DOI:
10.1039/C5RA06072A
(Paper)
RSC Adv., 2015,
5, 43268-43278
Functional group effects on structure and topology of cadmium(II) frameworks with mixed organic ligands†
Received
5th April 2015
, Accepted 6th May 2015
First published on 7th May 2015
Abstract
Four new Cd(II) frameworks [Cd(tib)(p-BDC-OH)]·H2O (1), [Cd(tib)(m-BDC-OH)]·3H2O (2), [Cd(tib)(m-BDC-CH3)]·3H2O (3) and [Cd(tib)(m-BDC-CH3)]·11H2O (4) (tib = 1,3,5-tris(1-imidazolyl)benzene, p-H2BDC-R = 2-R-1,4-benzenedicarboxylic acid, m-H2BDC-R = 5-R-1,3-benzenedicarboxylic acid, R = OH or CH3) have been successfully synthesized under solvothermal conditions. 1 is a 3D framework with a Point symbol of {810}{83} constructed from Cd-tib 3D net and Cd-p-BDC-OH 1D helical chain. 2 and 3 possess different 3D structures with the same topology of {4·62}{4·66·83} built from Cd-tib 2D networks and Cd-m-BDC-R 1D linear chains, while 4 is a 2D network with {42·67·8}{42·6} topology based on Cd-tib and Cd-m-BDC-CH3 1D chains. The results show that the dicarboxylate ligands and reaction medium play important role in determining the structures of MOFs. Moreover, ferroelectric and photoluminescence properties of the complexes were studied.
Introduction
Metal–organic frameworks (MOFs) continue to attract great interest from chemists due to their intriguing architectures and potential applications in gas storage/separation, host–guest chemistry, catalysis, luminescence and chemical sensing.1–5 Such applications may benefit from their outstanding characteristics that can be tailored by choosing suitable organic linkers and metal ions as well as external reaction conditions.6 Among all the factors, the organic ligands play important role in manipulating versatile structures, and the length, rigidity, shape, symmetry, coordination modes, functional groups or substituents of the organic ligands can bring about consequential effects on the final structures and properties of MOFs.7,8
Recently, the functionalization and modification of the ligands to construct new MOFs have received considerable attention. In this field, one important aspect is the ability to alter their chemical composition, functionality, and molecular dimensions systematically without changing their underlying topology, which was termed as reticular synthesis. The most well known example of this is the synthesis of IRMOFs by Yaghi and co-workers where 1,4-benzenedicarboxylate (H2BDC) was modified with different functional groups (e.g., –NH2, –OH, –Br, –CH3) or substituted with more extended dicarboxylate ligands, resulting to tunable pore sizes and properties.8 MOFs modified with functional groups not only facilitates direct interaction with the gas substrate, fluorescence and other physical properties, but also provides additional coordination sites.9 Of course this modification can also be achieved by postsynthetic modification (PSM),10 to give frameworks with specific chemical functionality. One vital factor of reticular synthesis depends on the fact that the functional groups do not alter the topology of the desired MOFs, which occurs most often when the functional groups can interact with metal ions in a way that would interfere with MOF synthesis.8,11 Some other reticular synthesis such as MIL-53,12 UMCM,13 and UiO-66 have been realized.14 However, intensive exploration of functional group effects in carboxylate ligands on MOF structure and topology has been subjected to rare attention in mixed-ligand system. Up to now, only several MOFs have been reported to study functional group effects in mixed-ligand system.15
In our previous work, the reaction of 2-R-1,4-benzenedicarboxylic acid (p-H2BDC-R, R = NH2, OH, NO2) with Zn(II)-1,3,5-tris(1-imidazolyl)benzene (tib) system allowed for formation of a series of frameworks with diversity of topologies.16 As a continuous work, we introduced p-H2BDC-OH and 5-R-1,3-benzenedicarboxylic acid (m-H2BDC-R, R = OH, CH3) into Cd(II)-tib system and finally obtained four new Cd(II) MOFs [Cd(tib)(p-BDC-OH)]·H2O (1), [Cd(tib)(m-BDC-OH)]·3H2O (2), [Cd(tib)(m-BDC-CH3)]·3H2O (3) and [Cd(tib)(m-BDC-CH3)]·11H2O (4). Herein, we reported their syntheses, structures and luminescence properties.
