DOI:
10.1039/C5RA06661A
(Paper)
RSC Adv., 2015,
5, 63000-63011
Nitrogen- and oxygen-containing activated carbons from sucrose for electrochemical supercapacitor applications†
Received
14th April 2015
, Accepted 14th July 2015
First published on 14th July 2015
Abstract
Nitrogen- and oxygen-containing activated carbons have been synthesized from sucrose and ammonium nitrate (AN) by carbonization at different temperatures (600–900 °C) under a flow of nitrogen gas with steam. Another set of carbons has been synthesized without AN. The carbons have been characterized using X-ray diffractometry (XRD), elemental analysis, solid-state 13C nuclear magnetic resonance (NMR) spectroscopy, X-ray photoelectron spectroscopy (XPS), thermo-gravimetric analysis (TGA), di-nitrogen adsorption–desorption, Fourier transform infrared (FTIR) spectroscopy, scanning electron microscopy (SEM) and transmission electron microscopy (TEM) techniques. Nitrogen from AN has been found to be incorporated into carbon samples. Electrochemical performances of the carbons have been studied in 1 M sulphuric acid using cyclic voltammetry and galvanostatic charge–discharge cycling. The use of AN favors the formation of carbons with higher surface areas and higher graphitic nature. One of the carbons with a BET surface area of 518 m2 g−1, a nitrogen content of 3% and an oxygen content of 20.4% shows a specific capacitance of 277 F g−1. Carbons obtained using AN show better capacitance than the ones obtained without AN. Higher carbonization temperatures favor the formation of carbon with the higher capacitance values.
1. Introduction
In recent years, supercapacitors have become some of the most important components of charge storage technologies with the advantages of high power density (10 kW kg−1), high charge/recharge rate (seconds) and long cycle life (>105).1,2 There are two types of capacitance known thus far and those are electric double layer capacitance (EDLC) involving charge storage by simple electrostatic attraction between the surface of the charged electrode and the electrolyte ions, a non-faradaic process, and pseudocapacitance involving fast and reversible reduction or oxidation, a faradaic process, of the bulk or surface of the electrode.3,4 In general, carbon materials are known to exhibit EDLC characteristics as they show fairly good electric conductivity, inertness, high surface area and suitable porosity.5 Nevertheless, the presence of certain oxidizable/reducible functional groups in carbon is responsible for it to show pseudocapacitance characteristics besides EDLC.6 Carbon materials are suitable candidates for capacitor applications due to the abundance, significant to high electrical conductivity, chemical (both in acid and alkali) and electrochemical (high operational window of potential) stabilities and light weight due to the low atomic weight of 12 (to show high gravimetric capacity).5,7 Among different types of carbons known, activated carbons have advantages such as low cost, higher surface area and tunability of their pore sizes by activation processes. Moreover, in the case of composite electrodes based on metal oxide or conducting polymer with activated carbons, activated carbons are used as conductive-additive for improving the rate performance or as matrix for improving cycling ability by buffering volume changes, respectively.7 The activation processes employed to improve the surface areas and pore sizes of carbons are classified into chemical and physical activations. The chemical activation process deals with the use of main activating agents such as KOH, ZnCl2 and H3PO4 whereas the physical activation deals with mainly the use of activating agents such as steam, CO2 and air.8 Although the chemical activation leads to higher surface area, the residues (ash) left behind in the resulting carbon demand tedious post-synthesis work-ups. In contrast, steam activation does not cause the incorporation of any residues into carbon, except for oxygen, so post-synthesis cleaning is not necessary in this case.
Sucrose is one of the carbon sources, because it, being a carbohydrate, can be carbonized by dehydration to get carbon in a facile manner.9 Carbons obtained from sucrose are free from impurities and inexpensive.10 Presence of nitrogen and oxygen in the lattice or nitrogen- and oxygen-based functional groups in carbon shows many advantages such as enhanced conductivity,11 offering pseudocapacitance5,12 and increased wettability towards aqueous electrolytes.5 In order to make a N-containing carbon, N-containing precursors such as melamine-formaldehyde,13 polypyrrole, polyaniline14 or waste coffee15 have been carbonized, carbon has been impregnated with the N-containing compounds such as melamine or urea before a heat-treatment,16 or using NH3 (ref. 17) or NH3–air mixture (for ammoxidation) during carbonization.7,18 Incorporation of oxygen in the carbon materials is performed by using O-containing precursors such as sucrose,19 activation with steam, KOH or air,8 treatment with HNO3,20 oxygen plasma21 or electro-oxidation.22 A combustion method dealing with synthetizing nanoparticles of different oxides using sucrose as the fuel, polyvinyl alcohol (PVA) as the matrix and nitrate salt of the metal or non-metal, whose oxide is of interest, as the combusting agent has been reported.19 Upon evaporation of water from the mixture, formation of a fluffy material was observed, which later was heated in air atmosphere to obtain nanoparticles of the metal oxide by removing the carbonaceous matters from sucrose and other impurities. Herein, we report the synthesis of activated carbons using sucrose as the carbon source and fuel, and ammonium nitrate as the combusting agent and nitrogen source. The fluffy material obtained was heat-treated under a flow of nitrogen gas with steam to obtain activated carbons. For the best of authors' knowledge, synthesis of nitrogen (N)-containing carbon from the mixture of sucrose and ammonium nitrate has not been reported yet. Ammonium nitrate is used here, because it decomposes completely into gaseous products at temperatures as low as 210 °C.23 So, the unreacted ammonium nitrate can be removed easily during the carbonization process.
2. Experimental section
2.1. Methods
2.1.1. Synthesis of activated carbons. In a typical synthesis, 15 g of sucrose (C12H22O11) and 5 g of ammonium nitrate (NH4NO3) (AN) were dissolved in 80 ml volume of DI water and aged in an air oven at 120 °C for 24 h. A fluffy material was observed and it was carbonized in a horizontal tubular furnace using a quartz boat at temperatures, viz., 600, 700, 800, and 900 °C, for 6 h in a stream of nitrogen gas containing water vapors. Nitrogen gas was passed through a water bubbler (cylindrical; 120 ml volume; 50% filled with DI H2O) at 29 °C at a flow rate of 50 ml min−1. Heating ramp of the furnace was 25 °C min−1. After carbonization, samples were naturally cooled to room temperature under the nitrogen gas flow. Another set of samples with 2
:
1 wt ratio of sucrose and AN was synthesized. For comparison, a set of carbon samples was synthesized from sucrose in the absence of AN under the similar conditions. In this case, no fluffy but a hard and pasty stuff was observed. The carbon samples synthesized using 3
:
1 and 2
:
1 wt ratios of sucrose and AN are denoted as ‘CNA’ and ‘CNB’. Carbons obtained from sucrose alone are marked as ‘C’. Temperature (in °C) of the carbonization is suffixed with the code of sample without unit. The as-synthesized samples were ground well with a mortar-pestle before characterization.
