DOI:
10.1039/C5RA07015E
(Paper)
RSC Adv., 2015,
5, 41135-41143
Development of new graphene oxide incorporated tricomponent scaffolds with polysaccharides and hydroxyapatite and study of their osteoconductivity on MG-63 cell line for bone tissue engineering
Received
18th April 2015
, Accepted 29th April 2015
First published on 29th April 2015
Abstract
Regenerative medicine witnessed a paradigm shift from synthetic implants and tissue grafts to a tissue engineering approach that incorporates biodegradable, bioceramic composite scaffolds with biological cells. A combination of carbon nanomaterials and hydroxyapatite (HAP) with polysaccharides holds a great potential in bone tissue engineering. In the present study, new porous tricomponent scaffolds, graphene oxide (GO)–gellan–HAP, GO–alginate–HAP and GO–amylopectin–HAP were prepared by freeze drying method. The ionic interactions between the individual components in the formation of the composites were confirmed by FTIR and XRD. The porous morphology of scaffolds was confirmed by FE-SEM images. Osteoconductivity and biocompatibility of scaffolds on MG 63 cell line were confirmed by in vitro MTT assay. The increased mineralization could be visualized by alkaline phosphatase (ALP) activity. Among the scaffolds, the GO–amylopectin–HAP exhibited higher biocompatibility, mineralization and cell attachment. The compressive strength values were determined and found to be 466.8 ± 19 for GO–gellan–HAP, 171 ± 17 for GO–alginate–HAP and 161 ± 4 for GO–amylopectin–HAP scaffolds. The higher biocompatibility, mineralization and cell attachment and lower compressive strength for GO–amylopectin–HAP was attributed to higher pore size and porosity. These results indicated that the prepared tricomponent scaffolds could be used as promising biomaterials in tissue engineering.
Introduction
Tissue engineering for bone is a multifarious and dynamic process that initiates with migration and recruitment of osteoprogenitor cells followed by their proliferation, differentiation, matrix formation along with remodelling of the bone.1 Recently, different biomaterials are investigated in the field of tissue engineering to overwhelm the hurdles which are essential for it to be successful in clinical applications.2 The essential properties required for an ideal scaffold in tissue engineering include osteoinduction, osteoconductivity, mechanical strength, porosity, mineralization, biodegradability and bioresorbability. An ideal 3D scaffold facilitates cells to undergo differentiation, proliferation and the ability to transfer cells and bioactive materials to defective sites for the new tissue formation. Further, it should be stable for a specific period of time to facilitate the body to get healed or to regain the ability to get healed. Then, the scaffold should biodegrade or become non-functional. Hence, biodegradable natural polysaccharides that are similar to biological macromolecules or that which can mimic natural extracellular matrix (ECM) are chosen to develop scaffolds.3–9 Some of the biodegradable natural polysaccharides reported for tissue engineering applications are alginic acid,10 amylopetcin, chitin, chitosan, chondritine sulfate,11 gellan gum,12 hyaluronic acid13 and xanthan gum.14
HAP is an important bioceramic in bone tissue engineering because of its similarity to the inorganic minerals present in the natural bone.15,16 It is used in several biomedical applications including drug delivery,17 orthopedics, dentistry, maxillofacial,18 bone and wound tissue engineering19 due to its biocompatibility, bioactivity and osteoconductivity.20 The carbonated HAP isolated from natural sources has several advantages like higher biocompatibility and bioresorption than synthesized HAP.21,22 Hence, natural HAP isolated from chicken bones23 are used in this study. The healing rate depends on the location of bone tissue in the human body. In order to mimic the role of bone, polymer–HAP composite should possess mechanical strength, resorption and biodegradation.2
The carbon based nano fillers such as carbon black, carbon nanotubes (CNTs), expanded graphite, graphene (GP) and carbon nanofiber were used as an excellent filler material in order to improve mechanical strength. CNTs are effective filler material but its higher production cost created a need for the low cost GP as an alternative.24,25 The hydrophobic nature of GP tends to form irreversible agglomeration in aqueous solution due to the strong π–π stacking interactions. Hence, the oxidised form of GP, GO with the presence of hydroxyl, carboxyl and epoxy groups emerge as a replacement due to greater interaction with proteins through covalent, electrostatic and hydrogen bonding. Further, it also improves the interfacial interaction with hydrophilic polymers.26–28 Previous studies revealed that the incorporation of GO in scaffolds not only enhanced the mechanical properties but also activate the functions of osteoblast culture.29 Hence, GO has created much attention in biomedical applications including bioassays, biosensors, bio-imaging, drug delivery and tissue engineering.24,27,30
The preparation of tricomponent scaffolds has gained increased attention in recent times due to the reason that it can mimic the natural bone. Since the natural bone is composed of organic and inorganic materials, bioresorbable polysaccharides (gellan, alginate and amylopectin) are used as organic material and bioactive carbonated HAP as inorganic mineral in this study. Further, GO has been included as effective filler material for increasing the mechanical strength. The combination of polysaccharides with HAP and GO showed improved osteoconductivity with increased mechanical strength. Moreover, osteoconductive biomaterials act as a passive site for cellular adhesion, proliferation and differentiation.31 Ideal scaffolds should be porous in order to allow cell to in growth, migrate and permit the efficient transport of nutrients along with the removal of cellular waste product. Further, porosity can increases the biodegradation of the implant material by increasing the surface area in contact with body fluids. This may enhances osteoconductivity but decreases the mechanical strength. The mechanical strength of the scaffolds are improved by the addition of GO. Hence, freezing and lyophilisation method was used to prepare porous scaffolds which has the advantages of simplicity in procedure and minimal equipments.32–37 There are many tricomponent scaffolds like multi walled carbon nanotube (MWCNT)–chitosan–HAP,21 MWCNT–gelatin–HAP,38 GO–chitosan–HAP18 and GO–hyaluronic acid–HAP39 which have already been reported. To best of our knowledge, we report the preparation, characterization, compressive strength, in vitro osteconductivity and ALP activity of new tricomponent scaffolds of GO–alginate–HAP, GO–amylopectin–HAP and GO–gellan gum–HAP on MG-63 cell line for the first time. The hydrophilic nature possessed by the components present in the scaffolds enhances the cell adhesion and proliferation. All the tricomponent scaffolds showed better cell proliferation, differentiation and compressive strength based on their porosity.