Experimental
Materials and methods
All commercially available chemicals and solvents are of reagent grade and were used as received without further purification. The tib ligand was synthesized according to the method reported previously.17 Elemental analyses for C, H and N were performed on a Perkin-Elmer 240C Elemental Analyzer at the analysis center of Nanjing University. FT-IR spectra were recorded in the range of 400–4000 cm−1 on a Bruker Vector22 FT-IR spectrophotometer using KBr pellets. Powder X-ray diffraction (PXRD) measurements were performed on a Bruker D8 Advance X-ray diffractometer using Cu-Kα radiation (λ = 1.5418 Å), in which the X-ray tube was operated at 40 kV and 40 mA. Thermogravimetric analyses (TGA) were performed on a Mettler-Toledo (TGA/DSC1) thermal analyzer under the N2 atmosphere with a heating rate of 10 °C min−1. The electric hysteresis loops were obtained on a Ferroelectric Tester Precision Premier II supplied by Radiant Technologies, Inc. The luminescence spectra for the powdered solid samples were measured on an Aminco Bowman Series 2 spectrofluorometer with a xenon arc lamp as the light source. In the measurements of emission and excitation spectra the pass width is 5 nm, and all the measurements were carried out under the same experimental conditions.
Preparation of [Cd(tib)(p-BDC-OH)]·H2O (1). A mixture of tib (8.2 mg, 0.03 mmol), p-H2BDC-OH (5.5 mg, 0.03 mmol), CdCO3 (10.3 mg, 0.06 mmol), N,N-dimethylacetamide (DMA, 2 mL) and H2O (6 mL) was sealed in Teflon-lined stainless steel container and heated at 85 °C for 3 days. After being cooled to room temperature, colorless needle-like crystals of 1 were isolated in 75% yield. Anal. calcd for C23H18CdN6O6: C 47.07, H 3.09, N 14.32%. Found: C 47.16, H 3.22, N 14.18%. IR (KBr pellet, cm−1): 3457 (s), 3137 (m), 1621 (s), 1586 (w), 1537 (m), 1507 (s), 1430 (m), 1399 (m), 1290 (m), 1242 (m), 1116 (m), 1073 (m), 1019 (m), 928 (w), 838 (w), 807 (w), 760 (w), 736 (m), 681 (w), 651 (w).
Preparation of [Cd(tib)(m-BDC-OH)]·3H2O (2). A mixture of tib (8.2 mg, 0.03 mmol), m-H2BDC-OH (5.5 mg, 0.03 mmol), CdCO3 (10.3 mg, 0.06 mmol), ethanol (EtOH, 2 mL), 0.5 M NaOH (0.05 mL) and H2O (8 mL) was sealed in Teflon-lined stainless steel container and heated at 85 °C for 3 days. After being cooled to room temperature, colorless block crystals of 2 were obtained in 76% yield. Anal. calcd for C23H22CdN6O8: C 44.35, H 3.56, N 13.49%. Found: C 44.53, H 3.47, N 13.55%. IR (KBr pellet, cm−1): 3440 (m), 3120 (w), 1743 (w), 1617 (s), 1544 (s), 1381 (s), 1248 (m), 1109 (m), 1073 (s), 1013 (m), 977 (w), 934 (w), 886 (w), 832 (w), 790 (w), 760 (w), 723 (m), 681 (w), 645 (m).
Preparation of [Cd(tib)(m-BDC-CH3)]·3H2O (3). Complex 3 was synthesized by the same procedure of 2, except that m-H2BDC-CH3 (5.5 mg, 0.03 mmol) was used instead of m-H2BDC-OH, colorless block crystals of 3 were obtained in 56% yield. Anal. calcd for C24H24CdN6O7: C 46.43, H 3.90, N 13.54%. Found: C 46.39, H 3.95, N 13.61%. IR (KBr pellet, cm−1): 3404 (m), 3150 (w), 1623 (s), 1508 (s), 1423 (m), 1369 (s), 1309 (w), 1248 (m), 1115 (m), 1067 (s), 1013 (s), 934 (m), 886 (w), 813 (w), 766 (w), 736 (m), 656 (m), 602 (w).
Preparation of [Cd(tib)(m-BDC-CH3)]·11H2O (4). A mixture of tib (8.2 mg, 0.03 mmol), m-H2BDC-CH3 (5.5 mg, 0.03 mmol), Cd(NO3)2·4H2O (15.4 mg, 0.05 mmol), DMA (6 mL) and H2O (2 mL) was sealed in Teflon-lined stainless steel container and heated at 85 °C for 3 days. After being cooled to room temperature, colorless block crystals of 4 were obtained in 80% yield. Anal. calcd for C24H40CdN6O15: C 37.68, H 5.27, N 10.99%. Found: C 37.64, H 5.54, N 10.86%. IR (KBr pellet, cm−1): 3409 (m), 3126 (w), 1617 (s), 1562 (m), 1502 (s), 1417 (w), 1369 (s), 1385 (w), 1242 (m), 1116 (w), 1079 (s), 1019 (s), 928 (m), 862 (w), 777 (w), 729 (m), 687 (w), 656 (m), 591 (w).