2.2. Characterization
Powder X-ray diffraction (XRD) patterns of the carbon samples were recorded using Rigaku MiniFlex2 diffractometer at a scan rate of 0.1° s−1 with Ni-filtered Cu Kα radiation (λ = 1.5406 Å) as the X-ray source. CHN elemental analyses of the samples were carried out with a Perkin-Elmer 2400 II series CHNS/O analyzer. Solid-state 13C NMR studies were carried out using a Bruker Avance™ 400 MHz instrument of probe head with a zirconium rotor of 4 mm diameter. The entire spectrum was recorded at a spinning speed of 12 kHz. X-ray photoelectron spectra (XPS) were recorded using an Omicron NanoTechnology instrument with Mg Kα radiation. The base pressure of the analysis chamber during the scan was 2 × 10−10 mbar. The spectra were recorded with a step width of 0.05 eV. The pass energies for individual and survey scans were 20 and 100 eV, respectively. Data were processed using the CasaXPS program (Casa Software Ltd., UK). Thermo-gravimetric analysis (TGA) was performed with Perkin-Elmer TGA (Delta series TGA7) instrument at a heating rate of 10 °C min−1 in air atmosphere. Nitrogen adsorption–desorption experiments were carried out at −196 °C using a Carlo-Erba Sorptomatic (model 1990) sorpometer and a Micrometrics ASAP 2010 accelerated surface area systems after degassing samples at 150 °C for 12 h. Specific surface areas of the samples were determined based on the Brunauer–Emmett–Teller (BET) method. The total pore volume was calculated from the amount of nitrogen adsorbed at a relative pressure of P/P0 = 0.99. Micropore and mesopore size distributions were calculated by Horvath–Kawazoe (HK) and Barrett–Joyner–Halenda (BJH) methods respectively. FTIR spectra of the samples were recorded using a Perkin-Elmer spectrum 1 instrument in the range 400–4000 cm−1 at room temperature. The powdered samples were ground with KBr and pressed into pellets for recording the FTIR spectra. Scanning electron micrographs were recorded using a FEI Quanta FEG 200 scanning electron microscope working at 30 kV. Transmission electron micrographs were collected using a Philips CM12 microscope operating at 100 kV accelerating voltage. Sample for TEM imaging was prepared by dispersing the sample in ethanol by sonication followed by drop-drying on copper grids coated with carbon film.
2.3. Electrode fabrication and electrochemical measurements
Electrochemical performances of carbon samples were studied using cyclic voltammetry curves and charge–discharge cycling. Fabrication of electrodes for electrochemical studies was done as follows. In a typical procedure, 10 mg of carbon samples was dispersed in 0.25 ml of doubly distilled water in a vial by ultrasonication. From the colloid, a volume of 10 μl was transferred on to polished glassy carbon surface, dried at 60 °C to get the semisolid and 5 μl of Nafion solution (5 wt% in lower aliphatic alcohol and water) was coated on the sample as the binder.24 Electrochemical studies were performed in 50 ml of 1 M sulphuric acid solution. Cyclic voltammograms were recorded at a scan rate of 10 mV s−1 in the potential window of 0 to 1 V (vs. Ag/AgCl) and galvanostatic charge–discharge curves were collected with a current of 0.5 mA. Cyclic voltammetric and galvanostatic charge–discharge experiments were carried out using a CHI 660A (CH Instruments, USA) electrochemical work station. For the electrochemical studies, a three-electrode cell composing a polished glassy carbon (GC) (0.07 cm2) working electrode, a Ag/AgCl (Satd. KCl) reference electrode and a Pt foil (5 cm2) counter electrode was used. Nitrogen gas was purged through the electrolyte solution for removing dissolved oxygen before and during the electrochemical measurements.
3. Results and discussion
3.1. Yield and elemental analysis
A photograph of precursors corresponding to C and CNB samples is shown in Fig. 1. It is clear that formation of fluffy materials is observed only in the case of using NH4NO3 with sucrose due to the combustion that occurs. The fluffy material forms due to the sudden evolution of gases, taking place due to combustion. The precursor from sucrose alone is highly sticky whereas the fluffy material is crispy and deliquescent. It was observed that higher the AN content, the higher would be the volume of fluffy material. The yields of the synthesized carbons are provided in Table 1. The C samples show the highest yield of 84.2% and increase in the temperature of synthesis consistently reduces the yield. CNA and CNB samples show lower yields than C samples. Between CNA and CNB samples, CNA analogues show higher yield than CNB. The loss of carbon is expected to take place due to the decomposition of sucrose, the precursor, during the initial treatment with AN and during the carbonization at higher temperatures, and burning-off of the carbon occurring due to steam activation. The lower yields shown by CNA and CNB are due to the loss of carbon into escaping gaseous products, which in turn due to the high degree of decomposition of precursor.
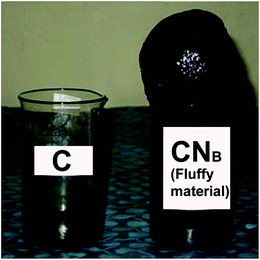 |
| Fig. 1 Photograph of precursors used for the synthesis of C and CNB carbon samples. The precursors were obtained from aqueous sucrose (C) and aqueous mixture of sucrose and ammonium nitrate (CNB). | |
Table 1 Yield, interplanar distance, crystallite size, average number of layers, elemental composition and textural properties of carbon samples
Sample |
Yield (%) |
d(002) (Å) |
Lc (Å) |
Nc |
C (%) |
H (%) |
N (%) |
O (%) |
BET surface area (SSA) (m2 g−1) |
Total pore volume (cm3 g−1) |
C600 |
84.2 |
3.92 |
5.96 |
1.52 |
73.5 |
3.7 |
0 |
22.8 |
11 |
0.01 |
C700 |
81.4 |
3.92 |
5.73 |
1.46 |
80.1 |
2.4 |
0 |
17.5 |
16 |
0.03 |
C800 |
77.4 |
3.71 |
7.25 |
1.95 |
78.8 |
2.2 |
0 |
19 |
138 |
0.11 |
C900 |
75.1 |
3.71 |
7.42 |
2.00 |
77.2 |
2.4 |
0 |
20.4 |
205 |
0.13 |
CNA600 |
68.2 |
3.66 |
7.40 |
2.02 |
78.5 |
2.3 |
7.1 |
12.1 |
105 |
0.07 |
CNA700 |
65.0 |
3.64 |
7.81 |
2.15 |
77.5 |
2.0 |
5.6 |
14.9 |
277 |
0.21 |
CNA800 |
61.1 |
3.59 |
9.38 |
2.61 |
79.0 |
2.0 |
4.0 |
15 |
370 |
0.22 |
CNA900 |
58.4 |
3.54 |
8.80 |
2.49 |
74.4 |
2.3 |
2.3 |
21 |
489 |
0.32 |
CNB600 |
63.6 |
3.66 |
7.60 |
2.07 |
75.7 |
2.3 |
8.8 |
13.2 |
47 |
0.06 |
CNB700 |
59.2 |
3.59 |
8.52 |
2.38 |
77.7 |
2.0 |
7.1 |
13.2 |
320 |
0.18 |
CNB800 |
56.8 |
3.50 |
9.72 |
2.78 |
76.5 |
2.0 |
4.1 |
17.4 |
477 |
0.25 |
CNB900 |
53.7 |
3.50 |
9.09 |
2.60 |
74.6 |
2.0 |
3.0 |
20.4 |
518 |
0.28 |
C, H, N and O elemental composition of the carbon samples are given in Table 1. The oxygen content (wt%) of the carbon samples have been calculated by subtracting the sum wt% of C, H and N elements from hundred. The C, H and O content of sucrose is 42.11, 6.43 and 51.46 respectively. The carbon content of the synthesized samples range from 73.5 to 80.1%. This implies that carbon content has increased due to the carbonization at the expense of hydrogen and oxygen (i.e., dehydration). Hydrogen content of the carbon samples ranges from 2 to 3.7%. Hydrogen content of C samples is found to be higher than that of CNA and CNB samples, implying that the combustion might be the reason for the observed high degree of hydrogen removal and/or that C sample might have smaller and more polynuclear aromatic hydrocarbons (PAHs). Also, the presence of lower content of H indicates the presence of bigger and lesser condensed polynuclear aromatic hydrocarbons in carbon because H content arises mainly from the periphery of the PAHs.25 The presence of nitrogen in the carbons (CNA and CNB) obtained via combustion route by using AN is evidenced. And, it is notable that C samples are nitrogen-free. These observations reveal that nitrogen in CNA and CNB is from AN that was used as the combustion agent, but not from the nitrogen gas stream used for carbonization. Although the N content is as high as 8.8% in the case of CNB600, it is only 3% for CNB900. This observation indicates that amount of nitrogen incorporated in the carbon decrease when the temperature of carbonization increases. Oxygen content of the carbons is in the range of 12.1 to 22.8%. Except for C samples, O content of the carbons is found to increase with the increase in the carbonization temperature. This increase might be depending on the degree of reactivity between steam and carbon, which probably increases with the raise in the temperature from 600 °C to 900 °C.