Result and discussion
Thermal stability
The thermo gravimetric analysis of the scaffold materials was shown in Fig. 1. The first weight loss was observed between 30 °C to 180 °C in the scaffold materials due to the evaporation of absorbed water molecules.22 Further, the major weight loss between 214 °C to 580 °C in GO–gellan–HAP, 209 °C to 568 °C in GO–alginate–HAP and 186 °C to 586 °C in GO–amylopectin–HAP was attributed to the thermal decomposition of gellan, alginate and amylopectin in to carbonaceous material and combustion of GO. The negligible amount of weight loss observed above 600 °C indicated the thermal stability of HAP.18,40–42 Hence, the TG analysis provides the evidences for the presence of all the components in the prepared tricomponent scaffolds.
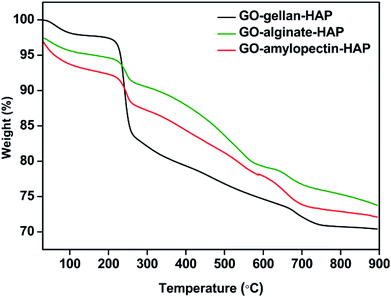 |
| Fig. 1 TGA images of tricomponent scaffolds. | |
FTIR analysis
FTIR spectra of raw materials and tricomponent scaffolds were recorded to identify the interactions between the components in scaffolds and shown in Fig. 2. All characteristic peaks for HAP 3570, 1460, 1049, 966, 881 and 634 cm−1 were observed.11,23 The appearance of peaks corresponding to OH− stretching at 3352 cm−1, C
O at 1716 cm−1, C
C vibration at 1620 cm−1, C–OH stretching at 1224 cm−1 and C–O–C stretching at 1051 cm−1 confirmed the oxidation product of graphite, the GO.43
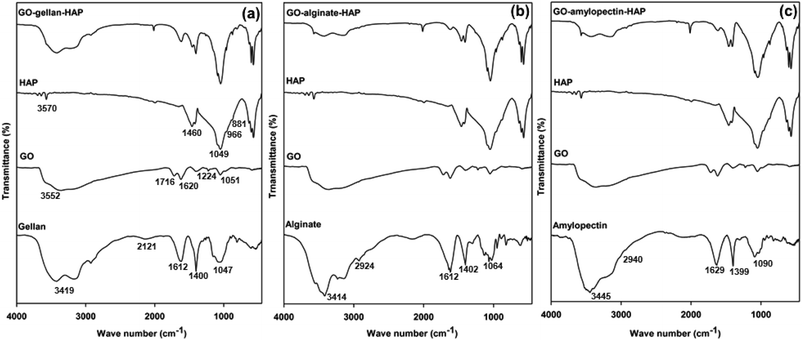 |
| Fig. 2 FTIR spectra of (a) GO–gellan–HAP, (b) GO–alginate–HAP and (c) GO–amylopectin–HAP scaffold. | |
The characteristic bands OH− stretching at 3419 cm−1, C–H stretching at 2121 cm−1, asymmetric carboxylate at 1612 cm−1, symmetric carboxylate at 1400 cm−1 and pyranoside ring at 1047 cm−1 for gellan,44,45 OH− stretching at 3414 cm−1, C–H stretching vibrations at 2924 cm−1, asymmetrical COO− stretching at 1612 cm−1, symmetrical COO− stretching at 1402 cm−1 and C–O–C stretching (saccharide structure) at 1064 cm−1 for alginate,46,47 and OH− stretching at 3445 cm−1, asymmetric C–H stretching at 2940 cm−1, adsorbed water at 1629 cm−1, angular deformation of C–H at 1399 cm−1, C–O ether stretching at 1090 cm−1 and C–O alcohol stretching at 1028 cm−1 for amylopectin11,48 confirmed the presence of gellan, alginate and amylopectin.