X-ray crystallography
The crystallographic data collections for 1–4 were carried out on a Bruker Smart Apex II CCD area-detector diffractometer with graphite-monochromated Mo Kα radiation (λ = 0.71073 Å) at 293(2) K using the ω-scan technique. The diffraction data were integrated by using the SAINT program,18 which was also used for the intensity corrections for the Lorentz and polarization effects. Semi-empirical absorption corrections were applied using SADABS program.19 The structures were solved by direct methods and all the non-hydrogen atoms were refined anisotropically on F2 by the full-matrix least-squares technique using the SHELXL-97 crystallographic software package.20 The hydrogen atoms except those of water molecules were generated geometrically and refined isotropically using the riding model. The hydrogen atoms of free water molecules in 1–4 were not found. The free water molecules in 2 are disordered. In order to subtract the contribution of the disordered solvent molecules in 4, the SQUEEZE command was applied. SQUEEZE removed eleven H2O molecules for 4 per formula unit. This value is calculated based upon volume/count-electrons analysis and TG data of 4. For this complex, all hydrogen atoms attached to water molecules were not located, but were included in the structure factor calculations.21 The details of the crystal parameters, data collection, and refinements for the complexes are summarized in Table 1 and selected bond lengths and angles are listed in Table S1 (ESI†) and hydrogen bonding data for 4 are given in Table S2 (ESI†).
Table 1 Crystal data and structure refinements for complexes 1–4
|
1 |
2 |
3 |
4 |
R1 = ∑‖Fo| − |Fc‖/∑|Fo|. wR2 = |∑w(|Fo|2 − |Fc|2)|/∑|w(Fo)2|1/2, where w = 1/[σ2(Fo2) + (aP)2 + bP]. P = (Fo2 + 2Fc2)/3. |
Empirical formula |
C23H18CdN6O6 |
C23H22CdN6O8 |
C24H24CdN6O7 |
C24H40CdN6O15 |
Formula weight |
586.83 |
622.87 |
620.89 |
765.02 |
Crystal system |
Tetragonal |
Monoclinic |
Monoclinic |
Orthorhombic |
Space group |
P41 |
P21/n |
P21/c |
Pbca |
a (Å) |
9.0420(3) |
9.8120(3) |
11.5640(16) |
13.8290(5) |
b (Å) |
9.0420(3) |
14.2830(5) |
22.347(3) |
20.2540(8) |
c (Å) |
27.231(19) |
19.5720(6) |
10.1790(14) |
25.7650(10) |
β (°) |
90.00 |
96.9410(10) |
103.343(2) |
90.00 |
V (Å3) |
2226.35(19) |
2722.81(15) |
2559.5(6) |
7216.6(5) |
Z |
4 |
4 |
4 |
8 |
ρcalcd (g cm−3) |
1.751 |
1.519 |
1.611 |
1.408 |
μ (mm−1) |
1.036 |
0.857 |
0.909 |
0.675 |
F(000) |
1176 |
125 |
1256 |
3152 |
Rint |
0.0176 |
0.0181 |
0.0339 |
0.0666 |
Data collected |
13 342 |
14 937 |
17 247 |
67 567 |
Independent data |
3800 |
4795 |
5909 |
8275 |
Flack parameter |
0.004(19) |
— |
— |
— |
Goodness-of-fit |
1.100 |
1.180 |
1.057 |
1.041 |
R1a (I > 2σ(I)) |
0.0206 |
0.0430 |
0.0319 |
0.0413 |
wR2b (I > 2σ(I)) |
0.0585 |
0.1605 |
0.0808 |
0.1126 |
Results and discussion
Crystal structures of [Cd(tib)(p-BDC-OH)]·H2O (1)
Crystallographic analysis indicates that 1 crystallizes in tetragonal space group P41. As shown in Fig. 1a, Cd1 is seven-coordinated with distorted pentagonal bipyramidal coordination geometry, in which the equatorial positions are occupied by four oxygen atoms (O1, O2, O3, O4) from two chelating carboxylate groups and one imidazole nitrogen atom (N5), and the axial positions are coordinated by two imidazole nitrogen atoms (N1, N3A). The Cd–N and Cd–O bond distances are in the range 2.276(3)–2.439(3) Å and 2.339(3)–2.579(3) Å (Table S1, ESI†), which are well-matched to those observed in the reported complexes.22 In 1, p-H2BDC-OH is completely deprotonated and each p-BDC-OH adopts a μ2-bridging mode to connect two Cd(II) atoms with its two carboxylate groups in (κ2)-(κ2)-μ2-p-BDC-OH coordination mode (Scheme 1a). p-BDC-OH ligands link Cd(II) atoms to form a one-dimensional (1D) helical chain (Fig. 1b). Meanwhile all the imidazole nitrogen atoms of tib ligand take part in coordination with Cd(II) atoms, and in turn each Cd(II) is coordinated by three distinct tib ligands to give a 3D framework (Fig. 1c). From topological view, the part of Cd(II)-tib 3D framework is 3-connected uninodal (ths or ThSi2) net, which is a 2-fold interpenetrating 3D framework with Point symbol of {103} (Fig. S1a, ESI†). Finally, the 1D chain fills the Cd-tib 3D structure, leading to the formation of the final 3D structure of 1 (Fig. 1d).
