DOI:
10.1039/C5RA06268C
(Paper)
RSC Adv., 2015,
5, 44530-44544
Enzyme immobilization on silicate glass through simple adsorption of dendronized polymer–enzyme conjugates for localized enzymatic cascade reactions†
Received
8th April 2015
, Accepted 11th May 2015
First published on 11th May 2015
Abstract
A methacrylate based, water soluble, polycationic second generation dendronized polymer (denpol de-PG2) was used for the preparation of de-PG2–enzyme conjugates. Aspergillus sp. glucose oxidase (GOD) and horseradish peroxidase isoenzyme C (HRP) were covalently bound to the denpol, using a UV/vis traceable bis-aryl hydrazone (BAH) linker, to form two different de-PG2–BAH–enzyme hybrid structures, each carrying several copies of either GOD or HRP on a single denpol chain (on average ≈ 50 GOD or 108 HRP bound per denpol chain). In addition, a conjugate with several copies of both types of enzymes on the same polymer chain was synthesized (≈25 GOD and 78 HRP). These denpol–BAH–enzyme conjugates were found to be useful for the immobilization of the enzymes on unmodified silicate glass surfaces via simple adsorption of the conjugates from solution in one single step. The adsorbed conjugates strongly adhered to the glass surface due to multiple interactions between the conjugates and the surface. The conjugate adsorption was characterized with the transmission interferometric adsorption sensor (TInAS) and by AFM imaging, which showed formation of a homogenous thin layer of the conjugates. Additionally, the catalytic activity and stability of the immobilized enzymes were determined and the conjugates were used for the simple fabrication of enzymatic flow reactors for catalyzing a cascade reaction which involved both enzymes, either through a sequential immobilization of the two enzymes, or through a co-immobilization. In both cases, the two enzymes remained highly active during continuous operation at room temperature for at least several hours without any desorption of the enzymes from the surface. Overall, the methodology presented can be considered as a promising platform for a desired (co-)localization of active enzymes on solid supports.
1. Introduction
The immobilization of enzymes on solid surfaces has been studied for many years, following the pioneering work of Katchalski and others in the 1960s.1,2 Often, a covalent binding of the enzyme molecules to an organic or inorganic surface is achieved by using one of the different methodologies that have been developed over the years.3–16 One example is the covalent surface binding of enzymes through a reaction of nucleophilic free amino groups of surface exposed lysine residues of the enzymes with electrophilic centres on previously modified solid surfaces (e.g. an epoxide or an aldehyde); or by using appropriate bifunctional spacer molecules as molecular linkers between the surface and the enzymes to keep the enzymes at some distance from the surface.3 In these ways, enzymes can be grafted to silicates.5 Alternatively, enzyme immobilization through non-covalent adsorption on free or modified solid surfaces is also possible.3,6,17,18 One particular recent example is the non-covalent immobilization of enzymes on anionic supports via an adsorption of enzyme chimeras harbouring a polycationic polypeptide at the N-terminus of the enzyme produced in E. coli.19–21
Covalently or non-covalently immobilized enzymes are applied in various areas, ranging from the large scale synthesis of fine chemicals, food additives and pharmaceuticals10,20–25 to small scale biosynthesis and bioanalysis.26–34 For any novel enzyme immobilization method, the enzymes' catalytic efficiency, the operational stability of the immobilized enzymes and the ease of preparation are important to consider.16 In addition to the development of miniaturized devices based on immobilized enzymes, there is growing interest in the development of strategies for the co-immobilization of different types of enzymes for carrying out localized enzymatic cascade reactions involving different types of immobilized enzymes.4,5,7,8,16,17,23,26,29,33,35–40 The work about which we report here contributes to this field. We present a simple and efficient method for the non-covalent immobilization of different types of enzymes. The idea for the experiments carried out stems from two of our previous successful studies with dendronized polymers, as briefly outlined in the following.
In a first previous investigation we have demonstrated that enzymes can be immobilized for bioanalytical applications non-covalently on SiO2 surfaces with the help of the biotin–avidin system and a second generation dendronized polymer (denpol), abbreviated as de-PG2 (Fig. 1).41,42 This dendronized polymer contains a second generation dendron with four de-protected amino groups at the periphery on every repeating unit of the polymethacrylate polymer chain. Therefore, the denpol de-PG2 is polycationic and water soluble at pH values below about 8. The synthesis of analogous denpols – all with the same type of branching structure in the dendritic repeating unit – is possible up to at least the eighth generation (PG8).43–45 Compared to a conventional linear polymer, such as poly-L-lysine (PLL), denpols of the type de-PG1 to de-PG8 have increased chain thickness, chain stiffness, and surface charge density with increasing generation. De-PG2 (Fig. 1) strongly binds to SiO2 surfaces, even if it is partially biotinylated.41 This was demonstrated with de-PG2 molecules which contained on average about 1000 repeating units, de-PG21000.41 The strong binding probably is due to the many amino groups along the denpol chain (≈4000 amines per chain). Through an adsorption of the partially biotinylated de-PG21000 on glass surfaces, followed by addition of avidin and then biotinylated enzymes, the immobilization of horseradish peroxidase isoenzyme C (HRP), Aspergillus sp. glucose oxidase (GOD) and E. coli β-galactosidase (β-Gal) could be achieved and applied successfully, both within glass micropipettes42 and within microfluidic channels.46 The three different types of enzymes – β-Gal, GOD, and HRP – were immobilized spatially separated in a defined and desired sequence, first β-Gal, then GOD, and finally HRP, for allowing the quantitative determination of lactose on the basis of enzymatic cascade reactions involving the three enzymes.42,46 In that previous work, de-PG2 served as a kind of “glue”, a soft organic layer for keeping the enzyme on the surface (non-covalent adsorption). The biotin–avidin system was used as macromolecular linker unit for attaching the enzymes to the adsorbed denpol,47 whereby the denpol–enzyme conjugates were prepared in situ on a solid glass surface.
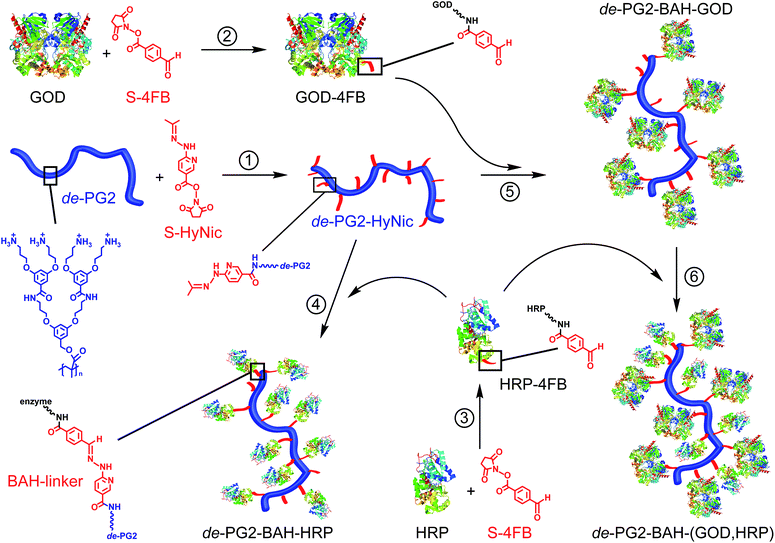 |
| Fig. 1 Chemical structure of the deprotected second generation denpol de-PG2 and schematic representation of the various steps involved for the synthesis of the denpol–BAH–enzyme conjugates prepared and used in this work. Step ➀: modification of de-PG2 with S-HyNic to yield de-PG2-HyNic. Step ➁: modification of GOD with S-4FB to yield GOD-4FB. Step ➂: modification of HRP with S-4FB to yield HRP-4FB. Step ➃: reaction of de-PG2-HyNic with HRP-4FB to yield de-PG2–BAH–HRP. Step ➄: reaction of de-PG2-HyNic with GOD-4FB to yield de-PG2–BAH–GOD. Step ➅: reaction of de-PG2–BAH–GOD with HRP-4FB to yield de-PG2–BAH–(GOD,HRP). See the Materials and methods for details. GOD: glucose oxidase; HRP: horseradish peroxidase; S-4FB, succinimidyl 4-formylbenzoate; S-HyNic, succinimidyl 6-hydrazinonicotinate acetone hydrazone; BAH, bis-aryl hydrazone. | |
In the second previous investigation, we have demonstrated that denpol–enzyme conjugates can be prepared in aqueous solution employing a covalent bis-aryl hydrazone (BAH) linkage instead of the biotin–avidin system.48,49 In that work, the denpols de-PG12000 and de-PG22000 were used and it could be shown that it is possible to attach not only one type of enzyme but also two different types of enzymes – bovine erythrocyte superoxide dismutase (SOD) and HRP – to one and the same denpol chain, whereby the two enzymes remained catalytically active.48,49
In the work which we present here, we combined the two previous approaches: denpol–BAH–enzyme conjugates were first prepared in solution, followed by their adsorption on a solid support. This allowed the easy co-immobilization of two different types of enzymes on glass surfaces. The two enzymes used were HRP and GOD. Both enzymes (i) are well suited for biosensor and enzymatic fuel cell applications,50–52 (ii) catalyze two sequential steps of an enzymatic cascade reaction, and (iii) are commercially available in high purity and at an affordable price. The denpol applied was again de-PG2 (Fig. 1), this time with a number average degree of polymerization of 1400, i.e. de-PG21400. Compared to the denpol-mediated enzyme immobilization via the biotin–avidin system developed previously,41,42,46 our novel approach with the denpol–BAH–enzyme conjugates is simpler and the two enzymes can be immobilized not only spatially separated, but also in close proximity to each other at a defined ratio of the two enzymes (through an adsorption of conjugates containing both enzymes). To the best of our knowledge, this is the first time that such type of co-immobilization under retention of the enzymes' activities could be achieved. This approach is promising for the straightforward fabrication of devices which are based on the spatially controlled localization of different types of enzymes at a desired ratio, for example in microreactors.26
2. Materials and methods
2.1 Materials
Aspergillus sp. glucose oxidase (GOD, product GLO-2022, lot 9448520002, 242 U mg−1, EC 1.1.3.4, M ≈ 153 kDa) and horseradish peroxidase isoenzyme C (HRP, product PEO-131, grade I, lot 2131616000, 278 U mg−1, EC 1.11.1.7, M ≈44 kDa) were from Toyobo Enzymes.53 De-PG21400 (Pn ≈ 1400, PDI ≈ 4.7) was synthesized by Dr Baozhong Zhang, following a procedure described previously.43 Silicate microscopy glass coverslips were obtained from Science Services, Germany (round coverslips, 8 mm diameter) and Knittel Gläser (rectangular coverslips, cut to 12.5 × 8 mm). Glass micropipettes (intraMARK 200 μL) were purchased from Brand, Germany. Succinimidyl 6-hydrazinonicotinate acetone hydrazone (S-HyNic) and succinimidyl 4-formylbenzoate (S-4FB) were synthesized by Dr Andrea Grotzky as described earlier.48,54 2,2′-Azino-bis(3-ethylbenzothiazoline-6-sulfonic acid) diammonium salt (ABTS2−), o-phenylenediamine (OPD), D-glucose, and sodium dihydrogenphosphate were purchased from Sigma-Aldrich (Switzerland). Sodium chloride and hydrogen peroxide were obtained from Merck (Switzerland) and 3-(N-morpholino)propanesulfonic acid (MOPS) and 2-(N-morpholino)ethanesulfonic acid (MES) were purchased from AppliChem (Germany).