3.2. Powder XRD study
XRD powder patterns of C, CNA and CNB samples obtained at different temperatures are given in Fig. 2. The broad peaks in the 2θ range of 22.7° to 25.5° and 43.7° to 43.8° respectively correspond to the (002) planes and (101) planes of amorphous carbon with hexagonal phase (JCPDS file no 41-1487).26 The interplanar distances, d, of (002) peaks correspond to interlayer (graphene) distances of carbons and are provided in Table 1.26 The d(002) of graphite is 3.35 Å and the d(002) values of the synthesized carbons deviate from that of graphite by different magnitudes. For the set of C, CNA and CNB samples, the minimum d(002) value are 3.71, 3.54 and 3.50 Å respectively. This observation reveals that CNA and CNB samples show d(002) values closer to that of graphite, meaning CNA and CNB samples are more graphitic than C samples. Moreover, increase in the carbonization temperature shrinks the interlayer distance (d(002)) or increases the degree of graphitization in all samples. Average crystallite sizes, Lc, of carbons calculated using Scherrer's equation from XRD peaks corresponding to (002) planes are attributed to the size of the crystallites along the ‘c’ axis of the hexagonal lattice and given in Table 1. The Scherrer's equation used for the calculation is Lc = 0.89λ/β
cos
θ, where, ‘λ’ is wavelength of X-rays, ‘β’ is full width at the half maximum of (002) diffraction peak and ‘θ’ is Bragg angle of the peak.27 The highest Lc shown by C samples is found to be 7.42 Å, which is lower than that of CNA (9.38 Å) and CNB (9.72 Å) samples. Calculation of La using (10) peak has not been done due to that (10) peaks of carbons studied here are too small and also merged with that of (101) peaks. Average numbers of layers ‘Nc’ of carbons along the ‘c’ axis of their hexagonal lattice are calculated by dividing their Lc values by their d(002) values and given in Table 1. The Nc values show that highest Nc of C samples is 2 while those of CNA and CNB are 2.61 and 2.78 respectively. This implies that CNA and CNB samples possess more carbon layers in ‘c’ axis.28 These observations imply that the combustion route followed to synthesize carbon samples might be responsible for the lower d values and the higher Lc of the CNA and CNB carbons.
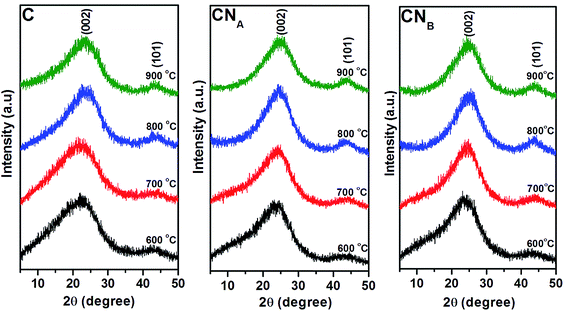 |
| Fig. 2 Powder XRD patterns of carbon samples synthesized at different temperatures. | |
3.3. 13C NMR and FT-IR spectral studies
In order to understand the changes in the nature of carbons present in the synthesized carbon samples, solid-state 13C NMR spectra of sucrose, fluffy material of CNA and carbon samples synthesized at 800 °C were recorded, and the spectra are provided in Fig. 3. The six peaks observed for sucrose are in the range of 63–106 ppm, which correspond to the aliphatic carbons of carbohydrates.29–31 The intense peak centered at 76 ppm corresponds to hydroxylated methylene carbon.29 Moving onto the fluffy material, a single broad peak centered at 76 ppm is observed, corresponding to hydroxylated methylene carbon as in the case of sucrose. However, merging of six peaks to a single peak has taken place during the treatment of sucrose with AN, indicating that the nature of sucrose has changed due to its reaction with AN. In the case of C800, there are three predominant peaks seen and their positions are at 124, 158 and 198 ppm. And, the peak at 124 ppm indicates the presence of the sp2 carbon which corresponds to polynuclear aromatic hydrocarbons or graphite-like carbon while the one at 158 ppm belongs to the aromatic carbon with oxygen functionalities such as hydroxyl (phenolic). The peak present at 198 ppm corresponds to aromatic carbon attached to carbonyl carbons such as aldehyde and ketones.31 The peak at 158 ppm is more intense, indicating that phenolic carbon is present in higher amount than normal aromatic carbon and also that more of oxygen functionalities is present in C800. As for CNA800 and CNB800, only the broad single peaks centered respectively at 127 ppm and 131 ppm are observed. These peaks are attributed to the graphite-like carbons. Thus, 13C NMR spectral studies show that aliphatic carbons from sucrose or fluffy materials are converted into graphite-like aromatic carbons.
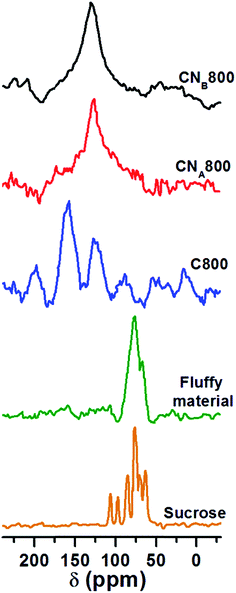 |
| Fig. 3 Solid-state 13C NMR spectra of carbon samples synthesized at 800 °C, fluffy material and sucrose. | |
FTIR spectra of sucrose, fluffy material of CNA, C, CNA and CNB carbons are given in Fig. 4. Sucrose shows absorption bands at 3385, 2948, and 1434 cm−1, corresponding to O–H stretching, C–H stretching and CH2 scissoring vibrations respectively. And, peaks observed at 1060 and 991 cm−1 are attributed to C–O stretching and deformation vibrations respectively. The band at 572 cm−1 is due to C–O–C deformation vibrations.32,33 C600 shows a weak band for O–H stretching along with C–H stretching vibration in addition to a weak band located at 1718 cm−1, which corresponds to C
O vibrations. The band at 1585 cm−1 is probably a collective band that is contributed by the aromatic ring mode, conjugated systems such as ketoester, diketone, and carboxylate structures.34 The broad band observed between 1500 and 1060 cm−1 is owing to C–O stretching and O–H bending (in-plane) vibrations. The weak bands observed at 876 and 800 cm−1 are due to C–H bending vibrations.35,36 Strong and more intense bands observed for sucrose become weak while moving on to carbons due to the formation of the rigid, extended network of carbon from the molecular species, i.e., sucrose. This observation confirms that, during carbonization, aromatic C
C bond is generated from aliphatic carbons of sucrose and that removal O–H groups have occurred by dehydration. On increase in the carbonization temperature to 800 and 900 °C, C–H bonds disappear and free O–H groups are generated as evidenced by the weak bands seen at 3835 and 3740 cm−1.37
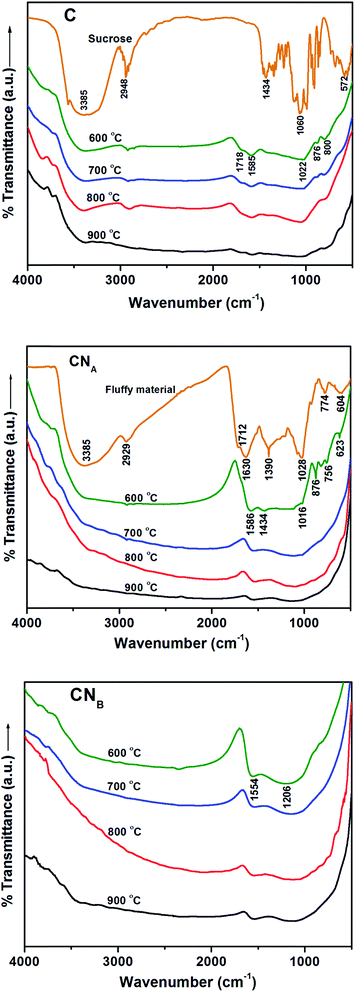 |
| Fig. 4 FTIR spectra of carbon samples synthesized at different temperatures. | |
Moving onto the fluffy material, a pair of new bands is observed at 1712 and 1630 cm−1. These bands might be because of C
O stretching vibrations and these carbonyl functional groups might have been created due to the oxidation of sucrose, which probably takes place due to the combustion. However, bands pertaining to C
O stretching are absent in the case of CNA600, implying that the carbonyl groups are removed during carbonization process at 600 °C or the peak shifted to low frequencies due to the formation of extended carbon network. The tiny bands observed below 900 cm−1 are probably due to O–H bending (out-of-plane) and C–H bending vibrations. As for CNA700, a small band at 1566 cm−1 is probably a collective band that is contributed by the aromatic ring mode, conjugated systems such as ketoester, diketone, and carboxylate structures.34 The band covering 1450 to 1000 cm−1 corresponds to C–O stretching as in ether or phenol.38,39 Possibility of phenolic C–O bond is low or ruled out due to the absence O–H band in the IR spectra. Further increase of carbonization temperature to 800 and 900 °C has caused C