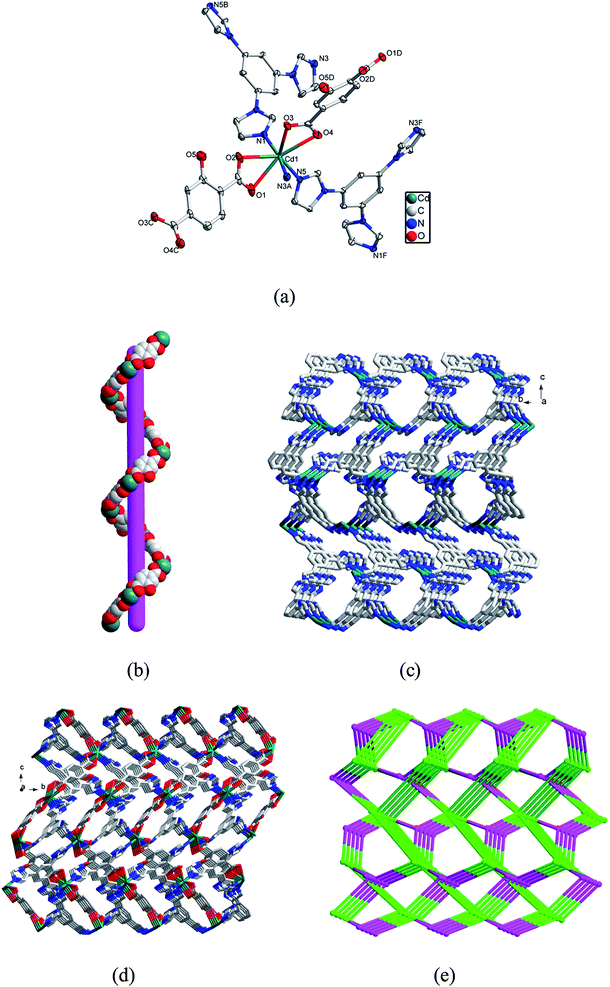 |
| Fig. 1 (a) The coordination environment of Cd(II) in 1 with the ellipsoids drawn at the 30% probability level. Hydrogen atoms and free water molecules are omitted for clarity. (b) The helical chain constructed by Cd(II) and p-BDC-OH. (c) The 3D framework of Cd(II)-tib. (d) The final 3D framework of 1. (e) Topology of 1: bright green, Cd atoms; pink, tib ligands. | |
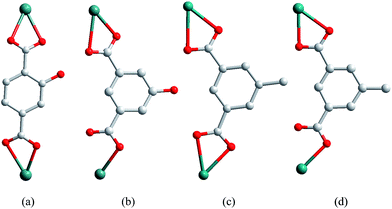 |
| Scheme 1 Coordination modes of BDC-R appeared in 1–4. | |
A better insight into the nature of this intricate architecture can be achieved by application of topological approach.23 As described above, the Cd(II) atom is surrounded by three tib and two p-BDC-OH ligands and thus can be viewed as a five-connector (Fig. S2a, ESI†), while the tib and p-BDC-OH ligands are considered as three- and two-connector, respectively. Such connectivity repeats infinitely to give the 3D framework of 1 as schematically shown in Fig. 1e. According to the simplification principle,24 the resulting structure of 1 can be simplified as a (3, 5)-connected 2-nodal 3D net with Point (Schläfli) symbol of {810}{83}.
Crystal structure of [Cd(tib)(m-BDC-OH)]·3H2O (2)
Crystallographic analysis reveals that the asymmetric unit of 2 contains one Cd(II) atom, one tib, one m-BDC-OH and three free water molecules. Each Cd(II) center is six-coordinated with distorted octahedral coordination geometry by three imidazole nitrogen (N1, N3C, N5A) from three distinct tib and three carboxylate oxygen atoms (O1, O2, O4B) from two different m-BDC-OH ligands (Fig. 2a). The Cd–N and Cd–O bond distances are in the range of 2.267(4)–2.431(4) Å and 2.296(4)–2.643(5) Å (Table S1, ESI†), respectively. Each m-BDC-OH in 2 connects two metal atoms using its two carboxylate groups in (κ1)-(κ2)-μ2-m-BDC-OH coordination mode (Scheme 1b), forming a 1D linear chain (Fig. 2b), rather than the 1D helix in 1 (Fig. 1b). Each tib links three Cd(II) centers to generate a two-dimensional (2D) network (Fig. 2c and S1b), which is further linked together by Cd-m-BDC-OH 1D chain to produce the final 3D structure of 2 (Fig. 2d). The topological analysis was carried out, and the simplified overall structure of 2 is a (3, 5)-connected 2-nodal 3D net with Point (Schläfli) symbol of {4·62}{4·66·83} (Fig. 2e).