2.2 Buffer solutions
All buffer solutions were prepared with Milli-Q water at room temperature and the pH was controlled with a pH-meter (Mettler-Toledo FE20). All reactions were carried out at room temperature (T ≈ 25 °C). Buffer-1: 100 mM MOPS, 150 mM NaCl, pH 7.6. Buffer-2: 100 mM MES, 150 mM NaCl, pH 4.7. Buffer-3: 10 mM NaH2PO4, 150 mM NaCl, pH 7.2. Buffer-4: 10 mM NaH2PO4, 150 mM NaCl, pH 5.0. Buffer-5: 100 mM MES, 1.15 M NaCl, pH 4.7. Buffer-6: 10 mM NaH2PO4, 150 mM NaCl, pH 7.0.
2.3 UV/vis-spectrophotometers
UV/vis-absorption measurements were carried out with a JASCO V-670 spectrophotometer from Jasco Inc. (Japan), or a Specord S 600 diode array spectrophotometer from Analytik Jena (Germany), using either quartz or polystyrene cuvettes. The quartz cuvettes were pretreated with de-PG2 solutions to minimize adsorption of the conjugates. For the determination of the activity of the enzymes immobilized inside glass micropipettes, a Z-flow cell (from Ocean Optics) was connected to the outlet of the micropipette and a buffered assay solution was pumped through the system. The absorption spectrum of the solution inside the flow-cell was measured with an Ocean Optics USB2000+ spectrometer, connected to the cell with a 400 μm optical fiber, all purchased from GMP SA, Renens, Switzerland.
2.4 Preparation of de-PG2-HyNic ➀ (Fig. 1)
Modification of de-PG2 with succinimidyl 6-hydrazinonicotinate acetone hydrazone (S-HyNic) was performed as described earlier.48 De-PG2 was dissolved in buffer-1 at a concentration of 2 mg mL−1. The repeating unit concentration was determined by UV/vis-spectrophotometry at 285 nm (ε285 nm = 5000 M−1 cm−1).41 0.4 equivalents S-HyNic per repeating unit were added from a 20 mM stock solution prepared in dry DMF. After 4 h at room temperature, the reaction was stopped by addition of an equal volume of buffer-2 and hydrolyzed S-HyNic was removed by repetitive ultrafiltration with buffer-2 in an Amicon Ultra-0.5 mL ultrafiltration device, 50 kDa MWCO (Millipore, Switzerland). The HyNic concentration in the retentate was determined by derivatization with 4-nitrobenzaldehyde54 and the repeating unit concentration was determined with the trypan blue assay.48
2.5 Preparation of GOD-4FB ➁ and HRP-4FB ➂ (Fig. 1)
GOD was dissolved at a concentration of 20 mg mL−1 in buffer-3 and the stabilizers contained in the enzyme preparation were removed by repetitive ultrafiltration with buffer-3 in an Amicon Ultra-0.5 mL ultrafiltration device, 50 kDa MWCO. The concentration of purified GOD was determined by UV/vis-spectrophotometry at 450 nm (ε450 nm = 28
200 M−1 cm−1).55 To a 50 μM solution of GOD 1.2 equivalents of succinimidyl 4-formylbenzoate (S-4FB) were added from a 5 mM stock solution in dry DMF. After 4 h at room temperature, hydrolyzed S-4FB was removed by repetitive ultrafiltration with buffer-2 in a 50 kDa MWCO ultrafiltration device. The 4FB concentration was determined by derivatization with 2-hydrazinopyridine as described by Solulink,54 with slight modifications. 5 μL GOD-4FB as obtained from ultrafiltration were added to 995 μL of 500 μM 2-hydrazinopyridine dihydrochloride in buffer-2 and the changes in the UV/vis spectra were monitored for 6 h with a spectrophotometer. The absorbance at 450 nm allows determination of the GOD concentration, while the increasing signal at 350 nm allows quantification of the 4FB linker (ε350 nm = 24
500 M−1 cm−1). Modification of HRP with a 4FB-linker was performed as described earlier.48
2.6 Preparation of de-PG2–BAH–HRP ➃ (Fig. 1)
The synthesis of de-PG2–BAH–HRP ➃ (Fig. 1) was performed as described before,48 with slight modifications. De-PG2-HyNic and HRP-4FB were mixed in buffer-2 containing 10 mM aniline at a HyNic concentration of 75 μM and a 4FB concentration of 50 μM. The aniline acts as a nucleophilic catalyst in the bis-aryl hydrazone formation as described by Dirksen and Dawson.56 The reaction was monitored in situ by UV/vis-spectrophotometry (see Fig. S1, ESI†), and after 3.5 h the excess enzyme was removed by repetitive ultrafiltration with buffer-4 on a Amicon Ultra-4 ultrafiltration device, 100 kDa MWCO.
2.7 Preparation of de-PG2–BAH–GOD ➄ (Fig. 1)
De-PG2-HyNic and GOD-4FB were mixed in buffer-5 at a concentration of 75 μM HyNic and 50 μM 4FB. The BAH formation was monitored by UV/vis-spectrophotometry, see Fig. S2 (ESI†). After 16 h, de-PG2–BAH–GOD was precipitated four times from 60% saturated ammonium sulfate by addition of 1.5 times the sample volume of saturated ammonium sulfate solution, followed by incubation for 15 min, centrifugation for 20 min at 16
000 × g, removal of the supernatant and re-dissolving the pellet in buffer-4. Under these conditions, free GOD and GOD-4FB remain in solution and are removed in the supernatant. Remaining ammonium sulfate was removed from the de-PG2–BAH–GOD solution by repetitive ultrafiltration with buffer-4 in a Centrisart I 300 kDa MWCO ultrafiltration device (Sartorius Stedim, Switzerland).
2.8 Preparation of de-PG2–BAH–(GOD,HRP) ➅ (Fig. 1)
The two-enzyme conjugate was synthesized in a sequential reaction of de-PG2-HyNic with GOD-4FB in a first step and HRP-4FB in a second step. De-PG2-HyNic and GOD-4FB were mixed in buffer-5 at a concentration of 100 μM HyNic and 50 μM 4FB. The reaction was monitored by UV/vis spectrophotometry for about 16 h by carrying out the reaction inside a quartz cuvette with a path length of 0.1 cm, Fig. 2. After this time, further increase in absorbance at 354 nm due to the formation of the BAH bond was negligible. Then, HRP-4FB was added to the reaction mixture to a concentration of 50 μM 4FB. The HyNic concentration after this dilution was about 75 μM. Again, the reaction of de-PG2–BAH–GOD with HRP-4FB could be monitored by UV/vis spectrophotometry for 16 h, after which time the increase in absorbance at 354 nm was negligible.57 The de-PG2–BAH–(GOD,HRP) conjugate was purified by precipitation from 50% saturated ammonium sulfate by addition of a volume of saturated ammonium sulfate solution corresponding to the reaction volume recovered from the UV/vis cuvette. The precipitation was performed 4 times with 15 min incubation after addition of the ammonium sulfate, 20 min centrifugation at 16
000 × g and re-dissolving the pellet in buffer-4. Under these conditions, free GOD, GOD-4FB, HRP and HRP-4FB remained in solution and could be removed from the de-PG2–BAH–(GOD,HRP) conjugate effectively. Remaining ammonium sulfate was removed from the solution by repetitive ultrafiltration with buffer-4 using a Centrisart I 300 kDa MWCO ultrafiltration device (Sartorius Stedim, Switzerland).
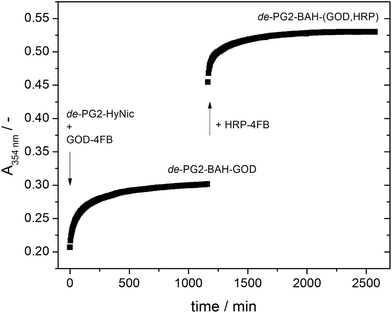 |
| Fig. 2 Continuous monitoring of the formation of de-PG2–BAH–(GOD,HRP) at pH = 4.7 and T = 25 °C by UV/vis spectrophotometry, recording changes in the absorbance at λ = 354 nm (A354 nm) originating from the formation of the BAH bond and an increase in turbidity (see text for details). In a first step, the formation of de-PG2–BAH–GOD occurs upon mixing solutions of de-PG2-HyNic and GOD-4FB (up to about 1250 min ≈ 21 h). Afterwards, a further increase in A354 nm occurs upon addition of a solution of HRP-4FB to yield de-PG2–BAH–(GOD,HRP), followed up to about 2600 min (≈43 h). Path length = 0.1 cm. | |
2.9 AFM analysis
For the analysis of single chains of denpol and denpol–BAH–enzyme conjugates, a 20 μL aliquot of each solution (20 μM repeating unit concentration for de-PG2, about 2 μM repeating unit concentration for the denpol–BAH–enzyme conjugates, respectively) was deposited onto freshly cleaved mica, incubated for 2 minutes, rinsed with Milli-Q water and dried by air. For the analysis of the adsorbed denpol and denpol–BAH–enzyme conjugates on glass, the microscopy glass coverslips were prepared as for the enzymatic activity measurements (see below) and mounted on metallic support for AFM measurements. AFM measurements were performed by using a MultiMode VIII Scanning Probe Microscope (Bruker, USA) operated in intermittent mode under ambient conditions. The microscope was covered with an acoustic hood to minimize vibrational noise. AFM cantilevers (Bruker, USA) for tapping mode in soft tapping conditions were used at a vibrating frequency of 150 kHz. Images were simply flattened using the Nanoscope 8.1 software, and no further image processing was carried out.