In the FTIR spectrum of GO–gellan–HAP, GO–alginate–HAP and GO–amylopetcin–HAP scaffolds, all the characteristic peaks of the individual components were observed with a slight shift in the peak values. The mixed characteristic peaks confirmed the dispersion of HAP and GO in the polysaccharides such as alginate, amylopectin and gellan. Further, the chemical interactions in the tricomponent scaffolds were indicated by the shift in the peaks with reduced peak intensity.11,22 Furthermore, the shift of phosphate peak in HAP (1049 cm−1) to lower wave numbers confirmed the strong intermolecular hydrogen bonding between HAP and GO.49
XRD analysis
Phase and crystallinity of the prepared tricomponent scaffolds were identified using XRD analysis and shown in Fig. 3. The diffraction pattern of isolated HAP was found to be in good conformity with the standard values of HAP (JCPDS-09-0432/1996). The disappearance of the characteristic peak of graphite at 26.4° with a d-spacing 3.37 Å and the appearance of the characteristic peak of GO at 11.9° with a d-spacing 7.43 Å confirmed the oxidation of graphite to GO. The higher d-spacing value of GO was due to the presence of oxygen containing functional groups such as carboxyl, hydroxyl, epoxy and other structural defects.43 The broad diffraction peaks obtained for gellan (19.34°), alginate (13.57° and 22.75°) and amylopectin (13.62°) indicated the amorphous nature with lower crystallinity.7 The shift of characteristic peaks of HAP, GO, gellan, alginate and amylopectin for all the composite and broadening of peaks of HAP along with peaks of reduced intensity for GO and polysaccharides in the XRD patterns of tricomponent scaffolds for GO–gellan–HAP, GO–alginate–HAP and GO–amylopectin–HAP confirmed the formation of composites and the interactions between HAP, GO and polysaccharides.11,18,21,22
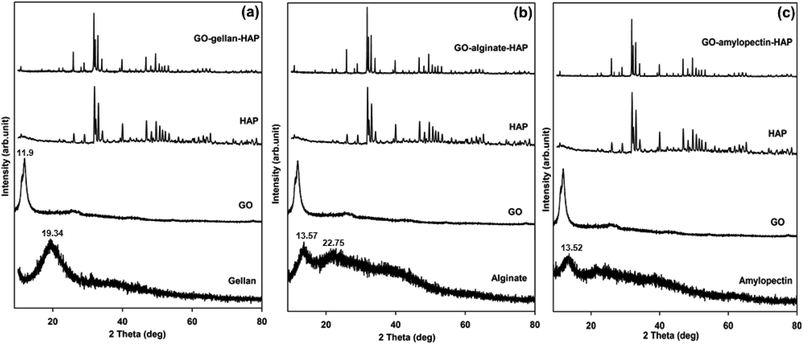 |
| Fig. 3 XRD pattern of (a) GO–gellan–HAP, (b) GO–alginate–HAP and (c) GO–amylopectin–HAP scaffold. | |
FE-SEM analysis
Morphological studies of prepared tricomponent scaffolds were analyzed by FE-SEM analysis and shown in Fig. 4. FE-SEM images of the isolated HAP (Fig. 4a) confirmed the crystalline nature with crystal size of 300–400 nm. Morphology of GO–alginate–HAP (Fig. 4c) and GO–amylopectin–HAP (Fig. 4d) confirmed the porous nature with pore size 56–66 μm and 89–101 μm respectively, whereas GO–gellan–HAP (Fig. 4b) showed low porosity with pore size 17–32 μm (Fig. 4e insert) which may be attributed to the rigidity and higher viscous nature of the gellan. The lower porosity possessed by GO–gellan–HAP scaffolds was further corroborated from the cross sectional SEM images (Fig. 5a–c) of tricomponent scaffolds. The uniform deposition of HAP on polysaccharides can be visualized and confirmed by FE-SEM images (Fig. 4b–d).
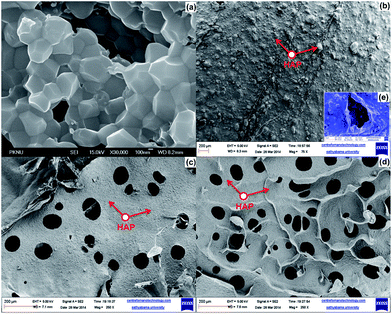 |
| Fig. 4 FE-SEM images of (a) HAP (b) GO–gellan–HAP (insert (e) higher magnification), (c) GO–alginate–HAP and (d) GO–amylopectin–HAP scaffold. | |
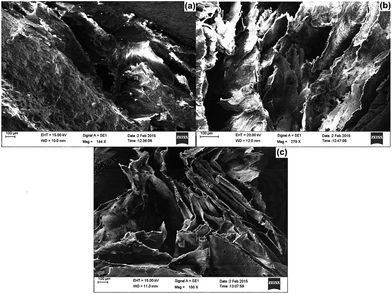 |
| Fig. 5 Cross sectional FE-SEM images of (a) GO–gellan–HAP, (b) GO–alginate–HAP and (c) GO–amylopectin–HAP scaffold. | |
Porosity analysis
Natural bone is composed of collagen–HAP composite with 10–30% porous hard outer layer and 30–90% porous interior. The higher porosity of scaffold is an important factor for new bone tissue formation which allows the migration and proliferation of osteoblasts and mesenchymal cells, as well as vascularization and delivering genes and proteins.1,11 Total porosity of the prepared tricomponent scaffolds were determined by liquid displacement methods and the values were found to be 80.76% for GO–gellan–HAP scaffolds, 90.64% for GO–alginate–HAP and 94.79% for GO–amylopectin–HAP. The higher porosity of the prepared tricomponent scaffolds met the requirements of the material for bone tissue engineering.
In vitro biocompatibility assay
Biocompatibility is a prerequisite for the use of tricomponent scaffolds in tissue engineering. The cell viability and proliferation of MG 63 cells on the prepared tricomponent scaffolds were evaluated using the MTT assay and shown in Fig. 6. When seeding 3D scaffold with cells it is desired that the cells will infiltrate and colonize the scaffold laying down their own ECM. The higher porosity (∼90%)50 and its ability to promote the cell migration and tissue growth are some of the advantages of the scaffolds prepared.51 The cell proliferation increased with increasing days as shown in Table 1 which proves their biocompatibility. The results revealed that the prepared tricomponent scaffolds does not exert any cytotoxic effect on MG 63 cell line. Recent studies proved that the cytocompatability of GO was due to the presence of oxygen containing functionalities.