C stretching vibrational bands to shift bathochromically to 1534 and 1542 cm−1 respectively. The rationale for these shifts is that extension of carbon network (i.e., increase in conjugation) might have occurred due to the carbonization performed at higher temperatures. On the other hand, CNB600 shows the only bands of aromatic ring (C
C) of conjugated systems with ketoester, diketone, and carboxylate structures and of C–O stretching, implying that, as compared to C and CNA analogues, CNB samples underwent a higher degree of carbonization.34 This higher degree of carbonization shown by CNB is probably due to the use of more AN. So, there are definite differences between the carbons obtained with and without the use of AN. There is a possibility that some C
N stretching bands might be present in the spectra of CNA and CNB, because the presence of N is evidenced by CHN and XPS results. However, those bands might have merged with aromatic ring (C
C) stretching bands.
3.4. XPS analysis
In order to understand the chemical state of carbon, oxygen and nitrogen elements, XPS were recorded for C900 and CNB900 samples and the results are presented in Fig. 5. The C1s spectrum of C900 shows a peak which is resolvable by deconvolution into 3 peaks, namely, C-I, C-II and C-III, with the binding energy values of 284.7, 286.4 and 288.3 eV and with the peak areas (%) of 54.1, 33 and 12.9, respectively. The peak C-I corresponds to graphitic carbon while C-II corresponds to carbon in phenol and/or ether groups.22,40–43 And, the peak C-III is attributed to carbonyl and/or quinone functionalities. In the case of C900, there was no peak observed for nitrogen in the N1s region (data not given). The resolved O1s spectrum of C900 shows two peaks (O-II and O-III) centered at 533.3 and 535.7 eV with the peak areas of 91.1 and 8.9%, respectively. The peak O-II is due to oxygen in phenol and/or ether while the peak O-III is due to chemisorbed oxygen (carboxylic group) and/or water, respectively.20,34,40,42,44,45
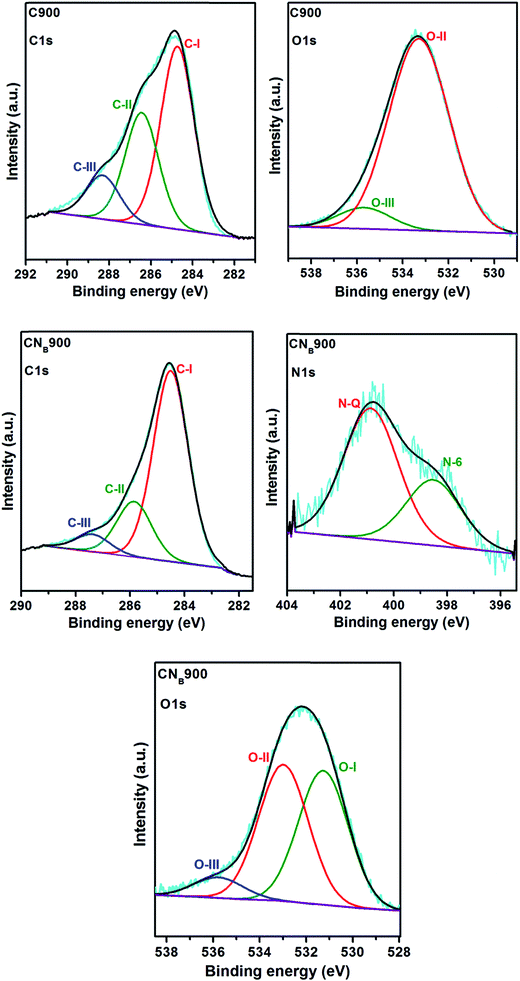 |
| Fig. 5 XPS of C900 and CNB900 samples. C1s and O1s core level spectra of C900 and C1s, N1s and O1s core level spectra of CNB900. | |
The C1s spectrum of CNB900 exhibits three resolved peaks (C-I, C-II and C-III), centered at 284.5, 285.9 and 287.5 eV respectively. The percentages of relative peak areas are 68.2, 22.4 and 9.4. Similar to C900, C-I is attributed to graphitic, C-II is attributed to phenolic, and/or ether groups and C-III peak is attributed to carbonyl and/or quinone functionalities.22,40–43 It is interesting to note that the relative area of graphitic carbon is higher for CNB900 (68.2%) than C900 (54.1%). The N1s spectrum shows two resolved peaks located at 398.6 (area: 33.7%) and 400.9 eV (area: 66.3%), corresponding to pyridinic (N-6) and quaternary (N-Q) nitrogens respectively.12,20,34,43 As there is nitrogen in the sample, C–N bond might have also contributed to peaks C-II and C-III in C1s spectrum of CNB900.46–48 The N1s spectra imply that N atom has substituted some C atoms in the carbon lattice. The O1s spectrum of CNB900 exihibits three resolved peaks (O-I, O-II and O-III), positioned at 531.3, 533 and 535.8 eV with the relative peak areas of 45.9, 47 and 7.1% respectively. The peak O-I is indexed to quinonic and/or carbonyl oxygen and O-II is indexed to oxygen(s) in phenol and/or ether. And, O-III corresponds to chemisorbed oxygen (carboxylic group) and/or water.36,39,41–44
3.5. BET surface area and TG-DTG analyses
BET surface areas and total pore volumes of the carbon samples are given in Table 1. All the three sets of carbon samples show increase in the surface area with the increase in the carbonization temperature. This increase of surface area is due to the generation of new pores which is, in turn, due to the burning off of the carbon occurring by the reaction of carbon with steam.49 High temperatures are found to be effective to burn carbon so that more pores are created. In the current work, sintering and burning of carbon take place simultaneously because the carbonization and the activation are performed simultaneously. The C samples show surface area values in the range of 11–205 m2 g−1. On the other hand, CNA samples show higher surface area values, ranging from 105–489 m2 g−1. Surface area of CNA900 is more than twice as high as that of C900. This implies that fluffy material obtained by the combustion of the mixture has yielded the carbon samples of greater surface area than the C samples. In the case of CNB900, surface area of as high as 518 m2 g−1 is observed. However, surface area of CNB900 is higher by only 29 m2 g−1 than CNA900, even though the ratio of AN to sucrose used is higher in the case of CNB900. This implies that after a certain amount of AN, its quantity may not have a considerable effect on surface area of the resulting carbon. The total pore volumes of the all carbon samples lie between 0.01 and 0.32 cm3 g−1. Similar to surface area, pore volume also increases with the increase in the temperature. These lower pore volumes (<0.32 cm3 g−1) observed for the materials with high surface areas indicate that the carbon samples are microporous.50,51
Nitrogen adsorption–desorption isotherm and BJH and Horvath–Kawazoe pore size distributions of CNB900 are provided in Fig. 6. Fig. 6a shows the type I isotherm of IUPAC classification for CNB900, which is characteristic of microporous material.52 BJH pore size distribution in the inset of Fig. 6a shows that average pore diameter lies below 2 nm and confirms that CNB900 is mainly microporous. Horvath–Kawazoe pore size distribution of CNB900 in Fig. 6b shows the sharp peak, centered in the region of micropores, and this implies that the average pore width of CNB900 is 0.5 nm. Also, t-plot surface area and the external surface area of CNB900 are 458 m2 g−1 and 60 m2 g−1 respectively. This implies that 88.4% of the total surface area of CNB900 is contributed by its micropores. Three dimensionally porous graphene balls obtained from coal tar pitch by KOH activation has shown SSA up to 1947 m2 g−1.53 An animal cellulose precursor has yielded an O-rich activated carbon with a SSA of 3442 m2 g−1 with KOH activation.54 N-carbon nanosheets derived from the Typha orientalis plant in the presence of NH3 (99.9%) exhibit a SSA of 1498 m2 g−1.43 A bean dregs-derived activated carbon activated with KOH shows a SSA of 2876 m2 g−1.55 A sucrose-derived hydrochar, by the activation with steam and KOH, has yielded activated carbons with SSAs of 814 and 2431 m2 g−1 respectively.10 According to another report, KOH activation of sucrose-derived carbon spheres yields a carbon with a SSA of 2823 m2 g−1.56 A sucrose-derived activated carbon obtained by phosphoric acid activation has been reported to show a SSA of 460 m2 g−1.57 A carbon derived from sucrose with ZnCl2 activation shows a SSA of 1434 m2 g−1.58 Activated carbons synthesized from sucrose with CO2 activation show SSAs up to 2953 m2 g−1.59 These reports imply that KOH and CO2 activations offer better SSAs for activated carbons obtained from sucrose than the other activation methods.
