
13.6 |
1.03 |
0.525 |
12.45 |
14.13 |
PDMS/Ag (1%) |
89° ± 2° |
83° ± 2° |
13.15 |
15.98 |
0.66 |
0.004 |
13.81 |
15.98 |
PDMS/Ag (3%) |
85° ± 3° |
78° ± 2° |
15.01 |
18.53 |
5.29 |
0.001 |
20.29 |
18.54 |
PDMS/Ag (5%) |
80° ± 1° |
73° ± 2° |
17.49 |
21.21 |
0.03 |
0.39 |
17.49 |
21.6 |
3.4. Characteristics of the tailored FR coating system based on spherical Ag NPs
We developed a novel model of a FR coating for ship hulls using spherical Ag NPs. The model enhanced the surface characteristics of silicone FR coatings by increasing the hydrophobicity and decreasing the roughness and surface free energy, thereby preventing the adhesion of fouling microorganisms through a physical non-toxic mechanism (see Fig. 4).
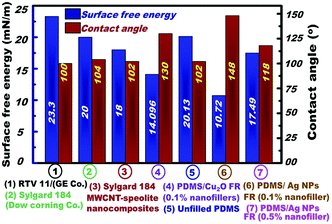 |
| Fig. 4 Comparison of the prepared PDMS/Ag nanocomposites and other commercial FR coatings. | |
To explore the real applicability of the PDMS/Ag NP nanofiller model design, the FR performance of the newly fabricated PDMS/spherical Ag nanocomposites (0.1% loading because of its superior properties) in terms of surface free energy and surface hydrophobicity was compared with the following models; (1) two commercial FR silicone coatings, namely, Sylgard® 184 (hydrosilation cured PDMS) and RTV11 (condensation cured PDMS),45 (2) Sylgard 184/multi-wall carbon nanotubes (MWCNT),46 and (3) Cu2O silicone nanocomposites (0.1% of cubic Cu2O), repectively.16 These model compositions were proven as effective FR coatings against certain bacterial growth and fouling settlements.47,48 Sylgard® 184 and RTV11 FR coatings were evaluated and compared based on surface hydrophobicity and surface free energy, as reported previously (see ESI†).45–48
Fig. 4 shows evidence that the silicone/spherical Ag nanocomposites fabricated using the preferred concentration (0.1% Ag NPs) achieved the highest surface hydrophobicity, microroughness and surface free energy, among the four FR models. This finding indicates that the designed silicone/spherical Ag nanocomposites exhibited superior FR properties and are promising as environment-friendly materials.
Our finding might indicate that the low surface energy of the silicone/spherical Ag nanofiller design is mainly associated with the existence of a high-density of {111} facets along the spherical, face-centered-cubic (fcc) Ag nanocrystal domains, which are different to other fcc metal NPs predominantly associated along low-density {110} or {100} facets, as previously reported.49a The Ag NPs orientation around {111} facets enabled a minimal interfacial energy surface that effectively affected the selective surface exposure properties and chemical activity of the nanofiller coatings, leading to pronounced FR efficiency.49
The developed nanofiller model also showed a profound effect compared with our previous model of a Cu2O based system.16a The contact angle increased up to 148° ± 1°, indicating self-cleaning ability, whereas the surface free energy decreased (approximately 10.73 mJ m−2) and became highly resistant to fouling. The biological and field test results of the prepared PDMS/Ag nanocomposites confirmed their extraordinary properties to retard fouling and prevent adhesion through a physical adhesion failure mechanism without causing toxicity. The promising AF adhesion and settlement results of the designed FR nanocomposites indicate that they are an excellent ecofriendly alternative to the existing AF systems.