2.10 TInAS measurements
The adsorption of the denpol–BAH–enzyme conjugates on SiO2 coated sensor chips was monitored with a transmission interferometric adsorption sensor (TInAS).58,59 The SiO2 sensor surface was cleaned with toluene and 2-propanol (3 times each for 10 minutes in a sonication bath) and subsequent oxygen plasma cleaning for two minutes. After assembly of the flow cell, the sensors were equilibrated with buffer-4 and mounted on the TInAS instrument. All measurements were performed with a constant flow rate of 20 μL min−1 at room temperature. During solution changes, the flow rate was increased to 0.4 mL min−1 to assure a rapid exchange of the solution inside the flow cell and to minimize diffusion effects. For the adsorption of the denpol de-PG2 a solution at a concentration of 20 μM (repeating unit concentration) in buffer-4 was used. For the adsorption of the denpol–BAH–enzyme conjugates, a solution containing the conjugate at a concentration of about 2 μM (repeating unit concentration) was used. The evaluation of the adsorption was performed according to Sannomiya et al.59 using the refractive index increment of dn/dc = 0.140 cm3 g−1 for de-PG2,41 and a calculated refractive index increment for the three different denpol–BAH–enzyme conjugates, as determined in the following way: the molecular weight of HRP was taken as 44 kDa, containing an average of 20 mass% of glycosylation,60 and for GOD, a molecular weight of 153 kDa as specified by the supplier53 and a carbohydrate content of 16.2 mass% (ref. 61) was considered for the calculations. The values for dn/dc for the different conjugates were calculated as a linear combination of the values of the single components, denpol, protein and protein-glycosylation, taking into account their contribution to the total mass of the conjugates and using a value of dn/dc = 0.186 cm3 g−1 for the protein and dn/dc = 0.136 cm3 g−1 for the glycosylation.62 The calculated values for the prepared conjugates (see below) were dn/dc = 0.170 cm3 g−1 for de-PG21400–BAH–HRP108, dn/dc = 0.174 cm3 g−1 for de-PG21400–BAH–GOD50, and dn/dc = 0.173 cm3 g−1 for de-PG21400–BAH–(GOD25,HRP78).
2.11 Adsorption of denpol–BAH–enzyme conjugates
2.11.1 Adsorption onto microscopy glass coverslips. Round coverslips (8 mm diameter, total surface 1 cm2) and rectangular coverslips (1.25 cm × 0.8 cm, total surface 2 cm2) were cleaned by bath sonication in ethanol (3 times 10 min; Bandelin Sonorex RK 100H) and dried with nitrogen. After wetting of the coverslips with buffer-4, a diluted solution of the denpol–BAH–enzyme conjugate was added (about 2 μM repeating unit concentration), as established for the TInAS measurements. After 1 h at room temperature, the denpol–BAH–enzyme solution was removed and the coverslips were washed 3 times with fresh buffer-4 and 3 times with buffer-6. Between the activity measurements for the determination of the storage stability, the coverslips were kept in buffer-6 in closed 2 mL polypropylene reaction tubes at 4 °C.
2.11.2 Adsorption inside glass micropipettes. The glass micropipettes were cleaned 3 times by sonication in ethanol for 10 min and dried with nitrogen. After wetting the inner surface with buffer-4, a diluted solution of the denpol–BAH–enzyme conjugate (about 2 μM repeating unit concentration) was filled into the micropipette for adsorption on the inner glass surface. After 1 h at room temperature, the solution was removed and the micropipette was washed 3 times with fresh buffer-4 and 3 times with buffer-6. For storage, the micropipettes were filled with buffer-6 and kept at 4 °C.
2.12 Enzyme activity measurements
The activities of the two enzymes, HRP and GOD, were determined at pH = 7.0 by applying two established assays, as outlined in detail below. For HRP, the substrates were ABTS2− and hydrogen peroxide (H2O2) and the formation of the ABTS˙− radical was followed spectrophotometrically at λ = 414 nm (ε414 nm = 36
000 M−1 cm−1).48,63 The activity of GOD was measured with a coupled assay with HRP and its substrate OPD by using D-glucose and dissolved dioxygen (O2) as substrates for GOD.33 In brief, the oxidation of D-glucose by GOD/O2 yields glucono-δ-lactone and H2O2 as products; the formed H2O2 – in presence of excess HRP – leads to a quantitative oxidation of OPD to 2,3-diaminophenazine (DAP) which can be followed spectrophotometrically at λ = 418 nm (ε418 nm = 16
700 M−1 cm−1).64 For an estimation of the amount of glass surface-adsorbed active GOD and HRP ({GOD}apparent and {HRP}apparent, respectively, expressed in pmol cm−2), the determined activities of the adsorbed enzymes were compared with the activities of known amounts of enzymes measured in bulk solution under the same conditions, see ESI, Fig. S3 and S4.†
2.12.1 Activity of denpol–BAH–enzyme conjugates adsorbed on microscopy glass coverslips. For measuring the activity of HRP, the storage buffer (buffer-6) was removed from the polypropylene reaction tubes containing the glass coverslips and the coverslips were washed with fresh buffer-6. After removal of the washing buffer, a substrate solution containing 1 mM ABTS2− and 200 μM H2O2 in buffer-6 was added to the reaction tubes. After a defined reaction time, typically 1 min, the assay solution was removed from the reaction tubes and the coverslips were washed with fresh buffer-6. The assay solution was immediately analyzed by UV/vis spectrophotometry using 1 cm polystyrene cuvettes, and the ABTS˙− radical formation was determined from the increase in absorbance at λ = 414 nm (ΔA414 nm). The obtained ΔA414 nm/time values were correlated with ΔA414 nm/time values obtained under the same conditions of substrate concentrations and pH with known amounts of HRP, see the calibration curve in Fig. S3 (ESI†).For measuring the GOD activity, the storage buffer (buffer-6) was removed and the coverslips were washed with fresh buffer-6. After removal of the washing buffer, an assay solution containing 3.45 mM D-glucose, 3.14 mM OPD,65 and 2 nM HRP in buffer-6 was added to the reaction tubes containing the coverslips. The reaction tubes were incubated at room temperature and protected from light and after a defined reaction time, typically 20 min, the assay solution was removed and the coverslips were washed with buffer-6. The assay solution was analyzed by UV/vis spectrophotometry immediately after removal using 1 cm polystyrene cuvettes, and the formation of DAP was determined from the increase in absorbance at λ = 418 nm (ΔA418 nm). The obtained ΔA418 nm/time values were correlated with ΔA418 nm/time values obtained under the same conditions of substrate concentrations and pH with known amounts of GOD, see the calibration curve in Fig. S4 (ESI†).
For measuring the cascade activity of co-immobilized GOD and HRP, a similar assay as for measuring the GOD activity was applied, except that the assay solution did not contain any additional HRP, i.e. a solution of 3.45 mM D-glucose and 3.14 mM OPD65 in buffer-6 was used.
2.12.2 Activity and stability of denpol–BAH–enzyme conjugates adsorbed inside glass micropipettes. The activity and stability of GOD and HRP during continuous substrate conversion was monitored by using a continuous flow system connected to UV/vis detection. For the sequential enzymatic cascade, a glass micropipette with adsorbed de-PG2–BAH–GOD was connected with a short silicone tubing to a micropipette with adsorbed de-PG2–BAH–HRP. A substrate solution containing 3.45 mM D-glucose and 0.25 mM ABTS2− in buffer-6 was supplied from a reservoir via PTFE tubing and the product formation was monitored online using a UV/vis spectrophotometer connected to a flow cell. The flow rate was controlled using a peristaltic pump P-1 from Pharmacia-LKB. For the analysis of the co-immobilized GOD and HRP, a single glass micropipette with adsorbed de-PG2–BAH–(GOD,HRP) was installed in the same setup.
3. Results and discussion
3.1 Preparation of denpol–BAH–enzyme conjugates
The general procedures which we used for the stepwise synthesis of the denpol–BAH–enzyme conjugates are summarized in Fig. 1. The deprotected denpol de-PG2 was modified first in solution at pH = 7.6 through a reaction of some of the amino groups of de-PG2 with S-HyNic (succinimidyl 6-hydrazinonicotinate acetone hydrazone), yielding de-PG2-HyNic (reaction ➀ in Fig. 1). GOD and HRP were modified separately in solution at pH = 7.2 with S-4FB (succinimidyl 4-formylbenzoate) through a reaction of amino groups of lysine side chains which are localized on the surface of the two enzymes, yielding GOD-4FB (reaction ➁ in Fig. 1) and HRP-4FB (reaction ➂ in Fig. 1). The experimental conditions were chosen such that most of the modified enzyme molecules contained one 4FB unit. Three types of denpol–BAH–enzyme conjugates were then prepared in solution at pH = 4.7: de-PG2–BAH–HRP (through a reaction of dissolved de-PG2-HyNic with dissolved HRP-4FB, reaction ➃ in Fig. 1), de-PG2–BAH–GOD (through a reaction of de-PG2-HyNic with GOD-4FB, reaction ➄ in Fig. 1); and de-PG2–BAH–(GOD,HRP) (through a reaction of de-PG2–BAH–GOD with HRP-4FB, reaction ➅ in Fig. 1). For experimental details, see Materials and methods.