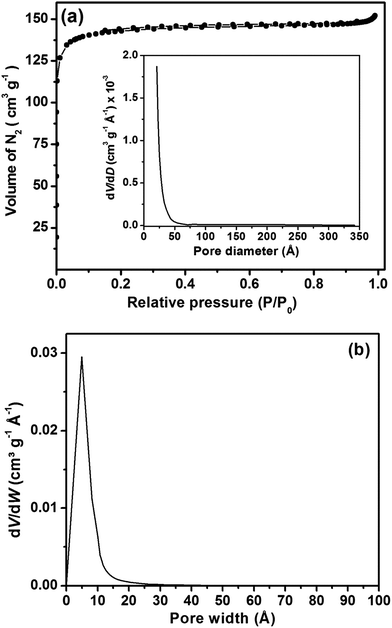 |
| Fig. 6 Nitrogen adsorption–desorption isotherm (a), BJH pore size distribution (inset of a) and Horvath–Kawazoe pore size distribution (b) of CNB900. | |
TG-DTG profiles of C, CNA and CNB sample synthesized at 900 °C, performed in air atmosphere are given in Fig. 7. TGA and DTG of C900 indicates that there are three different events of weight loss occurring. According to DTG, those events centere at 314, 538 and 616 °C and the corresponding weight losses are 8.9, 57.7 and 29.9%. In the cases of CNA900 and CNB900, only two weight losses are observed and the major weight losses occur at 590 and 597 °C respectively. As for CNA900 and CNB900, the starting points of the major weight losses are 438 and 448 °C respectively and these major weight losses correspond to 89.9 and 90.4% respectively. Their initial steady weight losses occurring up to 438 and 448 °C correspond to 8.4 and 8.3% respectively. The initial weight loss occurring below 448 °C is due to the removal of water molecules and volatile matter present in the carbon.52,60 The major weight loss of the carbon occurs due to the burning of the carbon by reacting with oxygen present in the air. The low temperature burning of C900 happens due to the possession of higher amount of functional groups and/or its lower degree of graphitic nature. Among the three samples under study, CNB900 shows the best thermal stability. The higher thermal stability shown by the CNA900 and CNB900 might be due to that they may not possess a larger number of easily-oxidizable (thermally) functional groups and/or their higher degrees of graphitic nature.61
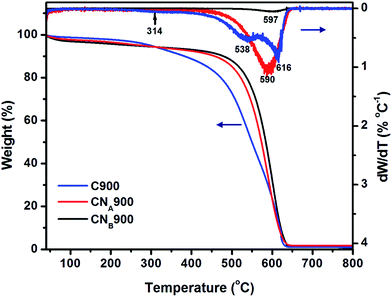 |
| Fig. 7 TG-DTG profiles of carbon samples synthesized at 900 °C, recorded in air atmosphere. W and T refer to weight and temperature respectively. | |
3.6. Electron microscopic studies
SEM images of carbon samples synthesized at 900 °C are given in Fig. 8. SEM images of all carbons show particles of non-spherical morphologies including sheet and of polydispersity. C900 in Fig. 8a shows the particles of size in the range of ∼250 nm to ∼9 μm. And, CNA900 shows particles of size between ∼200 nm and ∼18 μm (Fig. 8b). As for CNB900 (in Fig. 8c), the size ranges from ∼200 nm to ∼21 μm. SEM analysis reveals that the synthesized carbons are of wide particles size distribution. Similar morphologies for carbons derived from sucrose have already been reported.9,59 XRD and SEM analyses complementarily prove that the fine carbon crystallites (sheets) are randomly arranged in carbon particles of size in the range of several nanometers to several micrometers. In other words, the carbon particles are polycrystalline in nature.60 TEM image of CNB900 shown in Fig. 8d shows the presence of particles of different shapes and sizes. Besides, there are several sheet-like particles seen in the TEM. These sheets must be made of several graphene layers. The relatively higher transparency (to electron beam) shown by these sheets is due to their lower thicknesses and the electrons can penetrate these sheets. In addition to sheets, there are aggregates of small particles seen, whose average size lies below 10 nm.