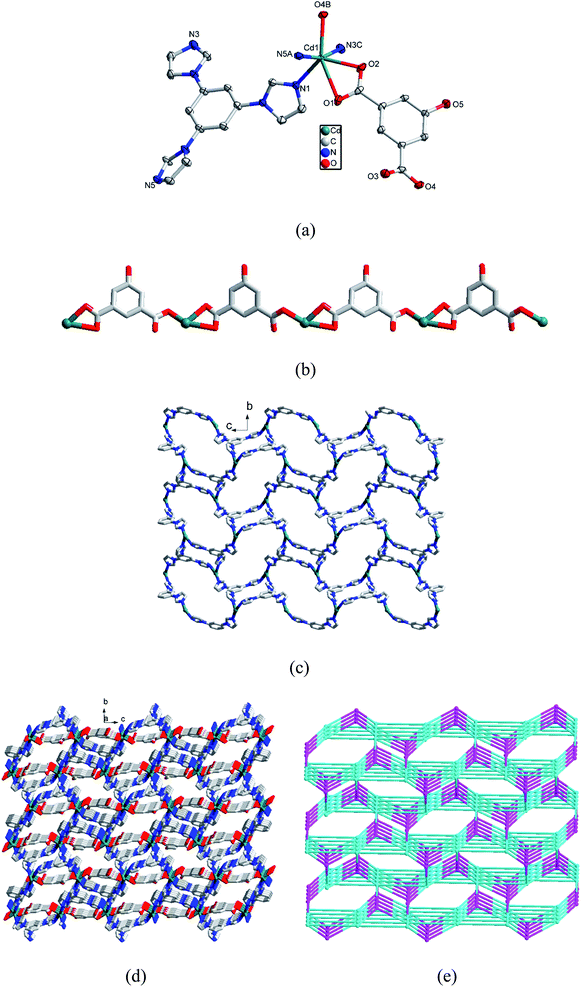 |
| Fig. 2 (a) The coordination environment of Cd(II) in 2 with the ellipsoids drawn at the 30% probability level. Hydrogen atoms and free water molecules are omitted for clarity. (b) The 1D chain built by Cd(II) and m-BDC-OH. (c) The 2D network of Cd(II)-tib. (d) The 3D framework of 2. (e) Topology of 2: turquoise, Cd atoms; pink, tib ligands. | |
Crystal structure of [Cd(tib)(m-BDC-CH3)]·3H2O (3)
When m-H2BDC-CH3, instead of m-H2BDC-OH, was used in the reaction under the same reaction conditions, complex 3 with different structure was obtained. The crystal structure of 3 is shown in Fig. 3a. Seven-coordinated Cd1 is surrounded by three imidazole nitrogen atoms (N1, N3C, N5A) belonging to three distinct tib and four carboxylate oxygen atoms (O1, O2, O3B, O4B) from two different m-BDC-CH3 to complete its distorted pentagonal bipyramidal coordination geometry. The Cd–N and Cd–O bond distances are in the range of 2.261(2)–2.458(2) Å and 2.3494(19)–2.623(3) Å (Table S1, ESI†), respectively. It is notable that m-BDC-CH3 connects two Cd(II) atoms with its two carboxylate groups in (κ2)-(κ2)-μ2-m-BDC-CH3 coordination mode (Scheme 1c) to give a 1D linear chain (Fig. 3b), likewise each tib links three Cd(II) to form a 2D network (Fig. 3c), which is further pillared by m-BDC-CH3 to eventually generate the 3D framework of 3 (Fig. 3d). Topological analysis by TOPOS program suggests that the Point (Schläfli) symbol of the 3D net is {4·62}{4·66·83} (Fig. 3e). It is noteworthy that there are large open channels in 3 (Fig. 3d), it is apt to form interpenetrating frameworks,25 accordingly, the final structure of 3 is a 2-fold interpenetrating 3D framework (Fig. 3f).