3.5. Biological activity of the FR coating system based on spherical Ag NPs
PDMS composites were believed to resist decomposition by bacteria and living organisms. However, in the 20th century, a study confirmed that biodegradation can be induced by living organisms.50 PDMS biodegradation yields dimethylsilanediol and, later, carbon dioxide and inorganic silicate.51 Nevertheless, siloxane biodegradation remains a challenge because of the lack of decomposition data available. Biodegradation studies were conducted on the prepared spherical Ag/silicone nanocomposites by applying biodegradability tests for 30 days of immersion with selected microorganisms. With various concentrations starting from 0.01% up to higher loadings, the biodegradability results were compared as shown in Fig. 5. The biodegradability percentages were higher in the unfilled PDMS and decreased gradually until it reached nearly zero for the 0.1% loading in the PDMS/Ag nanocomposites. This finding could be due to the good dispersion of the Ag NPs without any agglomeration, which improved the surface hydrophobicity, surface smoothing, and chemical bonding with the polymer chains (as indicated by the Ra measurements in Fig. 3a). As a result, the composites exhibited extraordinary FR characteristics of resistance to adhesion of fouling organisms and prevention of biofouling. By contrast, with increasing Ag nanofiller loading from 0.5% to 5%, biodegradability gradually increased after 30 days of immersion. This finding could be due to agglomeration and aggregation at higher Ag nanosphere concentrations.
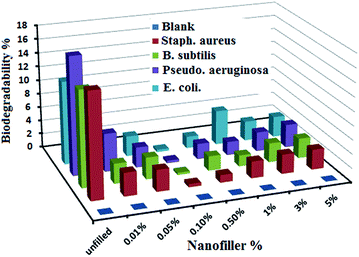 |
| Fig. 5 Biodegradability measurements of the unfilled and filled PDMS/Ag nanocomposites against various bacterial strains. | |
Consequently, roughness increased and chemical bonding with the polymer and hydrophobicity decreased to a lesser extent because of the decreased surface area of the clustered particles, thereby facilitating the ability of microorganisms to attach to the surface. These results were confirmed by film coverage pictures taken using a polarized optical microscope (ESI, Fig. S2†).
A field exposure test was applied on the prepared PDMS/spherical Ag nanocomposites (0.1% nanofiller concentration due to its superior FR properties), as illustrated in Fig. 6. In these experimental studies, we used unfilled PDMS as the control (ESI, Fig. S3†). Fouling prevention against micro and macro-fouling organisms occurred until the 12th month of immersion. No adherence of fouling organisms and surface deterioration were observed. In the formulation, after 12 months of immersion, the few adhered microorganisms on the edges were easily removed hydrodynamically because of the superior FR performance of the novel PDMS/Ag nanocomposite with renewable self-cleaning properties.
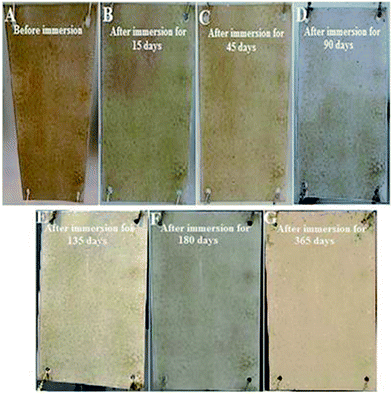 |
| Fig. 6 (A)–(G) are the field exposure test results of the prepared Ag (0.1%)/PDMS nanocomposites for 12 months of immersion in natural sea water. | |
3.6. Mechanical feature tests
The cross cut test was performed without resulting visible adhesion defects (ESI, Table S1†). The T-bend test was conducted without visible cracking. No intrusion was identified under a magnifying glass in any of the investigated coatings after penetration and bending on a <5 mm cylindrical spindle (ESI, Table S1†). No cracks were observed during the impact test, indicating the high elasticity and flexibility of the tested PDMS nanocomposites and the total formulation.