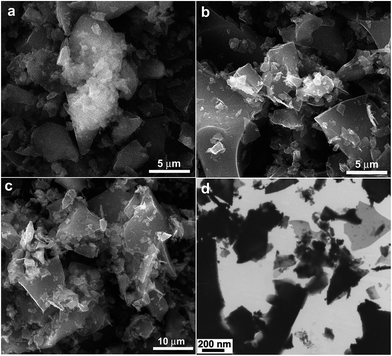 |
| Fig. 8 SEM images of C900 (a), CNA900 (b) and CNB900 (c) and TEM image of CNB900 (d). | |
3.7. Mechanism of formation of carbons
According to 13C NMR and FTIR results, carbons from gummy material and fluffy materials are different in terms of graphitic nature and textural properties. Due to the decomposition of AN after the evaporation of water from the aqueous mixture of sucrose and AN, a lot of heat and gases such as N2, H2O, O2, OH, HNO, NO3 might evolve. It is because decomposition of AN is exothermic.23 Charring of sucrose occurs due to the heat generated, and the foamy, voluminous appearance of the fluffy precursor is given rise to due to the sudden evolution of gases. There is a possibility that sucrose might react with AN and its decomposition products, which is evident from the presence of nitrogen revealed by CHN elemental and XPS analyses. As for both gummy and fluffy precursors, the carbons are aliphatic in nature. In the case of precursor formed with the use of sucrose alone, caramel, a polymer of sucrose, is only formed.29,62 Further, upon carbonization process of both precursors at high temperatures, subsequent polymerization by condensation reactions and aromatization by dehydration as well as dehydrogenation reactions to form polynuclear aromatic hydrocarbons (PAHs) occur.8,63 Subsequently, formation of graphene layers and their ordering (graphitization) to certain extent might take place.64,65 In general, the degree of graphitization is found to be low in sucrose-derived carbons even if the treatment temperature is as high as 1400 °C.62 Steam activation also has been known to favor the graphitization process.49
3.8. Electrochemical studies
Cyclic voltammograms (CVs) of carbon samples synthesized at different temperatures were collected in order to understand the nature of the capacitance operating in the case of carbon materials under study. Shape of the CV indicates the nature of the capacitance operating as to whether it is EDLC, pseudocapacitance or a combination of both. CVs of C, CNA and CNB samples synthesized at 900 °C are given in Fig. 9a and of C and CNB samples synthesized at 600–800 °C are given in Fig. S1a and S2a of ESI.† The observed shapes of CVs are attributed uniquely to carbon materials, revealing that both EDLC (non-Faradaic) and pseudocapacitance (Faradaic) take roles here.6,66 The CVs are not rectangular due to that some redox events take place in the potential range of 0–0.5 V. These redox reactions occur due to the presence of some functional groups in the carbons.6 Not only activated carbons but also CNTs and graphenes having functional groups (generated by oxidation with air, acid or by electro oxidation) exhibit this type of CV shape.66–68 Moreover, carbons having quinonic, phenolic, carboxylic, pyridinic (N-6) or pyrrolic (N-5) groups can undergo redox reactions and hence show peaks in their CVs.12,65,69,70 Since the area of the CV is directly proportional to specific capacitance (Cs) of an electrode material, the Cs of the carbons obtained at 900 °C should be in the following order; CNB900 > CNA900 > C900. For C samples obtained at <900 °C, the order of Cs should be C800 > C700 > C600. The same should be the order for CNB analogues. However, there is a high degree of decline in the area of the CV for C samples while moving to lower carbonization temperatures from 800 °C. Moreover, the areas of CV for C800, C700 and C600 samples are much lower than that of their CNB analogues.
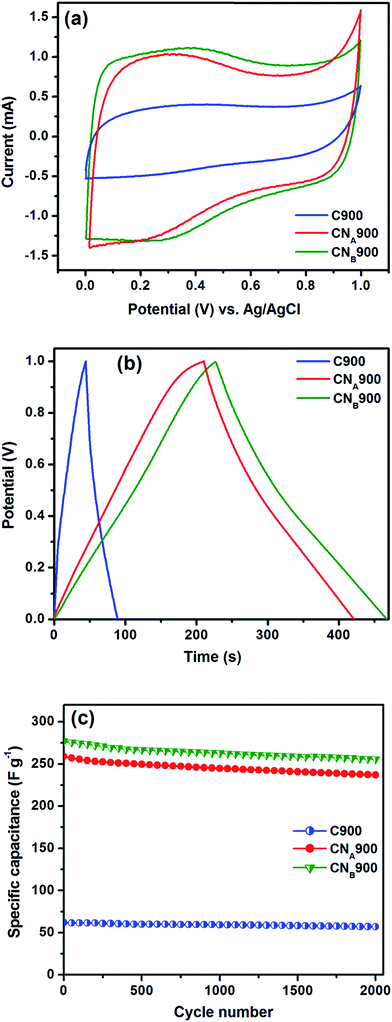 |
| Fig. 9 (a) Cyclic voltammograms of carbons, recorded in 1 M H2SO4 at the scan rate of 10 mV s−1, (b) galvanostatic charge–discharge curves collected with a current of 0.5 mA and (c) plot of variation of specific capacitance with cycle number of galvanostatic charge–discharge. | |
Charge–discharge curves of the carbon samples collected for estimating specific capacitance are shown in Fig. 9b, S1b and S2b.† The charge–discharge curves are linear and symmetrical, indicating the ideal capacitive characteristics. As for the plot, CNB900 and C900 take the longest and the shortest times respectively for charging and discharging, revealing that CNB900 and C900 hold the highest and the lowest charge-storage capacity among the three sets of carbons synthesized at 900 °C. As for the C and CNB samples made at 600–800 °C, the charging capacity is found to be higher for 800 °C analogues than their low temperature counterparts. Specific capacitance (Cs) values are calculated from galvanostatic discharge curves using the following relationship. Cs = IΔt/ΔVm, where, ‘I’ is the current, ‘Δt’ is discharge time of cycle, ‘ΔV’ is potential window and ‘m’ is mass of carbon.4 The Cs values of C900, CNA900 and CNB900 are 62, 259, and 277 F g−1 respectively. The order of the Cs for the carbons is as follows; CNB900 > CNA900 > C900. The trend observed among the Cs values of carbons is consistent with the trend of their surface areas. The observed higher Cs values of CNA900 and CNB900 are due to the positive effect of the precursors used. In order to ignore the effect of specific surface area (SSA) and understand the effect of other factors, ratio of Cs and SSA can be considered. The Cs/SSA values for C900, CNA900 and CNB900 are 0.30, 0.53 and 0.53 F m−2. The difference of 0.23 F m−2 observed between CNB900 and C900 may be due to the differences in conductivities, functional groups, wettability in electrolyte and diffusion barrier arising from the pore size.12,20,34,71
Activated carbon synthesized from sucrose with CO2 activation at 900 °C for 4 h and having a SSA of 2102 m2 g−1, has shown a Cs value of 165 F g−1 in 1 M sulphuric acid (Cs/SSA = 0.08).59 Activated microporous carbon nanofibers with a surface area of 597 m2 g−1 has shown a Cs value of 256 F g−1 in 6 M KOH electrolyte (Cs/SSA = 0.43).51 Activated carbon synthesized from sucrose-derived carbon spheres with a SSA of 2823 m2 g−1 has shown a Cs of 316 F g−1 in 6 M KOH electrolyte (Cs/SSA = 0.11).56 This implies that CNA and CNB carbons of the current work show superior performances even though they show lower SSAs. The Cs values and Cs/SSA ratio of the C600, C700 and C800 are 2, 3 and 35 F g−1 and 0.18, 0.19 and 0.25 F m−2 respectively. This reveals that Cs values decrease drastically while reducing the carbonization temperature from 800 °C to lower temperatures. This decrease might be due to the drastic reduction in SSA values. This proves the dependency of capacitance on surface area of the samples. It is worth to note that the Cs/SSA does not reduce considerably like Cs does. CNB600, CNB700 and CNB800 exhibit the Cs values of 18, 124 and 213 F g−1 and Cs/SSA of 0.38, 0.39 and 0.45 F m−2, respectively. As for the Cs value of CNB samples, it decreases with the decrease in the carbonization temperature. However, Cs/SSA value relatively stays as high as 0.38 F m−2 for CNB600 whereas it is only 0.18 F m−2 for C600. The poor Cs/SSA values shown by these C samples are probably due to their lower conductivities owing to the low degree of graphitic nature. This clearly indicates that the use of AN in the synthesis has a great role in the positive features of resulting carbons. Also, there is no considerable difference observed in the amount and nature of the observed the functional groups as per the XPS and CHN results, except for the presence of N functional groups (N-6 and N-Q) in CNB samples.