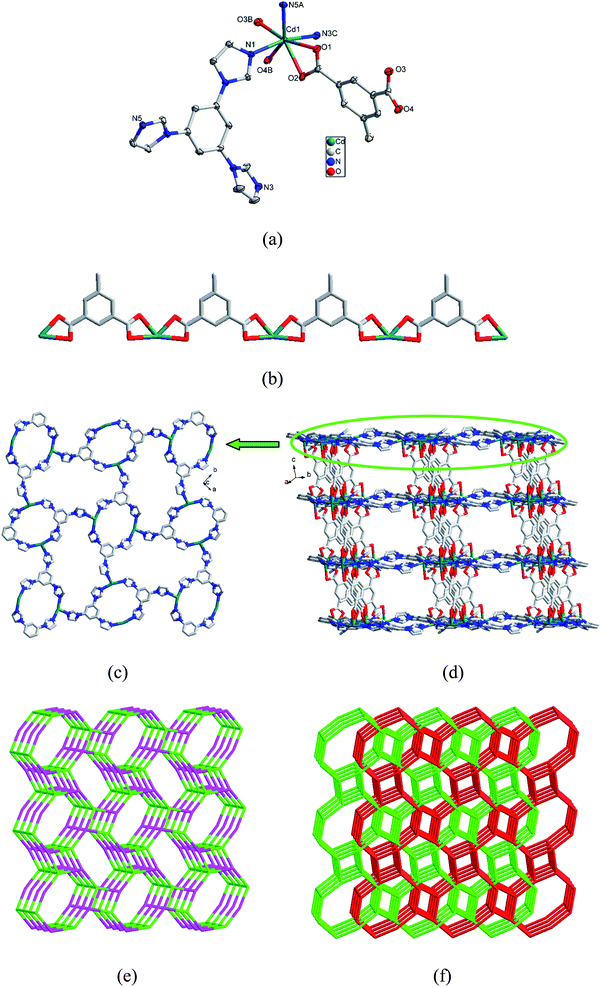 |
| Fig. 3 (a) The coordination environment of Cd(II) in 3 with the ellipsoids drawn at the 30% probability level. Hydrogen atoms and free water molecules are omitted for clarity. (b) The 1D chain constructed by Cd(II) and m-BDC-CH3. (c) The 2D network of Cd(II)-tib. (d) The 3D framework of 3. (e) Topology of 3: bright green, Cd atoms; pink, tib ligands. (f) The schematic representation of 2-fold interpenetration of 3. | |
It is deserve to mentioned that 2 and 3, isolated by the same procedure except the different substituent groups of m-H2BDC, possess different 3D frameworks but with the same Point (Schläfli) symbol of {4·62}{4·66·83},26 which is rare in the previously reported MOFs. It can be seen the influence of the substituent groups on their structures. First, the Cd(II) center in 2 is six-coordinated in a octahedral coordination geometry, while the one in 3 adopts a distorted pentagonal bipyramidal coordination geometry. Second, although Cd-m-BDC-R moieties in 2 and 3 are similar 1D chains, only three oxygen atoms of two carboxylate groups in m-H2BDC-OH take part in coordination with Cd(II) in 2, whereas all four oxygen ones of two carboxylate groups in m-H2BDC-CH3 coordinate with Cd(II) in 3. Third, tib ligands adopt different conformation to coordinate with Cd(II) (Fig. S3, ESI†). Such difference can be ascribed to steric and/or electronic effect of the substituent groups.15 The Cd-m-BDC-R 1D chains interpenetrate Cd-tib 2D networks obliquely in 2 whereas the ones in 3 pillar Cd-tib 2D networks vertically, both of which resulting in formation of non- and 2-fold interpenetrating 3D frameworks (Fig. S4, ESI†). Furthermore, the degree of the distortion of two Cd-tib networks is different, which can also do contribution to the different frameworks. The results also imply an approach to control interpenetration of MOFs by tuning the substituent groups of the auxiliary carboxylate ligands.27
Crystal structure of [Cd(tib)(m-BDC-CH3)]·11H2O (4)
Considering the counter anion and reaction medium can have influence on structure of MOFs, CdCO3 was replaced by Cd(NO3)2·4H2O and the reaction was carried out in DMA–H2O, complex 4 was isolated. As shown in Fig. 4a, each Cd(II) atom is located in a six-coordinated environment with three imidazole nitrogen atoms (N1, N3B, N5A) from three distinct tib and three carboxylate oxygen (O1, O3C, O4C) from two m-BDC-CH3 ligands. Each m-BDC-CH3 uses its two carboxylate groups in (κ1)-(κ2)-μ2-m-BDC-CH3 coordination mode (Scheme 1d) to join two Cd(II) atoms to form a 1D linear chain (Fig. 4b). Each tib in 4 acts as a tridentate linker to connect three Cd(II) atoms forming a 1D ladder chain (Fig. 4c), rather than the 2D network in 3 (Fig. 3c). Finally the m-BDC-CH3 links the 1D Cd(II)-tib chains to give a 2D network of 4 (Fig. 4d). From topological view, the 2D network of 4 can be simplified as (3, 5)-connected 2-nodal net, and its Point symbol is {42·67·8}{42·6} (Fig. 4e). In addition, the adjacent 2D networks are further linked together by hydrogen bonds to give a 3D supramolecular structure of 4 (Table S2 and Fig. S5, ESI†).