3.7. AF coating formulation for FR coating
The incorporation of pigments in marine coatings affects various physical properties. Studies performed on pigmented FR coatings revealed that the adhesion strength of the coating increased with increasing pigment level up to 10 wt%.52 This finding could be due to the increase in coating cohesive strength and subsequent increase in tensile strength and modulus caused by improved mechanical properties from surface homogeneity. Increasing pigment levels reduces the adhesion strength of the paint to the metallic surface because of pigment aggregation and agglomeration at high percentages.53 Red iron oxide was selected because of its low toxicity, cheap cost, and anticorrosive properties.53 The pigment surfactant was well-dispersed. A field test of the total formulation was further conducted in natural environments against various microorganisms. The results showed superior fouling resistance and prevention against macro-fouling of organism settlements (Fig. 7). The rust around the edges was not found until 12 months.
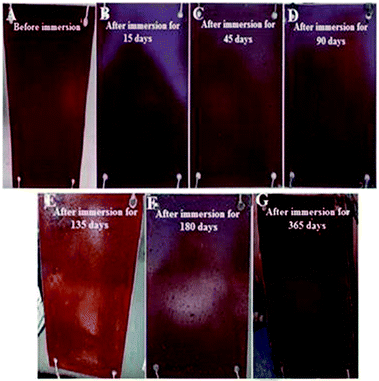 |
| Fig. 7 (A)–(G) Field test results of the formulated coating for 12 months of immersion in natural seawater. | |
4. Conclusions
This study demonstrates a simple, safe, cost-effective, and eco-friendly preparation of spherical Ag NPs. To our knowledge, this study is the first to utilize Ag NPs in silicone FR coatings. This study also elucidated the underlying mechanism, which is based on developing a superhydrophobic surface that prevents fouling from adhesion without leaching. The characterization results of the prepared Ag NPs revealed a homogenous spherical shape enclosed by {111} facets, particle sizes of less than 10 nm, and well-distributed NPs without agglomeration. For the shipping industry, this study provides insights regarding the capability of superhydrophobic PDMS/Ag nanocomposite coatings to inhibit biofouling through their inertness and low surface free energy. Advanced substrates were designed based on micro-nano fabricated PDMS/Ag hybrid nanocomposites with various nanofiller loading concentrations. The surface properties relevant to the developed Ag nanocomposites were reported on the basis of contact angle determinations and OWRK equations. The results demonstrated that a significant increase was observed at 0.1% loading, which exhibited the lotus effect, self-cleaning, and FR property. By contrast, high loadings showed severe deviation toward a lesser angle of contact, increased surface coarseness, nonhomogeneity, and rough topology because of agglomeration, thereby minimizing the FR ability. Interestingly, no significant effect on the viscoelastic properties was observed at low concentrations of Ag NPs up to 0.1%. By using a biological assay in the laboratory and field exposure to test seawater environments, the FR performance of the prepared nanocomposites was studied.
The designed PDMS/Ag nanocomposite surfaces could achieve economical savings, considering the annual costs sustained for controlling biofouling impact. The advantages of the developed composite could contribute to prolonging longevity and establishing strategies and investments in green technologies. This work strongly highlights that PDMS/Ag is a promising environment-friendly AF coating and exhibits high potential for applications in FR silicon/noble metal nanocomposite technology.