Regarding the effect of N content on the capacitance of carbon samples, the effect is not pronounced between CNA900 and CNB900. It is because their Cs/SSA values are the same (i.e., 0.53 F m−2) while having the N content of 2.3 and 3% respectively. Among all samples, the sum of N (%) and O (%) contents does not differ considerably. However, Cs/SSA values decrease with the reduction in the carbonization temperature. According to XPS analysis, CNB900 shows the presence of quinone, phenol and/or ether and chemisorbed oxygen (carboxylic acid) along with pyridinic (N-6) and quaternary (N-Q) nitrogen groups in it. Some reports reveal that besides quinone groups, phenolic, carboxylic, pyridinic (N-6) and pyrrolic (N-5) groups can undergo redox reactions to show pseudocapacitance.12,70,72 Moreover, phenolic, carboxylic and quaternary (N-Q) nitrogen groups can improve the electron transfer and wettability of the electrode.20,72 Thus, the presence of hetero atoms in the carbon improves the capacitance. However, it is realized that the high temperature carbonization is primarily important for enhanced capacitance of the carbons synthesized by the current method. In other words, the benefits of functional groups are realized only in the carbons of higher carbonization temperatures.
Stability of the capacitance of carbons obtained at 900 °C studied up to 2000 cycles using galvanostatic charge–discharge curves is given in Fig. 9c. Losses of capacitance of C900, CNA900 and CNB900 are 8.2, 9.1 and 7.6% respectively. The initial loss observed with the carbons might probably due to the loss of some amount of functional groups which are contributing to capacitance in terms of redox reactions. Further losses of capacitance observed might be due to peeling off of the electrode materials from current collector. The good stability shown by the carbon materials prove that they are promising ones for capacitor application.
4. Conclusions
Nitrogen- and oxygen-containing activated carbons have been synthesized using sucrose and ammonium nitrate. Use of ammonium nitrate has not only caused the nitrogen incorporation in carbon but also the formation of carbons with high surface areas. N-content of the carbons has increased with the increase in AN to sucrose ratio. One of the carbons obtained at 900 °C from sucrose and AN of 2
:
1 wt ratio retains 3 wt% of nitrogen in it. The carbons obtained using AN are found to be more graphitic and exhibit greater crystallite sizes (Lc) than the carbons from sucrose alone. The surface areas of carbons obtained using AN are as high as more than twice as that of carbons from sucrose alone. The steam activation employed here yields the microporous carbons. 13C NMR analysis proved that the carbons derived using AN show predominantly the carbons of aromatic nature whereas non-N-containing carbon shows the presence of aromatic carbons along with other functionalities including phenol and carbonyl carbons. Thermal stability of the N-containing carbons in air is found to be higher than non-N-containing carbons.
SEM and TEM results show that carbons particles synthesized are of size in the range of several nanometers to several micrometers with irregular shapes and they are proved to be polycrystalline in nature in complementary with XRD results. The presence of some graphene sheets also are identified along with 3D particles of carbon by TEM imaging. Electrochemical studies indicate the synthesized carbons show EDLC besides pseudocapacitance due to the presence of oxygen and nitrogen functional groups. One of the N- and O-containing carbons with the highest BET surface area of 518 m2 g−1 shows a specific capacitance of 277 F g−1. Reason for the higher Cs/SSA values shown by N- and O-containing carbons (900 °C) is believed to be due to higher surface areas and higher graphitic nature along with the presence of functional groups. Also, these carbons show excellent stability of capacitance over 2000 cycles. Thus, this synthetic method can be a promising one for the synthesis of N- and O-containing carbons and the carbons synthesized at higher temperature (i.e., 900 °C) can be potential candidates for supercapacitor application.
Acknowledgements
We acknowledge (Late) Prof. R. P. Viswanath and Prof. T. K. Varadarajan for their contributions to this work. The Department of Science and Technology (Government of India) is thankfully acknowledged for funding NCCR.
Notes and references
- Y. Zhai, Y. Dou, D. Zhao, P. F. Fulvio, R. T. Mayes and S. Dai, Adv. Mater., 2011, 23, 4828–4850 CrossRef CAS PubMed.
- J. Yan, Q. Wang, T. Wei and Z. Fan, Adv. Energy Mater., 2014, 4, 1300816 Search PubMed.
- P. Simon and Y. Gogotsi, Nat. Mater., 2008, 7, 845–854 CrossRef CAS PubMed.
- N. Subramanian, B. Viswanathan and T. K. Varadarajan, RSC Adv., 2014, 4, 33911–33922 RSC.
- M. Inagaki, H. Konno and O. Tanaike, J. Power Sources, 2010, 195, 7880–7903 CrossRef CAS PubMed.
- E. Frackowiak and F. Béguin, Carbon, 2001, 39, 937–950 CrossRef CAS.
- M. Sevilla and R. Mokaya, Energy Environ. Sci., 2014, 7, 1250–1280 CAS.
- A. G. Pandolfo and A. F. Hollenkamp, J. Power Sources, 2006, 157, 11–27 CrossRef CAS PubMed.
- L. Wei and G. Yushin, J. Power Sources, 2011, 196, 4072–4079 CrossRef CAS PubMed.
- A. S. Mestre, E. Tyszko, M. A. Andrade, M. Galhetas, C. Freire and A. P. Carvalho, RSC Adv., 2015, 5, 19696–19707 RSC.
- X. Y. Chen, C. Chen, Z. J. Zhang, D. H. Xie, X. Deng and J. W. Liu, J. Power Sources, 2013, 230, 50–58 CrossRef CAS PubMed.
- H. Liu, H. Song, X. Chen, S. Zhang, J. Zhou and Z. Ma, J. Power Sources, 2015, 285, 303–309 CrossRef CAS PubMed.
- Y. a. Huang, F. Yang, Z. Xu and J. Shen, J. Colloid Interface Sci., 2011, 363, 193–198 CrossRef CAS PubMed.
- G. Ćirić-Marjanović, I. Pašti, N. Gavrilov, A. Janošević and S. Mentus, Chem. Pap., 2013, 67, 781–813 Search PubMed.
- T. E. Rufford, D. Hulicova-Jurcakova, Z. Zhu and G. Q. Lu, Electrochem. Commun., 2008, 10, 1594–1597 CrossRef CAS PubMed.
- A. Bagreev, J. Angel Menendez, I. Dukhno, Y. Tarasenko and T. J. Bandosz, Carbon, 2004, 42, 469–476 CrossRef CAS PubMed.
- T. Horikawa, N. Sakao, T. Sekida, J. i. Hayashi, D. D. Do and M. Katoh, Carbon, 2012, 50, 1833–1842 CrossRef CAS PubMed.
- N. Kan-nari, S. Okamura, S.-i. Fujita, J.-i. Ozaki and M. Arai, Adv. Synth. Catal., 2010, 352, 1476–1484 CrossRef CAS PubMed.
- P. Pramanik, Bull. Mater. Sci., 1999, 22, 335–339 CrossRef CAS.
- D. Hulicova-Jurcakova, M. Seredych, G. Q. Lu and T. J. Bandosz, Adv. Funct. Mater., 2009, 19, 438–447 CrossRef CAS PubMed.
- K. Okajima, K. Ohta and M. Sudoh, Electrochim. Acta, 2005, 50, 2227–2231 CrossRef CAS PubMed.
- Z. R. Yue, W. Jiang, L. Wang, S. D. Gardner and C. U. Pittman Jr, Carbon, 1999, 37, 1785–1796 CrossRef CAS.
- S. Cagnina, P. Rotureau, G. Fayet and C. Adamo, Phys. Chem. Chem. Phys., 2013, 15, 10849–10858 RSC.
- L. Lai, H. Yang, L. Wang, B. K. Teh, J. Zhong, H. Chou, L. Chen, W. Chen, Z. Shen, R. S. Ruoff and J. Lin, ACS Nano, 2012, 6, 5941–5951 CrossRef CAS PubMed.
- C. R. M. Guiotoku, C. Maia and D. Hotza, in Microwave Heating, ed. U. Chandra, InTech, 2011, ch. 13, pp. 291–308 Search PubMed.
- Y.-G. Zhou, J.-J. Chen, F.-b. Wang, Z.-H. Sheng and X.-H. Xia, Chem. Commun., 2010, 46, 5951–5953 RSC.