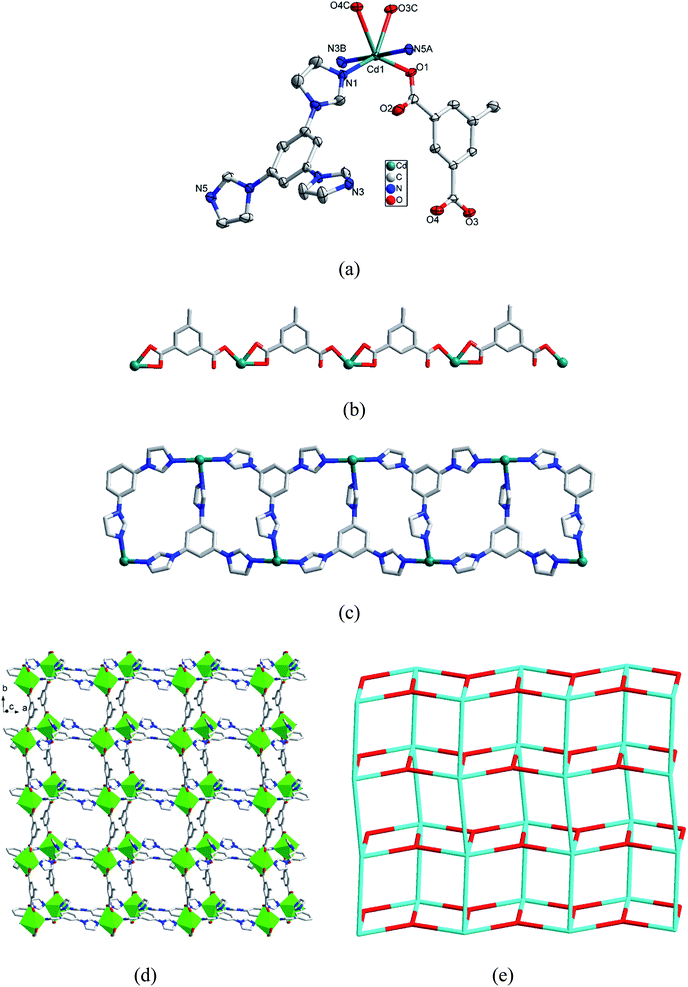 |
| Fig. 4 (a) The coordination environment of Cd(II) in 4 with the ellipsoids drawn at the 30% probability level. Hydrogen atoms and free water molecules are omitted for clarity. (b) The 1D chain formed by Cd(II) and m-BDC-CH3. (c) The 1D ladder chain of Cd(II)-tib. (d) The 2D network of 4. (e) Topology of 4: turquoise, Cd atoms; red, tib ligands. | |
Synthesis and comparison of the structures
In the present study, p-H2BDC-OH and m-H2BDC-R (R = OH; CH3) ligands were employed to react with Cd(II)-tib and new MOFs 1–4 were successfully isolated. The substituted H2BDC in 1–4 are all deprotonated to give BDC2− ligands as evidenced by X-ray crystallography as described above as well as by the IR spectral data since no vibration bands from –COOH were observed between 1680 and 1760 cm−1 (see Experimental section). It is noteworthy that each substituted BDC2− ligand links two Cd(II) and each tib connects three Cd(II) atoms in 1–4, however, the resulted structures are different. Namely, the Cd-p-BDC-OH is 1D helical chain in 1, while the Cd-m-BDC-R in 2–4 is 1D linear chain. More importantly, the Cd-tib moieties vary from 1D chain in 4, different 2D networks in 2 and 3 to the 3D framework in 1. As a result, different structures of 1–4 were obtained.