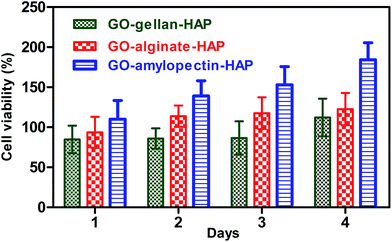 |
| Fig. 6 In vitro cytotoxicity assay of GO–gellan–HAP, GO–alginate–HAP and GO–amylopectin–HAP scaffolds on MG 63 cell line as a function of days. Values are expressed as mean ± standard deviation (P < 0.0001, n = 3). | |
Table 1 Cell viability of tricomponent composite scaffolds
Scaffold |
Cell viability (%) |
1st day |
2nd day |
3rd day |
4th day |
GO–gellan–HAP |
84.73 |
85.97 |
86.60 |
112.18 |
GO–alginate–HAP |
93.55 |
113.89 |
117.55 |
122.67 |
GO–amylopectin–HAP |
110.11 |
139.00 |
153.11 |
184.22 |
GO and polysaccharides are polar with negatively charged functionalities including hydroxyl and carboxyl groups. The presence of a polar constituent plays a prominent role in the biological functions of cells as they enhance the interaction with the constituent of cells and culture medium. In this case, osteoconductive HAP along with negatively charged GO and polysaccharides (gellan, alginate and amylopectin) enhance the cell matrix interactions through their negatively charged species. Hence, these materials can be used in human body as scaffolds or implants. Among the tricomponent scaffolds, GO–amylopectin–HAP exhibited higher cell proliferation due to higher porosity.21,30,52 This suggest that the pores of the scaffold mainly influence the cell adhesion and proliferation through infiltration of cells on the scaffolds. These results indicated that the higher cell proliferation and cell growth may be due to the influence of multiple factors including negatively charged components, porosity, and incorporation of osteoconductive HAP.
Alkaline phosphatase activity
Tissue engineering is an emerging area, wherein the success of osteogenesis is measured by robust ALP expression which inevitably leads to mineralization of the neotissue. Human have four ALP genes corresponding to intestinal, placental, placental-like and liver/bone/kidney. According to booster hypothesis, the function of ALP is to increase the concentration of inorganic phosphate.53 Differentiation of cells is an important process for bone regeneration. It was reported that the GO included HAP composites showed significantly higher ALP activity. This is a positive indication for the inclusion of GO in the scaffolds for better cell differentiation.18,27 In our in vitro test, the differentiation of MG 63 cells onto scaffolds were determined using ALP activity. ALP activity was used as a biochemical marker for the determination of osteoblast phenotype and was considered as an important factor in determining bone differentiation and mineralization.54–57 ALP activity of prepared tricomponent scaffolds are shown in Fig. 7. ALP on the scaffolds increased with increasing days, which is the positive indication for the mineralization for producing inorganic phosphate ion. Among the scaffolds, GO–amylopectin–HAP exhibited higher ALP activity and this is good correlation with the cytotoxicity assay also.
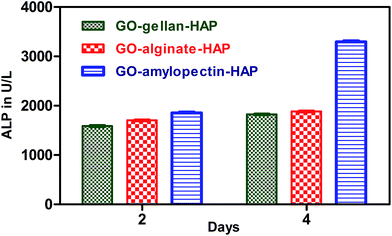 |
| Fig. 7 ALP assay of GO–gellan–HAP, GO–alginate–HAP and GO–amylopectin–HAP scaffolds on MG 63 cell line. Values are expressed as mean ± standard deviation (P < 0.0001, n = 3). | |
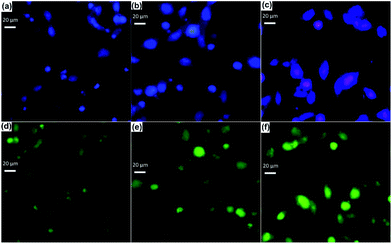 |
| Fig. 8 Optical microscopy images of Hoechst 3342 stained cells grown on (a) GO–gellan–HAP, (b) GO–alginate–HAP and (c) GO–amylopectin–HAP scaffolds and acridine orange stained cells grown on (d) GO–gellan–HAP, (e) GO–alginate–HAP and (f) GO–amylopectin–HAP scaffolds. | |
Cell attachment assay using stains
Cell attachment on tricomponent scaffolds was studied using Hoechst and acridine orange stain and the images are shown in Fig. 8. The fluorescent Hoechst 33342 DNA can be used to detect the adherence of cells to visualize nuclei and mitochondria.11,58 Whereas, acridine orange can bind with double stranded nucleic acid (DNA) and emits green fluorescence or red fluorescence if bound to single stranded nucleic acid (RNA). Viable cells have uniform bright green nuclei with an organized structure.59 The cell attachment assay on a tricomponent scaffolds of Hoechst stain images (Fig. 8a–c) (blue fluorescence) and acridine orange stain images (Fig. 8d–f) (green fluorescence) demonstrated that the MG 63 cell line was metabolically active. The uniform green fluorescence images observed in the Fig. 8d–f confirmed the presence of viable cells which may be due to the interaction of DNA in the viable cells with acridine orange dye. Most cells on the scaffolds were well distributed and exhibited highly branched morphology after 4 days. The hydroxyl groups present in GO and polysaccharides provide the sites to facilitate cell adhesion by retaining and recruiting cells on to the scaffold surface. Further, hydrophilic GO improves the interfacial adhesion with cell membrane.