- L. Muniandy, F. Adam, A. R. Mohamed and E.-P. Ng, Microporous Mesoporous Mater., 2014, 197, 316–323 CrossRef CAS PubMed.
- H. Takagi, K. Maruyama, N. Yoshizawa, Y. Yamada and Y. Sato, Fuel, 2004, 83, 2427–2433 CrossRef CAS PubMed.
- M. Darder and E. Ruiz-Hitzky, J. Mater. Chem., 2005, 15, 3913–3918 RSC.
- A. M. Vassallo and R. Codd, Carbon, 1988, 26, 553–558 CrossRef CAS.
- T. E. McGrath, W. G. Chan and M. R. Hajaligol, J. Anal. Appl. Pyrolysis, 2003, 66, 51–70 CrossRef CAS.
- A. B. Brizuela, L. C. Bichara, E. Romano, A. Yurquina, S. Locatelli and S. A. Brandán, Carbohydr. Res., 2012, 361, 212–218 CrossRef CAS PubMed.
- H. K. Sadhanala, J. Khatei and K. K. Nanda, RSC Adv., 2014, 4, 11481–11485 RSC.
- M. Seredych, D. Hulicova-Jurcakova, G. Q. Lu and T. J. Bandosz, Carbon, 2008, 46, 1475–1488 CrossRef CAS PubMed.
- Y. Guo and D. A. Rockstraw, Carbon, 2006, 44, 1464–1475 CrossRef CAS PubMed.
- J. Zawadzki, Carbon, 1995, 33, 1541–1546 CrossRef CAS.
- M.-W. Jung, K.-H. Ahn, Y. Lee, K.-P. Kim, J.-S. Rhee, J. T. Park and K.-J. Paeng, Microchem. J., 2001, 70, 123–131 CrossRef CAS.
- J. P. de Celis, M. S. Villaverde, A. L. Cukierman and N. E. Amadeo, Lat. Am. Appl. Res., 2009, 39, 165–171 CAS.
- M. Sevilla and A. B. Fuertes, Carbon, 2009, 47, 2281–2289 CrossRef CAS PubMed.
- S. Biniak, G. Szymanski, J. Siedlewski and A. Swiatkowski, Carbon, 1997, 35, 1799–1810 CrossRef CAS.
- Z. Yue, J. Economy and G. Bordson, J. Mater. Chem., 2006, 16, 1456–1461 RSC.
- A. M. Puziy, O. I. Poddubnaya, R. P. Socha, J. Gurgul and M. Wisniewski, Carbon, 2008, 46, 2113–2123 CrossRef CAS PubMed.
- P. Chen, L.-K. Wang, G. Wang, M.-R. Gao, J. Ge, W.-J. Yuan, Y.-H. Shen, A.-J. Xie and S.-H. Yu, Energy Environ. Sci., 2014, 7, 4095–4103 CAS.
- A. J. Plomp, D. S. Su, K. P. d. Jong and J. H. Bitter, J. Phys. Chem. C, 2009, 113, 9865–9869 CAS.
- D. Lennon, D. T. Lundie, S. D. Jackson, G. J. Kelly and S. F. Parker, Langmuir, 2002, 18, 4667–4673 CrossRef CAS.
- M. Kim, S. Hwang and J.-S. Yu, J. Mater. Chem., 2007, 17, 1656–1659 RSC.
- Y. Zhang, Z. Schnepp, J. Cao, S. Ouyang, Y. Li, J. Ye and S. Liu, Sci. Rep., 2013, 3, 2163 Search PubMed.
- T. Susi, T. Pichler and P. Ayala, Beilstein J. Nanotechnol., 2015, 6, 177–192 CrossRef CAS PubMed.
- K. László, E. Tombácz and K. Josepovits, Carbon, 2001, 39, 1217–1228 CrossRef.
- Y. J. Kim, Y. Abe, T. Yanagiura, K. C. Park, M. Shimizu, T. Iwazaki, S. Nakagawa, M. Endo and M. S. Dresselhaus, Carbon, 2007, 45, 2116–2125 CrossRef CAS PubMed.
- C. Ma, Y. Song, J. Shi, D. Zhang, X. Zhai, M. Zhong, Q. Guo and L. Liu, Carbon, 2013, 51, 290–300 CrossRef CAS PubMed.
- Z. Hu, M. P. Srinivasan and Y. Ni, Carbon, 2001, 39, 877–886 CrossRef CAS.
- X. He, H. Zhang, H. Zhang, X. Li, N. Xiao and J. Qiu, J. Mater. Chem. A, 2014, 2, 19633–19640 CAS.
- Y. Gao, Q. Yue and B. Gao, RSC Adv., 2015, 5, 31375–31383 RSC.
- C. Ruan, K. Ai and L. Lu, RSC Adv., 2014, 4, 30887–30895 RSC.
- L. Mao, Y. Zhang, Y. Hu, K. H. Ho, Q. Ke, H. Liu, Z. Hu, D. Zhao and J. Wang, RSC Adv., 2015, 5, 9307–9313 RSC.
- H. Liu, J. Zhang, H. H. Ngo, W. Guo, H. Wu, C. Cheng, Z. Guo and C. Zhang, RSC Adv., 2015, 5, 52048–52056 RSC.
- T. E. Rufford, E. Fiset, D. Hulicova-Jurcakova and Z. Zhu, in Green Carbon Materials: Advances and Applications, ed. T. E. Rufford, J. Zhu and D. Hulicova-Jurcakova, CRC press, Boca Raton, 2014, ch. 4, pp. 93–113 Search PubMed.
- L. Wei and G. Yushin, Carbon, 2011, 49, 4830–4838 CrossRef CAS PubMed.
- J. Kong, Q. Yue, B. Wang, L. Huang, B. Gao, Y. Wang and Q. Li, J. Anal. Appl. Pyrolysis, 2013, 104, 710–713 CrossRef CAS PubMed.
- T.-W. Kim, I.-S. Park and R. Ryoo, Angew. Chem., 2003, 115, 4511–4515 CrossRef PubMed.
- E. R. Buiel, A. E. George and J. R. Dahn, Carbon, 1999, 37, 1399–1407 CrossRef CAS.
- H. Marsh, M. Martínez-Escandell and F. Rodríguez-Reinoso, Carbon, 1999, 37, 363–390 CrossRef CAS.
- M. Sevilla and A. B. Fuertes, Chem.–Eur. J., 2009, 15, 4195–4203 CrossRef CAS PubMed.
- P. Adelhelm and P. E. de Jongh, J. Mater. Chem., 2011, 21, 2417–2427 RSC.
- R. Wang and X. Yan, Sci. Rep., 2014, 4, 3712 Search PubMed.
- H. Jiang, T. Zhao, C. Li and J. Ma, Chem. Commun., 2011, 47, 8590–8592 RSC.
- J. H. Chen, W. Z. Li, D. Z. Wang, S. X. Yang, J. G. Wen and Z. F. Ren, Carbon, 2002, 40, 1193–1197 CrossRef CAS.
- N. P. Wickramaratne, J. Xu, M. Wang, L. Zhu, L. Dai and M. Jaroniec, Chem. Mater., 2014, 26, 2820–2828 CrossRef CAS.
- Z. Zhou, Z. Zhang, H. Peng, Y. Qin, G. Li and K. Chen, RSC Adv., 2014, 4, 5524–5530 RSC.
- L. L. Zhang, Z. Lei, J. Zhang, X. Tian and X. S. Zhao, in Encycl. Inorg. Chem., John Wiley & Sons, Ltd, 2006 Search PubMed.
- C. Yuan, X. Liu, M. Jia, Z. Luo and J. Yao, J. Mater. Chem. A, 2015, 3, 3409–3415 CAS.
Footnote |
† Electronic supplementary information (ESI) available. See DOI: 10.1039/c5ra06661a |
|
This journal is © The Royal Society of Chemistry 2015 |