Notes and references
-
(a) J. Curtis, M. Greenberg, J. Kester, S. Phillips and G. Krieger, Toxicol. Rev., 2006, 25, 245–260 CrossRef CAS
;
(b) M. Khairy, S. A. El Safty, M. A. Shenashen and E. A. Elshehy, Nanoscale, 2013, 5(17), 7920–7927 RSC
;
(c) S. A. El-Safty, S. Abdellatef, M. Ismael and A. Shahat, Adv. Healthcare Mater., 2013, 2(6), 854–862 CrossRef CAS PubMed
;
(d) M. Khairy, S. A. El Safty and M. Ismael, Chem. Commun., 2012, 48(88), 10832–10834 RSC
;
(e) M. Khairy and S. A. EL-Safty, RSC Adv., 2013, 3, 23801–23809 RSC
;
(f) M. Khairy and S. A. EL-Safty, Chem. Commun., 2014, 50, 1356–1358 RSC
;
(g) M. Khairy and S. A. El-Safty, Sens. Actuators, B, 2014, 193, 644–652 CrossRef CAS PubMed
. -
(a) J.-P. Kaiser, S. Zuin and P. Wick, Sci. Total Environ., 2013, 442, 282–289 CrossRef CAS PubMed
;
(b) T. C. Thomas and R. A. Narvaez, J. Commerc. Biotechnol., 2006, 12, 105–110 CrossRef
. -
(a) S. A. El-Safty, J. Colloid Interface Sci., 2003, 260, 184–194 CrossRef CAS
;
(b) S. A. El-Safty, M. A. Shenashen and M. Khairy, Talanta, 2012, 98, 69–78 CrossRef CAS PubMed
;
(c) S. A. El-Safty, A. A. Ismail, H. Matsunaga, H. Nanjo and F. Mizukami, J. Phys. Chem. C, 2008, 112, 4825–4834 CrossRef CAS
;
(d) E. A. Elshehy, S. A. EL-Safty, M. A. Shenashen and M. Khairy, Sens. Actuators, B, 2014, 203, 363–374 CrossRef CAS PubMed
. -
(a) R. Handy, R. Owen and E. Valsami-Jones, Ecotoxicology, 2008, 17, 315–325 CrossRef CAS PubMed
;
(b) M. Khairy, S. A. El-Safty and M. A. Shenashen, Trends Anal. Chem., 2014, 62, 56–68 CrossRef CAS PubMed
;
(c) S. A. El-Safty, M. Sakai, M. Selim and A. A. Alhamid, Chem.–Asian J., 2015 DOI:10.1002/asia.201500421
. - R. L. Townsin, Biofouling, 2003, 19, 9–15 CrossRef PubMed
. - E. Korkut and M. Atlar, Ocean Eng., 2012, 41, 1–12 CrossRef PubMed
. -
(a) J. Genzer and K. Efimenko, Biofouling, 2006, 22(5), 339–360 CrossRef CAS PubMed
;
(b) L. Xue, X. Lu, H. Wei, P. Long, J. Xu and Y. Zheng, J. Colloid Interface Sci., 2014, 421, 178–183 CrossRef CAS PubMed
;
(c) N. D. Hoa and S. A. El-Safty, Nanotechnology, 2011, 22, 485503 CrossRef PubMed
;
(d) N. D. Hoa and S. A. El-Safty, J. Phys. Chem. C, 2011, 115(17), 8466–8474 CrossRef
. -
(a) N. D. Hoa and S. A. El-Safty, Chem.–Eur. J., 2011, 17, 1286–1290 CrossRef PubMed
;
(b) N. D. Hoa, Anal. Methods, 2011, 3, 1948–1956 RSC
;
(c) S. A. El-Safty, N. D. Hoa and M. A. Shenashen, Eur. J. Inorg. Chem., 2012, 5439–5450 CrossRef CAS PubMed
. -
(a) V. A. Ganesh, A. Baji and S. Ramakrishna, RSC Adv., 2014, 4, 53352–53364 RSC
;
(b) M. V. Laitano, J. D. Nuñez and M. Cledón, Ecol. Indic., 2013, 34, 345–351 CrossRef CAS PubMed
. -
(a) IMO, International Convention on the Control of Harmful Anti-Fouling Substances on Ships, AFS/CONF/26, 2001;