From the previous report, it has been concluded that GO provided a platform to construct a biointerface with the dimensional capability for the growth of cells.52 The adhered cells on GO–alginate–HAP and GO–amylopectin–HAP scaffolds were higher when compared to GO–gellan–HAP, due to the porosity differences. This behaviour can be well correlated with the MTT assay. These results provide the evidences for the adhesion of cells on to the tricomponent scaffolds.45
Compressive strength
Scaffold materials used for bone tissue engineering should undergo various amounts of stresses during new bone formation for the protection of cultured cells from damaging and to keep the integrity of the scaffold.60 The roughness of HAP and GO would contribute to mechanical interlocking with the macromolecular chains of polysaccharides. Compressive strength of the freeze dried tricomponent scaffolds was determined and shown in Fig. 9. All scaffold materials exhibited sponge like behaviour. The compressive strength value determined was 466.8 ± 19 for GO–gellan–HAP, 171 ± 17 for GO–alginate–HAP and 161 ± 4 for GO–amylopectin–HAP scaffolds. The decrease in compressive strength is mainly due to the increasing porosity and pore size of the scaffolds along with decreasing viscosity of polysaccharide. Moreover, increasing porosity will act as a barrier against crack propagation due to the uniform distributing of applied stress in the porous.52,61
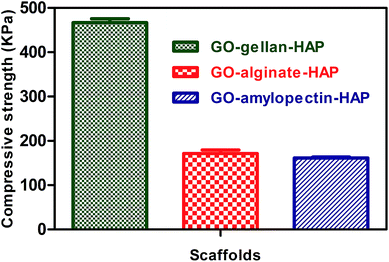 |
| Fig. 9 Compressive strength images of tricomponent scaffolds. Values are expressed as mean ± standard deviation. | |
Experimental
Materials
HAP was isolated from the chicken bones (purchased from local slaughterhouse) by the thermal calcinations method. The MG 63 cell line was obtained from the National Center for Cell Science, Pune, India. Graphite, amylopectin, sodium alginate, gellan gum, 3-(4,5-dimethylthiazol-2-yl)-2,5-diphenyltetrazolium bromide (MTT), acridine orange and bisbenzimide Hoechst 33342 stains were purchased from Sigma Aldrich. Dulbecco's Modified Eagle's Medium (DMEM) was purchased from HIMEDIA. Sodium phosphate buffer, NaCl and KCl were purchased from Merck. NaOH, KMnO4, NaNO3, 30% H2O2, con. H2SO4 (AR grade) were purchased from the S.D. fine chemicals.
Isolation of HAP from chicken bone
HAP was isolated by thermal calcinations method as reported previously by our group.38 The chicken bones were washed with concentrated NaOH solution followed by rinsing with water to remove the traces of meat, skin and other impurities present on the surface of the bones. Then the bones were dried in hot air oven at 100 °C and grained in to small pieces. Pre treated chicken bones were subjected to thermal calcinations at 800 °C with 20 h holding time in an electrical muffle furnace (SUNSIM, India).
Preparation of graphene oxide
GO was prepared from graphite by modified Hummer's method as reported by Marcano et al.62 Con. H2SO4 (23 ml) was added to a mixture of graphite (1 g) flakes and NaNO3 (0.5 g), then the mixture was cooled to 0 °C using an ice bath. KMnO4 (3 g) was added slowly in portions to maintain the reaction temperature below 20 °C. The reaction was warmed to 35 °C and stirred for 7 h. Additional KMnO4 (3 g) was added in one portion, and the reaction was stirred for 12 h at 35 °C. The reaction mixture was cooled to room temperature and poured onto the ice (∼400 ml) with 30% H2O2 (3 ml). The suspension was filtered and successively washed with 30% HCl, double distilled water and ethanol. The obtained solid product was oven dried.
Preparation of GO–polysaccharide–HAP scaffold
1.74 g of polysaccharides (gellan gum or alginate or amylopectin) was dissolved in 100 ml of double distilled water. 60 mg of GO was dispersed in distilled water by sonication and added drop wise to the polysaccharide solution then stirred for 3 h. 4.2 g of isolated HAP was suspended in 50 ml of distilled water and added slowly to the GO–polysaccharide solution and stirred for 24 h. The resultant composite solution mixture was transferred into 12 well plates with 2 g solution per well. The samples were freeze dried at −80 °C and lyophilized with a freeze dryer to form scaffold.
General characterization
The thermal stability of the composite scaffold was studied using thermo gravimetric (TG) (SDTQ 600 TA Instrument, USA) with scan range of 50 °C to 900 °C at a constant heating rate of 10 °C min−1 in the nitrogen atmosphere. The vibrational frequency of the samples was studied by Fourier transformed infrared spectroscopy (FTIR) (Jasco FTIR4100, Japan) and the spectrum was recorded within the range of 4000 cm−1 to 500 cm−1 using KBr. The phase and crystallinity of the samples were examined by powder X-ray diffraction (XRD) (Bruker, D8 Advance X-ray Diffraction spectrophotometer, German) at room temperature using Cu Kα as the radiation source with the wavelength 1.504 Å, over the angle range 10° to 80°, step size 0.02° and scan speed 0.5° min−1. The resultant XRD profile of the isolated HAP was compared with the standard Joint Committee on Powder Diffraction Standards (JCPDS) cards available in the system software. Further, the morphology of the tricomponent scaffolds was studied by field emission scanning electron microscopy (FE-SEM) (SUPRA 55, Carl Zeiss, Germany) analysis.