The extent of modification of de-PG2 with S-HyNic (reaction ➀ in Fig. 1) and the extent of modification of GOD and HRP with S-4FB (reactions ➁ and ➂ in Fig. 1) were determined by quantifying spectrophotometrically the amount of denpol bound HyNic with 4-nitrobenzaldehyde and the amount of 4FB bound to the enzymes with 2-hydrazinopyridine (see Materials and methods). The determined molar substitution ratio (MSR)48 for HyNic in de-PG2, MSR(HyNic)de-PG2 was 0.16 (given per repeating unit, r.u.),48 the MSR for 4FB in GOD, MSR(4FB)GOD, was 0.65, and MSR(4FB)HRP = 0.85. This means that the de-PG2-HyNic we prepared for this work had about every 6th r.u. one HyNic. Since each r.u. carries four peripheral amino groups, the molar ratio of HyNic to amino groups was 1/25 = 0.04, i.e. about 4% of all amino groups of de-PG2 were modified with HyNic. These HyNic groups had the potential to react with the 4FB groups bound to GOD or HRP, whereby MSR(4FB)enzyme < 1 indicates that most modified enzymes had one 4FB unit.48,66 Unmodified enzymes did not react with de-PG2-HyNic and were removed during work-up (see Materials and methods).
Since the formation of the bis-aryl hydrazone (BAH) bond at pH = 4.7 is accompanied by an increase in light absorption at λ ≈ 350 nm,54,66 the conjugation of the 4FB-modified enzymes to the HyNic-modified denpol can be analyzed spectrophotometrically. If the concentrations of the reacting denpol and enzymes are chosen appropriately, the BAH bond formation can be monitored in real time by performing the conjugation reaction within a quartz cuvette inside a UV/vis spectrometer.48,49,66 Fig. 2 shows the change in absorbance at λ = 354 nm, A354 nm, as a function of time after mixing solutions of the modified denpol and the modified enzymes at pH = 4.7 and T = 25 °C. The tracing on the left hand side of Fig. 2 reflects the formation of de-PG2–BAH–GOD as it occurred after mixing aqueous solutions of de-PG2-HyNic and GOD-4FB, while the tracing on the right hand side illustrates the succeeding formation of de-PG2–BAH–(GOD,HRP), i.e. the changes in A354 nm, occurring after adding an aqueous solution of HRP-4FB to the de-PG2–BAH–GOD solution. For both steps in Fig. 2, the time required for reaching “equilibrium”, i.e. reaching conditions where only a slow increase in A354 nm with time occurred, was about 16–17 h. The reaction of de-PG2–BAH–GOD with HRP-4FB was possible (i) since de-PG2–BAH–GOD still contained reactive HyNic units, and (ii) since the benzaldehyde moiety of HRP-4FB could reach these HyNic units along the de-PG2–BAH–GOD chain. In this way a denpol–BAH–enzyme conjugate could be prepared which contained both enzymes, GOD as well as HRP, similarly to what we achieved previously in the case of denpols containing SOD and HRP.48,49
Finally, the conjugate de-PG2–BAH–HRP was prepared by reacting de-PG2-HyNic with HRP-4FB, in the same way as described previously.48,49 BAH bond formation was again monitored by measuring the changes in A354 nm with time after mixing solutions of de-PG2-HyNic and HRP-4FB, see Fig. S1 (ESI†).
All three denpol–BAH–enzyme conjugates were purified, i.e. separated from free enzymes, as described in Materials and methods.
3.2 Characterization of the denpol–BAH–enzyme conjugates
3.2.1 Estimation of amount of enzyme bound to the denpol. The advantage of the BAH bond over other linkers is its quantifiability through simple UV/vis absorption measurements, see Fig. 2, S1 and S2 (ESI†).48,49,54,66 With the known molar extinction coefficient at λ = 354 nm (ε354 nm = 29
000 M−1 cm−1)54 the MSR values for de-PG2-HyNic, GOD-4FB and HRP-4FB could be determined, see Materials and methods. In the same way the BAH concentration in solutions of de-PG2–BAH–HRP could also be quantified.48,49 Typically, de-PG2-HyNic at a HyNic concentration of 75 μM was reacted at pH = 4.7 with HRP-4FB at a 4FB concentration of 50 μM (reaction ➃ in Fig. 1) yielding de-PG2–BAH–HRP with a BAH concentration of 36 μM. This means that de-PG2–BAH–HRP contained on average every 13 r.u. one HRP molecule. For a denpol with 1400 r.u. 108 HRP molecules were bound along the denpol chain. Since MSR(HyNic)de-PG2 = 0.16 (corresponding to 0.16 × 1400 = 224 HyNic per 1400 r.u. long denpol), there were still 116 (=224–108) unreacted HyNic in this 1400 r.u. long denpol, as well as 5376 free amines.67For de-PG2–BAH–GOD, a precise determination of the amount of BAH is hindered by the concomitant appearance of turbidity during the formation of de-PG2–BAH–GOD (reaction ➄ in Fig. 1). This denpol–BAH–enzyme conjugate becomes so large during conjugate formation that it leads to a scattering of light, as can easily be seen between λ = 850 and 550 nm where there should be no light absorption caused by the denpol, the enzyme, or the BAH linker, see Fig. S2 (ESI†). Therefore, the measured changes in “A354 nm” during the conjugate formation with GOD are due to BAH bond formation as well as due to an increase in turbidity. Similar problems were encountered in the synthesis of de-PG2–BAH–(GOD,HRP) (reaction ➅ in Fig. 1). For this reason, the amount of BAH bond formed in these two GOD-containing conjugates could only be estimated roughly. With the measured increase in “A354 nm”, the maximum amount of BAH-bonds in the GOD-containing conjugates was calculated as upper limit in the same way as described for de-PG2–BAH–HRP, taking into account the measured ΔA354 nm (Fig. 2). The value obtained for a denpol with 1400 r.u is 149 GOD molecules per denpol chain for de-PG2–BAH–GOD and 75 GOD molecules in the case of de-PG2–BAH–(GOD,HRP) which contained 78 HRP molecules. These values for GOD clearly are overestimations. The true values are probably about 30–50% of this maximal value.
3.2.2 Activity of the denpol–BAH–enzyme conjugates in solution. The enzymatic activities of dissolved de-PG2–BAH–HRP, de-PG2–BAH–GOD, and de-PG2–BAH–(GOD,HRP) were measured (i) with ABTS2− and H2O2 (for the activity of HRP), (ii) with D-glucose, free HRP and OPD (for the activity of GOD), and (iii) with D-glucose and OPD (for the combined activities of GOD and HRP), see Materials and methods. Both enzymes were still active when bound to de-PG2 in all three conjugates prepared. Detailed activity measurements of HRP were already carried out previously with de-PG22000–BAH–HRP.49 The new determinations of the activity of HRP bound to de-PG1400 are in good agreement with these earlier measurements, although two different batches of de-PG2 were used, de-PG22000 (previously)49 and de-PG21400 (this work).For GOD, the conjugates prepared in this work are novel. Since we encountered difficulties in precisely determining the BAH content in the conjugates (see above), a quantification of the percentage of active GOD in the two conjugates, de-PG2–BAH–GOD and de-PG2–BAH–(GOD,HRP) was difficult and could only roughly be estimated by taking into account ΔA354 nm and the contribution of turbidity, as described above.68
As a result, and expressed with the abbreviations used previously,48,49 the three denpol–BAH–enzyme conjugates prepared in this work were de-PG21400–BAH–HRP108, de-PG21400–BAH–GOD≈50,68 and de-PG21400–BAH–(GOD≈25,HRP78),68 indicating that a denpol chain of 1400 r.u. contained about 108 HRP molecules in the case of de-PG2–BAH–HRP, about 50 GOD molecules in the case de-PG2–BAH–GOD, and about 78 HRP and 25 GOD molecules in the case of de-PG2–BAH–(GOD,HRP).
3.2.3 Visualization of the denpol–BAH–enzyme conjugates by AFM. The denpol–BAH–enzyme conjugates and free denpol (de-PG21400) were deposited from aqueous solutions onto freshly cleaved mica and visualized in the dry state by AFM. Representative 3D AFM images with corresponding longitudinal height profiles are display in Fig. 3; for de-PG21400 (Fig. 3a), for de-PG21400–BAH–HRP108 (Fig. 3b), for de-PG21400–BAH–GOD≈50 (Fig. 3c) and for de-PG21400–BAH–(GOD≈25,HRP78) (Fig. 3d). Similarly to our previous visualization of de-PG12000–BAH–(HRP120,SOD60) and de-PG22000–BAH–(HRP120,SOD60),49 the bound enzymes are distributed along the denpol chain. Very rarely, segments of denpol without enzymes can be found. The height of the free denpol is similar to previously reported results49 and the “longitudinal profile” (height profile traced along the adsorbed denpol chain) has values around 1.5 nm. The longitudinal height profiles for the denpol–BAH–enzyme conjugates have higher values compared to the free denpol confirming the sufficient binding of the enzymes to the denpol. Furthermore, there are no free enzymes, indicating that enzyme adsorption only occurs if the enzyme is denpol-bound. The differences in the height profiles for de-PG2 (Fig. 3a, around 1.5 nm in height), de-PG21400–BAH–HRP108 (Fig. 3b, between about 1.5 and 5.5 nm in height) and de-PG21400–BAH–GOD≈50 (Fig. 3c, between about 4 and 16 nm in height) correlate with the expected sizes of the conjugates considering the size of the enzymes attached to the denpol (M(HRP) ≈ 44 kDa, M(GOD) ≈ 153 kDa (ref. 53)). In the case of de-PG21400–BAH–(GOD≈25,HRP78), the height profile is more heterogeneous with more pronounced variations along the chain than for the other conjugates, but a clear identification of the two different types of enzymes is not possible.