(b) C. Bressy, A. Margaillan, F. Faÿ, I. Linossier and K. Rehel, in Advances in Marine Antifouling Coatings and Technologies, ed. C. Hellio and D. M. Yebra, Woodhead Publishing, Cambridge, UK, 2009, pp. 445–491 Search PubMed
. - S. B. Sjollema, G. M. García, H. G. van der Geest, M. H. S. Kraak and P. Booij, Environ. Pollut., 2014, 187, 106–111 CrossRef CAS PubMed
. - K. V. Thomas and S. Brooks, Biofouling, 2010, 26, 73–88 CrossRef CAS PubMed
. - R. F. Brady, J. Coat. Technol., 2000, 72, 45–56 CrossRef
. - M. Nendza, Mar. Pollut. Bull., 2007, 54, 1190–1196 CrossRef CAS PubMed
. -
(a) S. Krishman, C. J. Welnman and C. K. Ober, J. Mater. Chem., 2008, 18, 3405–3413 RSC
;
(b) M. S. Rahaman, H. Thérien-Aubin, M. Ben-Sasson, C. K. Ober, M. Nielsen and M. Elimelech, J. Mater. Chem. B, 2014, 2, 1724–1732 RSC
. -
(a) M. S. Selim, S. A. El-Safty, M. A. El-Sockary, A. I. Hashem, O. M. Abo Elenien, A. M. EL-Saeed and N. A. Fatthallah, RSC Adv., 2015, 5(26), 19933–19943 RSC
;
(b) X. Liu, W. Tong, Z. Wu and W. Jiang, RSC Adv., 2013, 3, 4716–4722 RSC
. -
(a) M. Lejars, A. Margaillan and C. Bressy, Chem. Rev., 2012, 112, 4347–4390 CrossRef CAS PubMed
;
(b) R. F. Brady, J. Prot. Coat. Linings, 2000, 17, 42–46 Search PubMed
;
(c) M. Lejars, A. Margaillan and C. Bressy, Polym. Chem., 2013, 4, 3282–3292 RSC
. -
(a) T. Balaji, S. A. El-Safty, H. Matsunaga, T. Hanaoka and F. Mizukami, Angew. Chem., Int. Ed., 2006, 45, 7202–7208 CrossRef CAS PubMed
;
(b) S. A. El-Safty, A. Shahat, W. Warkocki and M. Ohnuma, Small, 2011, 7, 62–65 CrossRef CAS PubMed
;
(c) S. A. El-Safty and M. A. Shenashen, Trends Anal. Chem., 2012, 48, 98–115 CrossRef PubMed
;
(d) M. A. Shenashen, S. A. EI-Safty, E. A. Elshehy and M. Khairy, Eur. J. Inorg. Chem., 2015,(1), 179–191 CrossRef CAS PubMed
. -
(a) X. Y. Xu, Q. B. Yang, J. Bai, T. C. Lu, Y. X. Li and X. B. Jing, J. Nanosci. Nanotechnol., 2008, 8, 5066–5070 CrossRef CAS PubMed
;
(b) M. A. Shenashen, S. A. El-Safty and E. A. Elshehy, Particle, 2014, 31(3), 293–316 CAS
. - J. R. Morones, J. I. Elechiguerra, A. Camacho, K. Holt, J. B. Kouri, J. T. Ramirez and M. J. Yacaman, Nanotechnology, 2005, 16, 2346–2353 CrossRef CAS PubMed
. - J. Liu and R. H. Hurt, Environ. Sci. Technol., 2010, 44, 2169–2175 CrossRef CAS PubMed
. - K. J. Kim, W. S. Sung, B. K. Suh, S. K. Moon, J. S. Choi, J. G. Kim and D. G. Lee, BioMetals, 2009, 22, 235–242 CrossRef CAS PubMed
. - J. C. Trefry, PhD thesis Biomedical Sciences, Wright State University, 2011
. - S. Gurunathan, K. J. Lee, K. Kalishwaralal, S. Sheikpranbabu, R. Vaidyanathan and S. H. Eom, BioMetals, 2009, 30, 6341–6350 CAS
. -
(a) T. Szabó, J. Mihály, I. Sajó, J. Telegdi and L. Nyikos, Prog. Org. Coat., 2014, 77, 1226–1232 CrossRef PubMed
;