Porosity measurements
Porosity is an important property for the cell proliferation. The total porosity of the scaffold was obtained by the liquid displacement method.11 Initially, the volume of dry ethanol and dry weight of the scaffold was measured. Then the scaffold was immersed in dry ethanol for 48 h. After 48 h, a scaffold was taken out from ethanol and the weight of the scaffold was measured. The total porosity was calculated using the equation
Porosity = (W1 − W3)/(W2 − W3) |
where W1 = initial weight of the scaffold, W2 = sum of weights of ethanol and submerged scaffold, W3 = weight of ethanol after the removal of scaffold.
In vitro cell proliferation assay of scaffolds
The MG-63 cells were plated separately in 96 well plates at a concentration of 1 × 105 cells per well. After 24 h, cells were washed twice with 100 μl of serum-free medium and starved for an hour at 37 °C. After starvation, cells were seeded with the scaffold material which was previously sterilized with 75% alcohol followed by 100% alcohol. Cells treated without scaffold material was used as a control. At the end of the treatment period of 1, 2, 3 and 4 days, the medium was aspirated and serum free medium containing MTT (0.5 mg ml−1) was added and incubated for 4 h at 37 °C in a CO2 incubator. The determinations were performed using triplicates each time.
The MTT containing medium was then discarded and the cells were washed with phosphate buffer solution (PBS) (200 μl). The formed formazan crystals were then dissolved by adding 100 μl of DMSO and this was mixed properly by pipetting up and down. Spectrophotometrical absorbance of the purple blue formazan dye was measured in a microplate reader at 570 nm (Biorad 680).11,63 Cytotoxicity was determined using Graph pad prism 5 software.
Alkaline phosphatase assay
For the determination of ALP activity, cells were cultured as per the cytotoxicity analysis, after the incubation with scaffolds for 2 and 4 days, the cells were treated with 10 μl (100 mmol l−1) of p-nitrophenyl phosphate and incubated for 30 min at 37 °C in a CO2 incubator. ALP can hydrolyze the p-nitrophenyl phosphate in to p-nitrophenol and inorganic phosphate.64 The released p-nitrophenol absorbance was measured at 405 nm spectrophotometrically. The determinations were performed using triplicates each time.
Hoechst stain assay for cell attachment
Hosechst 33342 DNA staining has been used to identify the cell attachment and growth on scaffold material.11 For this, ethanol sterilized scaffold material was placed in the cell culture plate and seeded with MG 63 cells and incubated. After 4 days of culture, media was removed from the wells and washed with PBS solution. The cells were stained with 0.5 ml of Hoechst 33342 solutions (3.5 μg ml−1 in PBS) and incubated for 30 min at 37 °C. After 30 min, the Hoechst stained cells were visualized and photographed under the Microscope-Olympus-version-6.0, Carl Zeiss lens, Germany.
Acridine orange stain assay for cell attachment
Acridine orange is used to identify both viable and apoptotic cells on scaffold materials.59 For this, ethanol sterilized scaffold material was placed in the cell culture plate and seeded with MG 63 cells and incubated. After 4 days of culture, media was removed from the wells and washed with PBS solution. The cells were stained with 200 μl of dye mixture (100 μl mg−1 acridine orange distilled water). The suspension was immediately examined and viewed under Microscope-Olympus-version-6.0, Carl zeiss lens, Germany.
Compressive strength
The compressive strength of freeze dried scaffolds was obtained using universal testing machine (UTM). The tests were performed using an H5KS system (Tinius Olsen, Salfords, UK). A 5000 N load cell with the standard grips of crosshead speed of 0.5 mm min−1 was used for the compression measurements. The determinations were performed using five replicates.
Statistical analysis
Statistical analysis for MTT, ALP assay and compressive strength were performed using Graph pad prism 6 software and the results are presented as mean ± standard deviation.
Conclusion
In this study, new 3D tricomponent composite scaffolds of GO–gellan–HAP, GO–alginate–HAP and GO–amylopectin–HAP were successfully developed to mimic the role of natural bone and tested for their cytotoxicity for biocompatibility, ALP activity for cell differentiation and mineralization, cell attachment and compressive strength for biomaterials applications. The in vitro studies exhibited the osteoconductivity and biocompatibility of all the scaffolds. The prepared tricomponent scaffolds exhibited comparable compressive strength as reported earlier. The higher cell proliferation and biocompatibility observed in GO–amylopectin–HAP can be attributed to higher pore size and porosity.
Acknowledgements
The authors gratefully acknowledge the management of the VIT University, Vellore, India for the facilities offered to carry out this piece of work. One of the authors R. Rajesh gratefully acknowledges the RA fellowship from VIT University. The authors also acknowledge the powder XRD facility at SAS, VIT University, jointly funded by VIT and FIIST, DST India. Further, the authors thank the Pondicherry Center for Biological Sciences (PCBS), Pondicherry for the in vitro studies.
References
- S. Bose and M. Roy, Trends Biotechnol., 2012, 30, 546 CrossRef CAS PubMed.
- J. Malda and C. G. Frondoza, Trends Biotechnol., 2006, 24, 299 CrossRef CAS PubMed.
- M. E. Gomes and R. L. Reis, Int. Mater. Rev., 2004, 49, 261 CrossRef CAS.
- J. F. Mano, G. A. Silva, H. S. Azevedo, P. B. Malafaya, R. A. Sousa, S. S. Silva, L. F. Boesel, J. M. Oliveira, T. C. Santos, A. P. Marques, N. M. Neves and R. L. Reis, J. R. Soc., Interface, 2007, 4, 999 CrossRef CAS PubMed.
- F. M. P. Tonelli, A. K. Santos, K. N. Gomes, E. Lorencon, S. Guatimosim, L. O. Ladeira and R. R. Resende, Int. J. Nanomed., 2012, 7, 45111 Search PubMed.