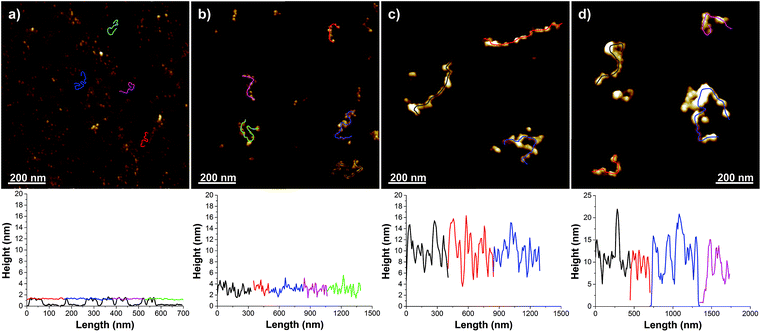 |
| Fig. 3 Tapping mode 3D AFM images of de-PG2 (a), de-PG2–BAH–HRP (b), de-PG2–BAH–GOD (c), and de-PG2–BAH–(GOD,HRP) (d) on mica with corresponding longitudinal height profiles along individual chains given as indicated with differently coloured lines (black, red, blue, purple, and green). The height variations indicated in black in the graph (a) correspond to the transversal height profile along the straight black line in the AFM image. | |
3.3 Immobilization of the denpol–BAH–enzyme conjugates
3.3.1 Overview. The denpol–BAH–enzyme conjugates were immobilized on SiO2 surfaces by simple adsorption from an aqueous solution. The adsorption process was first investigated with a transmission interferometric adsorption sensor (TInAS) on sensor chips which were coated with SiO2 particles (sputtered SiO2), see Materials and methods. Afterwards, the optimal conditions found with these TInAS measurements were applied for the adsorption of the conjugates on microscopy glass coverslips and on the inner wall of glass micropipettes. In both cases, the activity and stability of the enzymes were measured. Apart from the activity of both enzymes, GOD and HRP, a two-enzyme cascade reaction involving both immobilized enzymes was investigated as well: the oxidation of D-glucose to glucono-δ-lactone and H2O2, catalyzed by GOD with dissolved O2 as oxidant, followed by the oxidation of ABTS2− to ABTS˙− with the formed H2O2, catalyzed by HRP.
3.3.2 TInAS measurements. The TInAS allows quantitative analysis of the adsorbed dry mass on a surface of interest, as described by Heuberger and Balmer.58 The denpol–BAH–enzyme conjugates were applied on the SiO2 coated sensor as a dilute solution in buffer-4 at a concentration of about 2 μM r.u., see Materials and methods. For all of the three different denpol–BAH–enzyme conjugates, the adsorbed mass stabilized at a level of 550–650 ng cm−2 after about 60 min under these conditions (Fig. 4). Differences in the enzyme size were not reflected in these TInAS measurement, which yield average surface coverage.58,59 Therefore, it may be that de-PG2–BAH–HRP adsorbed more densely than de-PG2–BAH–GOD or de-PG2–BAH–(GOD,HRP). During subsequent washing of the sensor with buffer-4, there was no trace of desorption of the adsorbed mass from the surface within at least one hour, indicating a stable immobilization of the enzymes even without covalent attachment on the SiO2 surface. In control measurements with unmodified de-PG2, the adsorbed mass stabilized at a value of about 250 ng cm−2 (Fig. 4). Also in the case of the unmodified denpol, no desorption was observed during subsequent rinsing of the sensor with buffer-4, confirming the very stable adsorption of the polycationic polymer on the SiO2 surface as reported earlier.41,42 Based on an average adsorbed mass of 600 ng cm−2 for the conjugates, the amount of immobilized HRP can be calculated to be 11 pmol cm−2 in the case of adsorbed de-PG21400–BAH–HRP108 and 5.6 pmol cm−2 in the case of adsorbed de-PG21400–BAH–(GOD≈25,HRP78). Similarly, the amount of immobilized GOD was calculated to be 3.4 pmol cm−2 in the case of adsorbed de-PG21400–BAH–GOD≈50 and 1.8 pmol cm−2 with adsorbed de-PG21400–BAH–(GOD≈25,HRP78).69,70 These values are in the same order of magnitude as reported previously for enzymes immobilized by other methodologies involving both covalent71–73 or non-covalent bonds.41,42,70,74,75
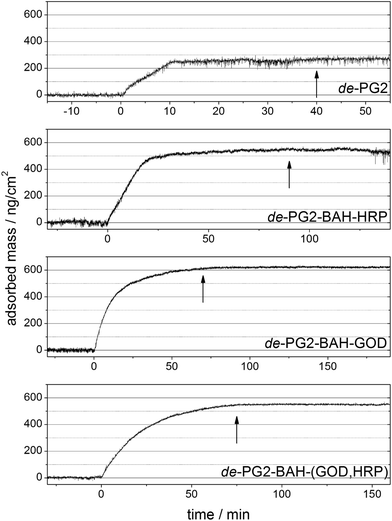 |
| Fig. 4 Adsorption of the denpol de-PG2 and the synthesized denpol–BAH–enzyme conjugates on sputtered silica surfaces monitored with the transmission interferometric adsorption sensor (TInAS). After equilibration of the flow cell with buffer-4, a solution of de-PG2 (A), de-PG2–BAH–HRP (B), de-PG2–BAH–GOD (C) or de-PG2–BAH–(GOD,HRP) (D) was injected, respectively. The adsorbed mass of the denpol–BAH–enzyme conjugates is higher than for de-PG2, without significant differences between the different conjugates. After stabilization of the adsorbed mass, desorption was probed by injecting buffer-4 into the flow cell (↑). Neither in the case of de-PG2 nor the denpol–BAH–enzyme conjugates any desorption was detected. | |
3.3.3 Immobilization on microscopy glass coverslips. To further analyze the immobilized denpol–BAH–enzyme conjugates, the same adsorption procedure as established for the TInAS measurements was applied for the immobilization of de-PG2–BAH–HRP, de-PG2–BAH–GOD or de-PG2–BAH–(GOD,HRP) on microscopy coverslips. These coverslips were then used for the analysis of the enzymatic activity and for a characterization of the homogeneity of the adsorbed conjugate layers by AFM after drying.
AFM measurements. Fig. 5 shows the 3D AFM images with corresponding transversal height profiles for de-PG2 (Fig. 5a), for de-PG2–BAH–HRP (Fig. 5b), de-PG2–BAH–GOD (Fig. 5c) and de-PG2–BAH–(GOD,HRP) (Fig. 5d), adsorbed on microscopy glass coverslips as used for the activity measurements (see below). The distribution of the denpol–BAH–enzyme conjugates on the surface is quite homogenous without large aggregates. AFM images with the scan size of 15 microns per edge are displayed in Fig. S5 in the ESI.† The corresponding transversal height profiles show that for most of the adsorbed denpol–BAH–enzyme conjugates deposition occurs as one layer. There are, however, some areas with overlapping chains (bright spots in Fig. 5). This is relevant for the substrate accessibility of the enzymes.
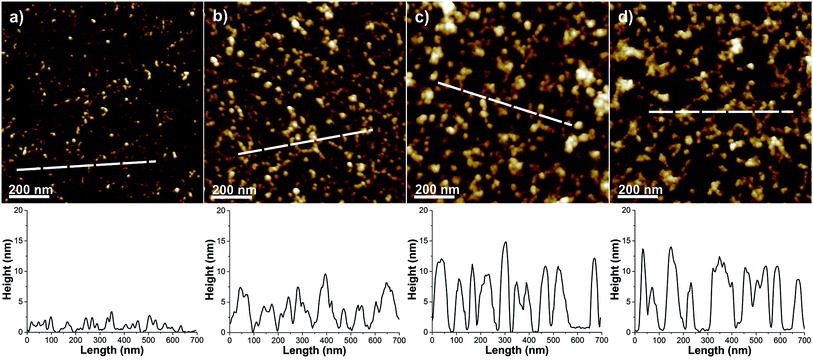 |
| Fig. 5 Tapping mode 3D AFM images of microscopy glass coverslips coated with de-PG2 (a), de-PG2–BAH–HRP (b), de-PG2–BAH–GOD (c), and de-PG2–BAH–(GOD,HRP) (d) with corresponding transversal height profiles along the indicated white lines. The height analysis confirms formation of a single layer of adsorbed denpol or denpol–BAH–enzyme conjugate, respectively, with a layer thickness consistent with the thickness of the different conjugates as observed in Fig. 3. | |
Enzyme activity measurements. Enzyme activities were evaluated using assays based on either the direct transformation of a chromogenic substrate in the case of HRP or an HRP coupled assay in the case of GOD. In both cases, the conversion was analyzed spectrophotometrically and correlated to the activity of the native enzymes in solution. Such comparison with appropriate calibration curves (see Fig. S3 and S4 (ESI†)) allows the determination of an apparent concentration of active enzyme per surface area {enzyme}apparent.For the quantification of HRP, the substrates ABTS2− and H2O2 were used. A surface of 1 cm2 with immobilized de-PG21400–BAH–HRP108 exhibited an enzymatic activity corresponding to 1.4 pmol HRP in bulk solution, i.e. {HRP}apparent = 1.4 pmol cm−2 for a de-PG21400–BAH–HRP108-coated glass surface.
The analysis of the enzymatic activity of immobilized de-PG21400–BAH–GOD≈50 was performed exploiting the cascade reaction including GOD and HRP as described by Fornera et al.42 using D-glucose, OPD and dissolved O2 as substrates and providing an excess of HRP in the assay solution for the second reaction step, see Materials and methods. This assay implied an apparent amount of active GOD {GOD}apparent of 1.3 pmol cm−2 for a de-PG21400–BAH–GOD≈50-coated glass surface.
The immobilized de-PG21400–BAH–(GOD≈25,HRP78) was similarly analyzed for GOD and HRP activity. In this case, the determined amount of active HRP on the glass coverslips was {HRP}apparent = 0.33 pmol cm−2 and of GOD {GOD}apparent = 0.28 pmol cm−2.