(b) M. A. Shenashen, S. A. El-Safty and E. A. Elshehy, Potential role of silver nanoparticles morphology on its applications, in Silver nanoparticles: synthesis, uses and health concerns, ed. I. Armentano and J.M. Kenny, Nova Science Publishers, Inc., N.Y., 2013, ch. 6, pp. 131–165 Search PubMed
. - K. K. Hoskote and B. K. Mandal, Spectrochim. Acta, Part A, 2015, 135, 639–645 CrossRef PubMed
. - A. A. Ashkarran, S. Estakhri, M. R. H. Nezhad and S. Eshghi, Phys. Procedia, 2013, 40, 76–83 CrossRef CAS PubMed
. - B. Sun, X. Jiang, S. Dai and Z. Du, Mater. Lett., 2009, 63, 2570–2573 CrossRef CAS PubMed
. - A. Regiel and K. Y. Agnieszk, Chemik, 2013, 67(8), 683–692 CAS
. - A. N. Vasiliev, E. A. Gulliver, J. G. Khinast and R. E. Riman, Surf. Coat. Technol., 2009, 203, 2841–2844 CrossRef CAS PubMed
. - F. Gentile, M. L. Coluccio, A. Accardo, G. Marinaro, E. Rondanina, S. Santoriello, S. Marras, G. Das, L. Tirinato, G. Perozziello, F. de Angelis, C. Dorigoni, P. Candeloro and E. Di Fabrizio, Microelectron. Eng., 2012, 97, 349–352 CrossRef CAS PubMed
. - K. M. M. Abou El-Nour, A. Eftaiha, A. Al-Warthan and R. A. A. Ammar, Arabian J. Chem., 2010, 3, 135–140 CrossRef CAS PubMed
. -
(a) J. Bai, Y. Li, J. Du, S. Wang, J. Zheng, Q. Yang and X. Chen, Mater. Chem. Phys., 2007, 106, 412 CrossRef CAS PubMed
;
(b) M. A. Shenashen, S. A. El-Safty and E. A. Elshehy, Synthesis methodology for size and shape control of silver nanoparticles, in Silver nanoparticles: synthesis, uses and health concerns, ed. I. Armentano and J. M. Kenny, Nova Science Publishers, Inc., N.Y., 2013, ch. 5, pp. 101–128 Search PubMed
. - Y. Li, Y. Wu and B. S. Ong, J. Am. Chem. Soc., 2005, 127, 3266–3267 CrossRef CAS PubMed
. - M. Oliveira, D. Ugarte, D. Zanchet and A. Zarbin, J. Colloid Interface Sci., 2005, 292, 429 CrossRef CAS PubMed
. - A. J. Chalk and J. F. Harrod, J. Am. Chem. Soc., 1965, 87, 16–21 CrossRef CAS
. -
(a) S. Wu, Polymer Interface and Adhesion, Marcel Dekker, New York, 1982 Search PubMed
;
(b) S. K. Rath, J. G. Chavan, S. Sasane, S. Jagannath, M. Patri, A. B. Samui and B. C. Chakraborty, Appl. Surf. Sci., 2010, 256, 2440–2446 CrossRef CAS PubMed
. - R. M. Atlas, Handbook of microbiological media, CRC Press LLC, New York, 3rd edn, 2004 Search PubMed
. -
(a) G. F. Moore and S. M. Saunders, Advances in biodegradable polymers, Smithers Rapra Publishing, 1998 Search PubMed
;
(b) E. M. Sadek, S. L. Abd-El-Messieh, A. A. Khalil, N. A. Fatthallah, A. I. A. Eid, K. M. El-Ashry and A. M. Motawie, IOSR J. Appl. Chem., 2014, 7(11), 37–45 CrossRef
;
(c) C. Howell, T. L. Vu, J. J. Lin, S. Kolle, N. Juthani, E. Watson, J. C. Weaver, J. Alvarenga and J. Aizenberg, ACS Appl. Mater. Interfaces, 2014, 6(15), 13299–13307 CrossRef CAS PubMed
. -
(a) G. W. Swain, J. R. Griffith, J. D. Bultman and H. L. Viencent, Biofouling, 1992, 6, 105–114 CrossRef CAS PubMed