- K. W.-H. Lo, B. D. Ulery, K. M. Ashe and C. T. Laurencin, Adv. Drug Delivery Rev., 2012, 64, 1277 CrossRef CAS PubMed.
- K. Y. Lee and D. J. Mooney, Prog. Polym. Sci., 2012, 37, 106 CrossRef CAS PubMed.
- M. V. Jose, V. Thomas, K. T. Johnson, D. R. Dean and E. Nyairo, Acta Biomater., 2009, 5, 305 CrossRef CAS PubMed.
- F. P. W. Melchels, M. A. N. Domingos, T. J. Klein, J. Malda and P. J. Bartolo, Prog. Polym. Sci., 2012, 37, 1079 CrossRef CAS.
- A. L. Rossi, I. C. Barreto, W. Q. Maciel, F. P. Rosa, M. H. Rocha-Leao, J. Werckmann, A. M. Rossi, R. Borojevic and M. Farina, Bone, 2012, 50, 301 CrossRef CAS PubMed.
- J. Venkatesan, R. Pallela, I. Bhatnagar and S.-K. Kim, Int. J. Biol. Macromol., 2012, 51, 1033 CrossRef CAS PubMed.
- J. T. Oliveira, T. C. Santos, L. Martins, L. Picciochi, A. P. Marques, A. G. Castro, N. M. Neves, J. F. Mano and R. L. Reis, Tissue Eng., Part A, 2010, 16, 343 CrossRef CAS PubMed.
- A. R. Shrivats, M. C. McDermott and J. O. Hollinger, Drug Discovery Today, 2014, 19, 781 CrossRef CAS PubMed.
- D. Dyondi, T. J. Webster and R. Banerjee, Int. J. Nanomed., 2013, 8, 47 Search PubMed.
- G. Muzio, G. Martinasso, F. Baino, R. Frairia, C. Vitale-Brovarone and R. A. Canuto, J. Biomater. Appl., 2014, 29, 728 CrossRef CAS PubMed.
- R. Kane and P. X. Ma, Mater. Today, 2013, 16, 418 CrossRef CAS PubMed.
- M. Descamps, J. C. Hornez and A. Leriche, J. Eur. Ceram. Soc., 2009, 29, 369 CrossRef CAS.
- F. Mohandes and M. Salavati-Niasari, RSC Adv., 2014, 4, 25993 RSC.
- P. T. S. Kumar, S. Srinivasan, V. K. Lakshmanan, H. Tamura, S. V. Nair and R. Jayakumar, Carbohydr. Polym., 2011, 85, 584 CrossRef.
- R. Rajesh, N. Senthilkumar, A. Hariharasubramanian and Y. D. Ravichandran, Int. J. Pharm. Pharm. Sci., 2012, 4, 23 CAS.
- J. Venkatesan, Z.-J. Qian, B. Ryu, N. A. Kumar and S.-K. Kim, Carbohydr. Polym., 2011, 3, 569 CrossRef.
- R. Rajesh, A. Hariharasubramanian, N. Senthilkumar and Y. D. Ravichandran, Int. J. Pharm. Pharm. Sci., 2012, 4, 716 CAS.
- R. Rajesh, A. Hariharasubramanian and Y. D. Ravichandran, Phosphorus, Sulfur Silicon Relat. Elem., 2012, 187, 914 CrossRef CAS.
- T. Kuilla, S. Bhadra, D. Yao, N. H. Kim, S. Bose and J. H. Lee, Prog. Polym. Sci., 2010, 35, 1350–1375 CrossRef CAS.
- I. Armentano, M. Dottori, E. Fortunati, S. Mattioli and J. M. Kenny, Polym. Degrad. Stab., 2010, 95, 2126 CrossRef CAS.
- Y. Pan, T. Wu, H. Bao and L. Lin, Carbohydr. Polym., 2011, 83, 1908 CrossRef CAS.
- M. Li, Q. Liu, Z. Jia, X. Xu, Y. Cheng, Y. Zheng, T. Xi and S. Wei, Carbon, 2014, 67, 185 CrossRef CAS.
- T. Ma, P. R. Chang, P. Zheng and X. Ma, Carbohydr. Polym., 2013, 94, 63–70 CrossRef CAS PubMed.
- S. Kang, J. B. Park, T.-J. lee, S. Ryu, S. K. Bang, W.-G. La, M.-K. Noh, B. H. Hong and B.-S. Kim, Carbon, 2015, 83, 162 CrossRef CAS.
- Y. Li, C. Liu, H. Zhai, C. Zhu, H. Pan, X. Xu and R. Tang, RSC Adv., 2014, 4, 25398 RSC.
- P. Gentile, M. Mattioli-Belmonte, V. Chiono, C. Ferretti, F. Baino, C. Tonda-Turo, C. Vitale-Brovarone, I. Pashkuleva, R. L. Reis and G. Ciardelli, J. Biomed. Mater. Res., Part A, 2012, 100, 2654 CrossRef PubMed.
- W. Lu, K. Ji, J. Kirkham, Y. Yan, A. R. Boccaccini, M. Kellett, Y. Jin and X. B. Yang, Cell Tissue Res., 2014, 356, 97 CrossRef CAS PubMed.
- F. J. O'Brein, Mater. Today, 2011, 14, 88 CrossRef.
- L. Meinel, V. Karageorgiou, R. Fajardo, B. Snyder, V. Shinde-Patil, L. Zichner, D. Kalpan, R. Langer and G. Vunjak-Novakovic, Ann. Biomed. Eng., 2004, 32, 112 CrossRef PubMed.