Comparing the activity based estimation of the apparent amount of immobilized enzyme (correlating the activity to bulk solution activities) and the TInAS based estimation (by calculation of the amount of adsorbed enzymes as part of the total adsorbed mass of the conjugates), it is clear that the estimated amounts based on activity were in all cases lower than the estimated amounts from the TInAS analysis. For de-PG21400–BAH–HRP108 the values were 1.4 pmol HRP cm−2 (enzyme activity) vs. 11 pmol HRP cm−2 (TInAS), for de-PG21400–BAH–GOD≈50 1.3 pmol GOD cm−2 vs. 3.4 pmol GOD cm−2; and for de-PG21400–BAH–(GOD≈25,HRP78) 0.33 pmol HRP cm−2 and 0.28 pmol GOD cm−2 vs. 5.6 pmol HRP cm−2 and 1.8 pmol GOD cm−2. There are at least two reasons for these differences. First, the given values are only estimations which involve several assumptions (see above). Second, the immobilized enzymes are expected to behave differently than dissolved free enzymes due to a restricted enzyme mobility and due to a restricted access of the substrate molecules to the enzymes' active sites if the enzymes are immobilized.16,76 A quantification and an estimation of the relative contributions of these effects is difficult and not possible to us at the moment. In any case, there is no doubt that at least a part of the immobilized enzymes is catalytically active. The question we then addressed was, whether the enzymes remain also active during operation and storage.
Stability of the immobilized enzymes. Immobilized GOD and HRP are quite stable if stored in wet state at 4 °C. To evaluate the storage stability of the immobilized denpol–BAH–enzyme conjugates, the coverslips were stored at pH = 7.0 and T = 4 °C. For immobilized GOD, a remaining activity of 80% was observed after 10 weeks, indicating a good stability under these conditions (Fig. 6). In the case of immobilized HRP, the activity dropped considerably to about 50% within the first week, but then stabilized at this level during further storage (Fig. 7). Similar behaviors were observed for GOD and HRP immobilized with de-PG2 and the biotin–avidin system.41,42 Interestingly, the storage stability of HRP of adsorbed de-PG2–BAH–(GOD,HRP) was improved considerably compared to the stability of the conjugate containing only HRP, as there was no significant reduction in activity after 3 weeks of storage (Fig. 8). In terms of GOD activity, the storage stability of the immobilized de-PG2–BAH–(GOD,HRP) conjugate was comparable to the stability of the conjugate containing only GOD, with an activity of about 85% after three weeks storage (Fig. 8). Additionally, the immobilized de-PG2–BAH–(GOD,HRP) conjugate was assayed for catalysis of the enzymatic cascade reaction. For this purpose, a solution of D-glucose and OPD was added to de-PG2–BAH–(GOD,HRP)-coated microscopy coverslips, but unlike in the GOD assay, no additional HRP was added. Under these conditions, the observed DAP formation could be attributed exclusively to the enzymatic cascade reaction catalyzed by GOD and HRP immobilized through the adsorption of de-PG2–BAH–(GOD,HRP). During storage at 4 °C, the activity decreased to about 75% within three weeks.
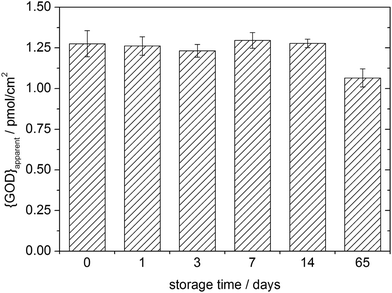 |
| Fig. 6 Storage stability of de-PG2–BAH–GOD adsorbed on glass coverslips stored at 4 °C in buffer-6. The enzymatic activity of GOD was measured using D-glucose and dissolved O2 as substrates in a coupled assay with HRP and its chromogenic substrate OPD. The apparent concentration of active enzyme immobilized on the glass surface, {GOD}apparent, was determined by using a calibration made with known amounts of free GOD in solution, see Fig. S1 (ESI†). The means and standard deviations are given as obtained from 3 measurements. | |
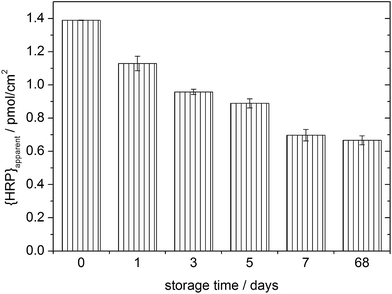 |
| Fig. 7 Storage stability of de-PG2–BAH–HRP adsorbed on glass coverslips stored at 4 °C in buffer-6. The enzymatic activity of HRP was determined using ABTS2− and H2O2 as substrates and the apparent enzyme concentration {HRP}apparent was determined using a calibration with known amounts of HRP in solution, see Fig. S2 (ESI†). The means and standard deviations are given as obtained from 3 measurements. | |
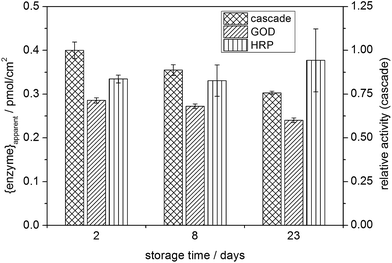 |
| Fig. 8 Storage stability of de-PG2–BAH–(GOD,HRP) adsorbed on glass coverslips stored at 4 °C in buffer-6. The enzymatic cascade reaction was monitored using D-glucose and dissolved O2 as substrates for GOD and the chromogenic HRP substrate OPD. The activities of GOD and HRP were monitored individually as described above (Fig. 6 and 7, respectively). The means and standard deviations are given as obtained from 3 measurements. | |
3.3.4 Immobilization inside glass micropipettes. Using the denpol–enzyme conjugates described above and commercially available glass micropipettes, the production of enzyme reactors for continuous flow operation could be achieved in a single adsorption step by simple filling of the glass micropipette with a dilute solution of the conjugate. Such micropipettes were used to analyze the operational stability of the two immobilized enzymes in two different setups: (i) sequentially immobilized and (ii) co-immobilized, see Materials and methods and Fig. 9a. To monitor the catalytic activity of the enzymatic cascade, a continuous feed of substrate was delivered with a peristaltic pump to the micropipettes in a flow reactor setup and the product formation was monitored for several hours using a Z-flow cell for online UV/vis spectrophotometric analysis.
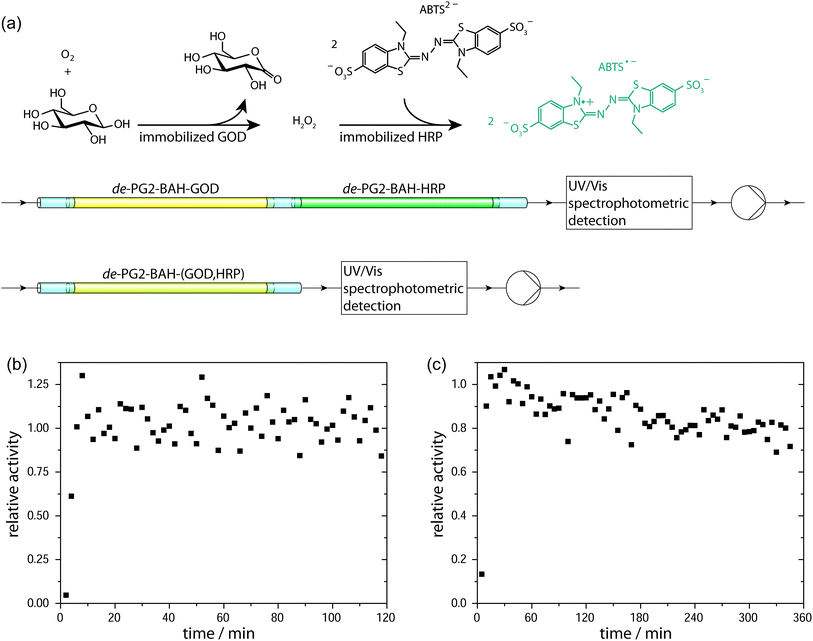 |
| Fig. 9 (a) Scheme of the flow reactors prepared with glass micropipettes containing immobilized enzymes. The sequential setup (top) contained a first micropipette with immobilized GOD (as de-PG2–BAH–GOD) connected to a second micropipette with immobilized HRP (as de-PG2–BAH–HRP), while the co-immobilization of GOD and HRP (bottom), as de-PG2–BAH–(GOD,HRP), allowed the formation of a flow reactor using only one glass micropipette. The conversion of the chromogenic substrate ABTS2− was monitored by online UV/vis analysis and the flow rate was controlled using a peristaltic pump. Oxidation of D-glucose with dissolved O2 catalyzed by GOD yields glucono-δ-lactone and H2O2, which in turn acts as oxidant to form the ABTS˙− from ABTS2− in the presence of catalytic HRP. The level of product formation was used as an indicator of the enzyme stabilities for the sequentially immobilized GOD and HRP (b) and for the co-immobilized GOD and HRP (c) under operating conditions. | |
Two-enzyme cascade reaction of sequentially immobilized GOD and HRP. A glass micropipette with adsorbed de-PG2–BAH–GOD was connected to a micropipette with adsorbed de-PG2–BAH–HRP and a substrate solution containing D-glucose, ABTS2− and dissolved O2 was fed to the micropipettes. The formation of ABTS˙− due to the enzymatic cascade reaction was monitored online at the outlet and the stability of the enzymes was determined in terms of decrease in the rate of ABTS˙− formation during continuous operation. For such a system, no significant decrease in activity was measured during the two hours of observation, see Fig. 9b.
Two-enzyme cascade reaction of co-immobilized GOD and HRP. Similar to the measurements of the sequential enzymatic cascade reaction, the co-immobilized GOD and HRP inside a glass micropipette, prepared by adsorption of de-PG2–BAH–(GOD,HRP) was analyzed in terms of operational stability by using the same substrate solution and a similar flow rate. Analysis of the conversion indicated retention of more than 90% of the initial activity after 2 h of operation, comparable to the sequentially immobilized enzymes, and still about 80% of the initial activity after 6 hours of continuous operation, see Fig. 9c.
Calculated catalytic cycles. For the sake of curiosity we calculated the average substrate conversion for each immobilized enzyme in the micropipette flow reactor during a total operation time of 2 hours under the condition used in the experiments shown in Fig. 9. Assuming that the amounts of immobilized GOD and HRP for the co-immobilization setup (Fig. 9c) were 1.8 pmol GOD cm−2 and 5.6 pmol HRP cm−2, respectively, a micropipette cut to 7 cm length contained about 6.3 pmol GOD and 19.6 pmol HRP.77 From the average product concentration measured during the first two hours of operation and considering the flow rate of 140 μL min−1 and a stoichiometry as indicated in Fig. 9a, each HRP converted 4800 molecules of ABTS2− to ABTS˙−, and each GOD converted 7400 molecules of D-glucose to glucono-δ-lactone.