;
(b) C. S. Lim, S. C. Lee, W. Leong, Y. X. Ng and S. L. M. Teo, Indian J. Geo-Mar. Sci., 2014, 43(11), 67–75 Search PubMed
. -
(a) J. I. Hussain, S. Kumar, A. A. Hashmi and Z. Khan, Adv. Mater. Lett., 2011, 2(3), 188–194 CrossRef CAS PubMed
;
(b) M. Mekawy, A. Yamaguchi, S. A. El-Safty, T. Itoh and T. Teramae, J. Colloid Interface Sci., 2011, 355, 348–358 CrossRef CAS PubMed
;
(c) S. A. El-Safty, M. Mekawy, A. Yamaguchi, A. Shahat, K. Ogawa and N. Teramae, Chem. Commun., 2010, 46, 3917–3919 RSC
. - Y. Zhang, H. Peng, W. Huang, Y. Zhou and D. Yan, J. Colloid Interface Sci., 2008, 325, 371–376 CrossRef CAS PubMed
. -
(a) V. Sunny, T. N. Narayanan, U. S. Sajeev, P. A. Joy, D. Sakthi Kumar and Y. Yoshida, Nanotechnology, 2006, 17, 4765–4772 CrossRef CAS PubMed
;
(b) S. A. El-Safty, Y. Kiyozumi, T. Hanaoka and F. Muzukami, Appl. Catal., A, 2008, 337, 121–129 CrossRef CAS PubMed
;
(c) M. Khairy, S. A. El-Safty, M. Ismael and H. Kawarada, Appl. Catal., B, 2012, 123–124, 162–173 Search PubMed
;
(d) M. Khairy, S. A. El-Safty, M. Ismael and H. Kawarada, Appl. Catal., B, 2012, 127, 1–10 CrossRef CAS PubMed
;
(e) S. A. El-Safty, Y. Kiyozumi, T. Hanaoka and F. Muzukami, Appl. Catal., B, 2008, 82(3–4), 169–179 CrossRef CAS PubMed
. - B. Yu, K. M. Leung, Q. Guo, W. M. Lau and J. Yang, Nanotechnology, 2011, 22(11), 115603 CrossRef
. - K. J. Wynne, G. W. Swain, R. B. Fox, S. Bullock and J. Uilk, Biofouling, 2000, 16, 277–288 CrossRef CAS PubMed
. - A. Beigbeder, P. Degee, S. L. Conlan, R. J. Mutton, A. S. Clare, M. E. Pettitt, M. E. Callow, J. A. Callow and P. Dubois, Biofuels, 2008, 24(4), 291–302 CrossRef PubMed
. - A. A. Al-Juhni, Ph.D. thesis, The Graduate Faculty, University of Akron, 2006
. - A. A. Al-Juhni and B. Z. Newby, Prog. Org. Coat., 2006, 56, 135–145 CrossRef CAS PubMed
. -
(a) H. A. Keul, M. Möller and M. R. Bockstaller, CrystEngComm, 2011, 13, 850–856 RSC
;
(b) B. Sun, X. Jiang, S. Dai and Z. Du, Mater. Lett., 2009, 63, 2570–2573 CrossRef CAS PubMed
;
(c) S. A. El-Safty, A. Shahat, M. Mekawy, H. Nguyen, W. Warkocki and M. Ohnuma, Nanotechnology, 2010, 21, 375603 CrossRef PubMed
. - R. Grümping, K. Michalke, A. V. Hirener and R. Hensel, Appl. Environ. Microbiol., 1999, 65(5), 2276–2278 Search PubMed
. - E. F. C. Griessbach and R. G. Lehmann, Chemosphere, 1999, 38(6), 1461–1468 CrossRef CAS
. - S. M. Mirabedini, M. Mohseni, S. PazokiFard and M. Esfandeh, Colloids Surf., A, 2008, 317, 80–86 CrossRef CAS PubMed
. - W. Hole Oceanographic Institution (WHOI), Marine Fouling and its Prevention, United States Naval Institute, Annapolis, Maryland, 1952 Search PubMed
.
Footnote |
† Electronic supplementary information (ESI) available. See DOI: 10.1039/c5ra07400b |
|
This journal is © The Royal Society of Chemistry 2015 |