- A. Jonitz, J. Wieding, K. Lochner, M. Cornelsen, H. Seitz, D. Hansmann and R. Bader, Materials, 2011, 4, 1249–1259 CrossRef CAS.
- N. G. Rim, C. S. Shin and H. Shin, Biomed. Mater., 2013, 8, 014102 CrossRef PubMed.
- F. Tamimi, J. Torres, K. Al-Abedalla, E. Lopez-Cabarcos, M. H. Alkhraisat, D. C. Bassett, U. Gbureck and J. E. Barralet, Biomaterials, 2014, 35, 5346 CrossRef PubMed.
- I.-K. Yoon, J.-Y. Hwang, J.-W. Seo, W.-C. Jang, H.-W. Kim and U. S. Shin, Carbon, 2014, 77, 379 CrossRef CAS.
- M. Li, Q. Liu, Z. Jia, X. Xu, Y. Shi, Y. Cheng, Y. Zheng, T. Xi and S. Wei, Appl. Surf. Sci., 2013, 284, 804 CrossRef CAS.
- N. Barbani, M. L. Coluccio, C. Cristallini, G. D. Guerra and E. Rosellini, J. Appl. Polym. Sci., 2010, 118, 3131 CrossRef CAS.
- M. Ionita, M. A. Pandele and H. Iovu, Carbohydr. Polym., 2013, 94, 339 CrossRef CAS PubMed.
- R. Rajesh, Y. D. Ravichandran, N. A. N. Raj and N. Senthilkumar, Polym.-Plast. Technol. Eng., 2014, 53, 1105 CrossRef CAS.
- G. M. Neelgund, A. Oki and Z. Luo, Mater. Res. Bull., 2013, 48, 175 CrossRef CAS PubMed.
- J. Roman, M. V. Cabanas, J. Pena and M. V. Regi, Sci. Technol. Adv. Mater., 2001, 12, 045003 CrossRef.
- G. Ciardelli, V. Chiono, G. Vozzi, M. Pracella, A. Ahluwalia, N. Barbani, C. Cristallini and P. Giusti, Biomacromolecules, 2005, 6, 1961 CrossRef CAS PubMed.
- L. Wang, Y. Li and C. Li, J. Nanopart. Res., 2009, 11, 691 CrossRef CAS.
- D. Leal, B. Matsuhiro, M. Rossi and F. Caruso, Carbohydr. Res., 2008, 343, 308 CrossRef CAS PubMed.
- D. C. Dragunski and A. Pawlicka, Mater. Res., 2001, 4, 77 CrossRef CAS.
- M. Li, Y. Wang, Q. Liu, Q. Li, Y. Cheng, Y. Zheng, T. Xi and S. Wei, J. Mater. Chem. B, 2013, 1, 475 RSC.
- J. F. O'Brien, B. A. Harley, M. A. Waller, I. V. Yannas, L. J. Gibson and P. J. Prendergast, Technol. Health Care, 2007, 15, 3 Search PubMed.
- I. V. Yannas, E. Lee, D. P. Orgill, E. M. Skrabut and G. F. Murphy, Proc. Natl. Acad. Sci. U. S. A., 1989, 86, 933 CrossRef CAS.
- D. Depan, B. Girase, J. S. Shah and R. D. K. Misra, Acta Biomater., 2011, 7, 3432 CrossRef CAS PubMed.
- E. E. Golub and K. B. Battaglia, Curr. Opin. Orthop., 2007, 18, 444 CrossRef.
- H. Wang, Y. Li, Y. Zuo, J. Li, S. Ma and L. Cheng, Biomaterials, 2007, 28, 3338 CrossRef CAS PubMed.
- R. Marom, I. Shur, R. Solomon and D. Benayahu, J. Cell. Physiol., 2005, 202, 41 CrossRef CAS PubMed.
- U. Stucki, J. Schmid, C. F. Hammerle and N. P. Lang, Clin. Oral Implants Res., 2001, 12, 121 CrossRef CAS PubMed.
- L. Pan, X. Pei, R. He, Q. Wan and J. Wang, Colloids Surf., B, 2012, 93, 226–234 CrossRef CAS PubMed.
- A. Stander, S. Marais, V. Stivaktas, C. Vorster, C. Albrecht, M. L. Lottering and A. M. Joubert, J. Ethnopharmacol., 2009, 124, 45 CrossRef PubMed.
- D. Baskic, S. Popovic, P. Ristic and N. N. Arsenijevic, Cell Biol. Int., 2006, 30, 924 CrossRef CAS PubMed.
- X. Wu, Y. Liu, P. Wen, Y. Zhang, Y. Long, X. Wang, Y. Guo, F. Xing and J. Gao, Acta Biomater., 2010, 6, 1167 CrossRef CAS PubMed.
- U.-J. Kim, J. Park, H. J. Kim, M. Wada and D. L. Kaplan, Biomaterials, 2005, 26, 2775 CrossRef CAS PubMed.
- D. C. Marcano, D. V. Kosynkin, J. M. Berlin, A. Sinitskii, Z. Sun, A. Slesarev, L. B. Alemany, W. Lu and J. M. Tour, ACS Nano, 2010, 4, 4806 CrossRef CAS PubMed.
- N. Ramalingam, T. S. Natarajan and S. Rajiv, J. Biomed. Mater. Res., Part A, 2015, 103, 16 CrossRef PubMed.
- J. Venkatesan and S.-K. Kim, J. Biomed. Nanotechnol., 2012, 8, 676 CrossRef CAS PubMed.
|
This journal is © The Royal Society of Chemistry 2015 |