4. Conclusions
We have demonstrated the successful synthesis of three different denpol–enzyme conjugates in aqueous solution by using a bis-aryl hydrazone (BAH) linker chemistry. Tracking the UV/vis signature of the BAH bond formed during conjugation allowed to estimate the average composition of the denpol–BAH–enzyme conjugates, yielding de-PG21400–BAH–HRP108, de-PG21400–BAH–GOD≈50, and de-PG21400–BAH–(GOD≈25,HRP78). The conjugates were characterized by AFM (in a dry state after deposition on mica and on glass coverslips) and by enzyme activity measurements. AFM analysis showed a uniform distribution of the enzymes along the denpol chain, indicating efficient conjugate formation. In the case of de-PG21400–BAH–(GOD≈25,HRP78), the preparation of a conjugate carrying two different types of enzymes, GOD and HRP, was possible by applying a strategy developed in our previous work in which denpol–enzyme conjugates were prepared which contained HRP and SOD;48,49 the larger enzyme was attached first (GOD in this work, HRP previously48,49), followed by the attachment of the smaller enzyme (HRP this work, SOD previously48,49).
The conjugates were used for the immobilization of the two enzymes GOD and HRP on glass surfaces, either separately or in combination, in a single step by simple exposure of the glass surface to a dilute aqueous solution of the corresponding conjugate. This adsorption step was quantified with the TInAS which indicated reproducible formation of a stable layer of denpol–BAH–enzyme conjugates on SiO2 surfaces without any sign of desorption; AFM imaging confirmed the formation of homogeneous layers on the surface of microscopy glass coverslips and indicated absence of enzymes which were not bound to the denpol. Although the immobilization of the denpol–BAH–enzyme conjugates occurred via non-covalent interactions, the conjugates remained strongly bound to the silica surface due to many contacts between the conjugate and the surface (no enzyme desorption from the surface). Using this enzyme immobilization technique allowed (i) the coating of glass coverslips for the quantification of the enzymatic activity and (ii) the preparation of a simple flow reactor using glass micropipettes. In such a flow reactor system, the operational stability of the enzyme was demonstrated in a sequential setup using de-PG2–BAH–GOD and de-PG2–BAH–HRP as well as in a setup using the simultaneous co-immobilization of GOD and HRP with de-PG2–BAH–(GOD,HRP). The high storage and operational stabilities of the immobilized enzymes can be correlated to the fact that denpol–BAH–enzyme conjugates do not desorb from the surface. This is – in combination with the simplicity of the enzyme immobilization involving a single adsorption step from a dilute aqueous solution of the conjugate only – a key characteristics of our novel enzyme immobilization methodology. In contrast to our earlier study using a sequential immobilization protocol involving the non-covalent biotin–avidin linker system,41,42 the adsorption in the approach presented here is not only mediated by the polycationic denpol but most probably also involves a considerable contribution from the enzyme. In other words, the strong and stable adherence of the denpol–BAH–enzyme conjugates to SiO2 surfaces is caused by multiple non-covalent interactions between the silicate surface and unmodified denpol repeating units as well as between the silicate surface and the denpol-bound enzymes. This results in a positioning of the enzyme molecules at some distance to the surface as well as close to it, which is seen as an advantage for enzymatic fuel cell applications in which electron transfer between an oxidative enzyme and an electrode occurs directly between the enzyme and the electrode and not through a mediator.50,52 Even though the catalytic activity of the enzymes might be impaired by enzyme–surface interactions,16,78 the introduction of the BAH linker system allows the formation of a dense enzyme layer, which is an important prerequisite for the successful co-immobilization of the two enzymes GOD and HRP, as presented in this work. Based on the success of the elaborated approach and the promising results obtained so far, we have recently shown that a spatially controlled enzyme localization by using denpol–enzyme conjugates is also possible in the case of mesoporous silicates.79 This demonstrates the potential of the approach as versatile enzyme-immobilization methodology complementary to already existing procedures. Furthermore, the denpol-mediated enzyme immobilization protocol may also be used for cascade reactions combining immobilized enzymes and organocatalysts.80
Acknowledgements
Dr Baozhong Zhang (Laboratory of Polymer Chemistry, Department of Materials, ETH Zürich) is acknowledged for providing the dendronized polymer, Dr Andrea Grotzky for providing the S-HyNic and S-4FB linkers, and Prof. Dr Nicholas Spencer (Surface Science and Technology, Department of Materials, ETH Zürich) for allowing to use the TInAS instrument.
Notes and references
- E. Katchalski-Katzir, Trends Biotechnol., 1993, 11, 471–478 CrossRef CAS PubMed.
- B. M. Brena and F. Batista-Viera, in Methods in Biotechnology: Immobilization of Enzymes and Cells, ed. J. M. Guisán, Humana Press Inc., Totowa, NJ, 2nd edn, 2006, pp. 15–30 Search PubMed.
- U. Hanefeld, L. Gardossi and E. Magner, Chem. Soc. Rev., 2009, 38, 453–468 RSC.
- R. A. Sheldon, Adv. Synth. Catal., 2007, 349, 1289–1307 CrossRef CAS.
- M. Hartmann and X. Kostrov, Chem. Soc. Rev., 2013, 42, 6277–6289 RSC.
- C. Mateo, J. M. Palomo, G. Fernandez-Lorente, J. M. Guisan and R. Fernandez-Lafuente, Enzyme Microb. Technol., 2007, 40, 1451–1463 CrossRef CAS.
- R. K. Singh, M. K. Tiwari, R. Singh and J.-K. Lee, Int. J. Mol. Sci., 2013, 14, 1232–1277 CrossRef CAS PubMed.
- R. A. Sheldon and S. van Pelt, Chem. Soc. Rev., 2013, 42, 6223–6235 RSC.
- R. C. Rodrigues, C. Ortiz, A. Berenguer-Murcía, R. Torres and R. Fernández-Lafuente, Chem. Soc. Rev., 2013, 42, 6290–6307 RSC.
- J. Kim, J. W. Grate and P. Wang, Trends Biotechnol., 2008, 26, 639–646 CrossRef CAS PubMed.
- D. N. Tran and K. J. Balkus Jr, ACS Catal., 2011, 1, 956–968 CrossRef CAS.
- P. Zucca and E. Sanjust, Molecules, 2014, 19, 14139–14194 CrossRef PubMed.
- O. Barbosa, R. Torres, C. Ortiz, Á. Berenguer-Murcia, R. C. Rodrigues and R. Fernandez-Lafuente, Biomacromolecules, 2013, 14, 2433–2462 CrossRef CAS PubMed.
- E. Magner, Chem. Soc. Rev., 2013, 42, 6213–6222 RSC.
- E. T. Hwang and M. B. Gu, Eng. Life Sci., 2013, 13, 49–61 CrossRef CAS.
- C. Garcia-Galan, Á. Berenguer-Murcia, R. Fernandez-Lafuente and R. C. Rodrigues, Adv. Synth. Catal., 2011, 353, 2885–2904 CrossRef CAS.
- J.-L. Lin, L. Palomec and I. Wheeldon, ACS Catal., 2014, 4, 505–511 CrossRef CAS.
- C. Draghici, J. Kowal, A. Darjan, W. Meier and C. G. Palivan, Langmuir, 2014, 30, 11660–11669 CrossRef CAS PubMed.
- J. Wiesbauer, J. M. Bolivar, M. Mueller, M. Schiller and B. Nidetzky, ChemCatChem, 2011, 3, 1299–1303 CrossRef CAS.
- J. M. Bolivar and B. Nidetzky, Langmuir, 2012, 28, 10040–10049 CrossRef CAS PubMed.
- J. M. Bolivar and B. Nidetzky, Biotechnol. Bioeng., 2012, 109, 1490–1498 CrossRef CAS PubMed.
- K. Nakanishi, A. Takeuchi and R. Matsuno, Appl. Microbiol. Biotechnol., 1990, 32, 633–636 CrossRef CAS PubMed.
- M. C. R. Franssen, P. Steunenberg, H. Zuilhof and J. P. M. Sanders, Chem. Soc. Rev., 2013, 42, 6491–6533 RSC.
- S. Cantone, V. Ferrario, L. Corici, C. Ebert, D. Fattor, P. Spizzo and L. Gardossi, Chem. Soc. Rev., 2013, 42, 6262–6276 RSC.
- R. DiCosimo, J. McAuliffe, A. J. Poulose and G. Bohlmann, Chem. Soc. Rev., 2013, 42, 6437–6474 RSC.
- K. Ariga, Q. Ji, T. Mori, M. Naito, Y. Yamauchi, H. Abe and J. P. Hill, Chem. Soc. Rev., 2013, 42, 6322–6345 RSC.
- J. Wang, Electrophoresis, 2002, 23, 713–718 CrossRef CAS PubMed.
- H. Mao, T. Yang and P. S. Cremer, Anal. Chem., 2002, 74, 379–385 CrossRef CAS PubMed.
- H. R. Luckarift, B. S. Ku, J. S. Dordick and J. C. Spain, Biotechnol. Bioeng., 2007, 98, 701–705 CrossRef CAS PubMed.
- Y. Asanomi, H. Yamaguchi, M. Miyazaki and H. Maeda, Molecules, 2011, 16, 6041–6059 CrossRef CAS PubMed.
- Y. S. Song, H. Y. Shin, J. Y. Lee, C. Park and S. W. Kim, Food Chem., 2012, 133, 611–617 CrossRef CAS.
- F. Costantini, R. Tiggelaar, S. Sennato, F. Mura, S. Schlautmann, F. Bordi, H. Gardeniers and C. Manetti, Analyst, 2013, 138, 5019–5024 RSC.
- M. Burchardt and G. Wittstock, Langmuir, 2013, 29, 15090–15099 CrossRef CAS PubMed.
- M. Safdar, J. Spross and J. Jänis, J. Chromatogr. A, 2014, 1324, 1–10 CrossRef CAS PubMed.
- R. J. Conrado, J. D. Varner and M. P. DeLisa, Curr. Opin. Biotechnol., 2008, 19, 492–499 CrossRef CAS PubMed.
- F. Lopez-Gallego and C. Schmidt-Dannert, Curr. Opin. Chem. Biol., 2010, 14, 174–183 CrossRef CAS PubMed.
- S. Schoeffelen and J. C. M. van Hest, Curr. Opin. Struct. Biol., 2013, 23, 1–9 CrossRef PubMed.
- F. Jia, B. Narasimhan and S. Mallapragada, Biotechnol. Bioeng., 2014, 111, 209–222 CrossRef CAS PubMed.
- G. Palazzo, G. Colafemmina, C. Guzzoni Iudice and A. Mallardi, Sens. Actuators, B, 2014, 202, 217–223 CrossRef CAS.
- J. Rocha-Martín, B. de las Rivas, R. Muñoz, J. M. Guisán and F. López-Gallego, ChemCatChem, 2012, 4, 1279–1288 CrossRef.
- S. Fornera, T. E. Balmer, B. Zhang, A. D. Schlüter and P. Walde, Macromol. Biosci., 2011, 11, 1052–1067 CrossRef CAS PubMed.
- S. Fornera, T. Bauer, A. D. Schlüter and P. Walde, J. Mater. Chem., 2012, 22, 502–511 RSC.
- Y. Guo, J. D. van Beek, B. Zhang, M. Colussi, P. Walde, A. Zhang, M. Kröger, A. Halperin and A. D. Schlüter, J. Am. Chem. Soc., 2009, 131, 11841–11854 CrossRef CAS PubMed.
- B. Zhang, R. Wepf, M. Kröger, A. Halperin and A. D. Schlüter, Macromolecules, 2011, 44, 6785–6792 CrossRef CAS.
-
(a) H. Yu, A. D. Schlüter and B. Zhang, Macromolecules, 2012, 45, 8555–8560 CrossRef CAS;
(b) H. Yu, A. D. Schlüter and B. Zhang, Macromolecules, 2014, 47, 4127–4135 CrossRef CAS.
- S. Fornera, P. Kuhn, D. Lombardi, A. D. Schlüter, P. S. Dittrich and P. Walde, ChemPlusChem, 2012, 77, 98–101 CrossRef CAS.
- The general concept of this immobilization of enzymes through a polycationic polymer and the biotin–avidin system is related to the approach undertaken previously by Zhen et al.: G. Zhen, V. Eggli, J. Vörös, P. Zammaretti, M. Textor, R. Glockshuber and E. Kuennemann, Langmuir, 2004, 20, 10464–10473 CrossRef CAS PubMed In that work a partially biotinylated PLL-g-PEG (with about 75–80 free amines per polymer chain) was used with neutrAvidin for the immobilization on niobium oxide (Nb2O5) of a genetically engineered β-lactamase which was biotinylated specifically.
- A. Grotzky, T. Nauser, H. Erdogan, A. D. Schlüter and P. Walde, J. Am. Chem. Soc., 2012, 134, 11392–11395 CrossRef CAS PubMed.
- A. Grotzky, E. Altamura, J. Adamcik, P. Carrara, P. Stano, F. Mavelli, T. Nauser, R. Mezzenga, A. D. Schlüter and P. Walde, Langmuir, 2013, 29, 10831–10840 CrossRef CAS PubMed.
- M. T. Meredith and S. D. Minteer, Annu. Rev. Anal. Chem., 2012, 5, 157–179 CrossRef CAS PubMed.
- W. Jia, C. Jin, W. Xia, M. Muhler, W. Schuhmann and L. Stoica, Chem.–Eur. J., 2012, 18, 2783–2786 CrossRef CAS PubMed.
- A. Heller and B. Feldman, Chem. Rev., 2008, 108, 2482–2505 CrossRef CAS PubMed.
- http://www.toyobo-global.com/seihin/xr/enzyme/e_top.html.
- S-HyNic and S-4FB are commercially available from Solulink, Inc., San Diego, California, http://www.solulink.com.
- B. E. P. Swoboda and V. Massey, J. Biol. Chem., 1965, 240, 2209–2215 CAS.
- A. Dirksen and P. E. Dawson, Bioconjugate Chem., 2008, 19, 2543–2548 CrossRef CAS PubMed.
- The conjugate preparation was carried out several times with always the same conditions as indicated above. As the concentrations of the stock solutions varied, the exact volumes were adjusted accordingly. For example, 69.3 μL buffer, 68.8 μL de-PG2-HyNic solution (with a HyNic concentration of 327 μM), and 94.8 μL GOD-4FB solution (with a 4FB concentration of 120 μM) were mixed for the first conjugation step, and after completion of the GOD coupling, 72.5 μL of HRP-4FB solution (with a 4FB concentration of 207 μM) were added.
- M. Heuberger and T. E. Balmer, J. Phys. D: Appl. Phys., 2007, 40, 7245–7254 CrossRef CAS.
- T. Sannomiya, T. E. Balmer, M. Heuberger and J. Vörös, J. Phys. D: Appl. Phys., 2010, 43, 405302 CrossRef.
- N. C. Veitch and A. T. Smith, Adv. Inorg. Chem., 2000, 51, 107–162 CrossRef.
- J. H. Pazur, K. Kleppe and A. Cepure, Arch. Biochem. Biophys., 1965, 111, 351–357 CrossRef CAS PubMed.
- H. Ye, Anal. Biochem., 2006, 356, 76–85 CrossRef CAS PubMed.
- R. E. Childs and W. G. Bardsley, Biochem. J., 1975, 145, 93–103 CrossRef CAS PubMed.
- K. C. Brown, J. F. Corbett and N. P. Loveless, Spectrochim. Acta, Part A, 1979, 35, 421–423 CrossRef.
- S. Fornera and P. Walde, Anal. Biochem., 2010, 407, 293–295 CrossRef CAS PubMed.
- A. Grotzky, Y. Manaka, T. Kojima and P. Walde, Biomacromolecules, 2011, 12, 134–144 CrossRef CAS PubMed.
- Calculated value based on the total amount of amino groups present in one de-PG21400 chain, 4 × 1400 = 5600, and taking into account the average number of bound HRP molecules per de-PG21400 chain, 108, and the average number of unreacted HyNic groups per de-PG21400 chain, 116: 5600 − 108 − 116 = 5376.
- The values given for the approximate amount of GOD bound to the denpol are based on a determination of the upper limit of bound GOD through measurements of ΔA354 nm during the conjugation reaction and then taking one third of this value (see text for details).
- The calculations are based on the determined (or estimated) molar ratio of enzyme to denpol chain in the denpol–BAH–enzyme conjugates and taking into account the molar masses of the enzymes, 44 kDa for HRP and 153 kDa for GOD, respectively.
- The amounts of immobilized HRP and GOD determined for de-PG21400–BAH–HRP108 and de-PG21400–BAH–GOD≈50, 11 pmol cm−2 and 5.6 pmol cm−2, respectively, are comparable to the previously determined amounts of immobilized HRP and GOD by using de-PG21000 and the biotin–avidin system: ≈0.6–1.4 pmol cm−2 for HRP,41 and ≈0.8 pmol cm−2 for GOD.42.
- HRP was covalently bound to modified glass slides at a surface density of (1.9 ± 0.3) × 1012 HRP molecules per cm2, which corresponds to ≈3 pmol cm−2: F. Vianello, L. Zennaro, L. M. L. Di Paolo, A. Rigo, C. Malacarne and M. Scarpa, Biotechnol. Bioeng., 2000, 68, 488–495 CrossRef CAS PubMed.
- Candida rugosa lipase was covalently attached to a polymer-coated glass plate at a surface density of ≈5 pmol cm−2 (0.50 × 10−7 mol lipase cm−2): P. Ye, R.-B. Wan and X.-P. Wang, J. Mol. Catal. B: Enzym., 2009, 61, 296–302 CrossRef CAS.
- HRP was covalently bound to polymer brushes which were first grown from a modified silicon wafer, yielding a surface density of up to ≈4 pmol cm−2 (2.6 × 1012 HRP molecules per cm2): S. M. Lane, Z. Kuang, J. Yom, S. Arifuzzaman, J. Genzer, B. Farmer, R. Naik and R. A. Vaia, Biomacromolecules, 2011, 12, 1822–1830 CrossRef CAS PubMed.
- Alkaline phosphatase conjugated to streptavidin was immobilized non-covalently on a biotinylated phospholipid bilayer-coated borosilicate surface, yielding a surface coverage of ≈1.2 pmol cm−2 (≈1.5 × 10−13 mol phosphatase molecules immobilized onto an area of 1.22 × 1013 nm2).28.
- Trametes versicolor laccase was immobilized non-covalently within a layer of amphiphilic triblock copolymers which were adsorbed onto silica slides, yielding ≈1–7 pmol cm−2 (0.6 to 4.21 × 1012 laccase molecules per cm2), depending on the polymers used.18.
- P. Grunwald, Biocatalysis: Biochemical Fundamentals and Applications, Imperial College Press, London, 2009 Search PubMed.
- Calculated on the basis of the following: a micropipette cut to a length of 7 cm contained a volume of 140 μL. By assuming the shape of a perfect cylinder, this gives a total surface of 3.5 cm2 per micropipette.
- F. Secundo, Chem. Soc. Rev., 2013, 42, 6250–6261 RSC.
- H. Gustafsson, A. Küchler, K. Holmberg and P. Walde, submitted.
- K. Kandel, S. M. Althaus, M. Pruski and I. I. Slowing, ACS Symp. Ser., 2013, 1132, 261–271 CrossRef CAS.
Footnote |
† Electronic supplementary information (ESI) available: Spectrophotometric monitoring of denpol–enzyme conjugation, enzyme activity calibration curves, AFM analysis of microscopy glass coverslips. See DOI: 10.1039/c5ra06268c |
|
This journal is © The Royal Society of Chemistry 2015 |