In our previous studies, the combination of M(II)-tib with p-H2BDC or m-H2BDC resulted in formation of series of M(II)-MOFs.28 Comparing with these, the substituent groups of dicarboxylate ligands can influence the structures of MOFs in two aspects. On one hand, they may possess the ability to involve interactions such as coordination, hydrogen bonding and aromatic stacking. For example, we recently reported a 2D MOF [Zn2(tib)(p-BDC-NH2)SO4(H2O)2]·5H2O, in which the nitrogen atom of NH2 in p-BDC-NH2 participates in the coordination with Zn(II).16 On the other hand, inert substituent groups (–NO2, CH3, –OH, –Br) may impose remarkable steric and/or electronic effects on the binding properties of the ligands. For instance, using tib and m-H2BDC as the mixed-ligands, [Zn(tib)(m-BDC)]·H2O and [Zn2(tib)(m-BDC)2(H2O)]·2H2O are 2D rectangular grid network with (4, 4) topology and trinodal (3, 4)-connected net with topology of (83)2(86),28a respectively. When the –OH and –CH3 was introduced to m-H2BDC, 2–4 with different structures and topologies were isolated. In addition, Wang et al. reported Cd(II)-MOFs constructed from tib and p-H2BDC-Br4, owing to the steric effect of –Br groups 3D self-penetrating framework was obtained.28d The results show that the substituent groups and solvents as well as counter anion have remarkable influence on the structures of 1–4, which provides an approach toward tuning structural diversity of MOFs by modifying the substituent groups of the auxiliary carboxylate ligands.15
Powder X-ray diffraction (PXRD) and thermal stability
The phase purity of the as-synthesized samples was further confirmed by PXRD measurements and each PXRD pattern of the as-synthesized sample is consistent with the simulated one (Fig. S6, ESI†), indicating the phase purity of the products.
Thermogravimetric analyses (TGA) of 1–4 were carried out under nitrogen atmosphere. As shown in Fig. S7 (ESI†), a weight loss of 3.42% was found before 315 °C in 1 due to the release of lattice water molecules (calcd 3.07%), and the residue is stable up to 360 °C. Complex 2 loses 8.35% of weight in the temperature range of 50–195 °C, which is attributed to the departure of the free water molecules (calcd 8.67%), and the residue can stable up to about 320 °C. Complex 3 shows a weight loss of 8.61% at 50–130 °C, corresponding to the loss of lattice water molecules (calcd 8.70%), and the decomposition of the framework occurred about at 360 °C. For 4, the TG curve displays a weight losses of 25.94% before 220 °C, suggesting the loss of the free water molecules (calcd 25.88%), and the framework is stable up to about 310 °C.
Ferroelectric property of complex 1
Since complex 1 crystallizes in the noncentrosymmetric space group (P41), which belongs to the polar point group (C4), one of the ten polar point groups (C1, Cs, C2, C2v, C3, C3v, C4, C4v, C6, C6v), required for ferroelectric behavior, the dielectrical hysteresis loop of 1 was measured at room temperature.29 Experimental result indicates that 1 displays typical ferroelectric behavior. Fig. 5 clearly shows there is electric hysteresis loop in 1 with a remnant polarization (Pr) of ca. 6.65 μC cm−2 and electric coercive field (Ec) of ca. 6.39 kV cm−1, which is comparable with that of the reported sample of [Cd(tib)(BDC)]·2H2O.28c While the remnant polarization value is significantly higher than that for the typical ferroelectric compounds.29a–c The saturation spontaneous polarization (Ps) of 1 is about 11.65 μC cm−2.
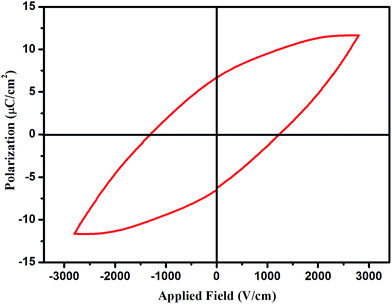 |
| Fig. 5 Polarization versus applied electric field curve (electric hysteresis loop) of 1. | |
Photoluminescence property
The complexes constructed by d10 metal centers and conjugated organic linkers are promising candidates for photoactive materials with potential applications such as chemical sensors, white light-emitting diodes (LEDs), and electroluminescent materials.30 Thus, the solid-state luminescent emission spectra of 1–4 were investigated at ambient temperature. An intense emission band was observed at 405 nm for free tib with excitation at 360 nm.31 As depicted in Fig. 6, intense luminescence emission bands were observed with peaks at 435 nm (λex = 360 nm) for 1, 419 nm (λex = 354 nm) for 2, 420 nm (λex = 337 nm) for 3, 422 nm (λex = 317 nm) for 4. The emissions of 1–4 are tentatively attributed to the tib ligand emission due to their close resemblance of the emission bands,32 since the emissions of the carboxylate ligands are usually weak compared to that of tib, and it was considered that the carboxylate ligands have no significant contribution to the fluorescence emission of 1–4 with the presence of the N-donor ligand.33 Compared with the free tib ligands, the red-shift of the emissions of 1–4 are considered to be caused by the coordination of the ligand to metal centers.34
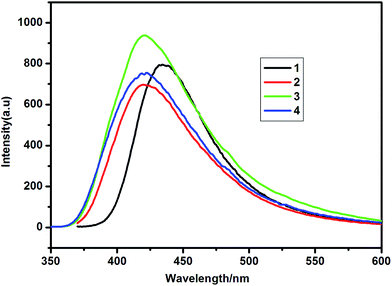 |
| Fig. 6 Emission spectra of 1–4 in the solid state at room temperature. | |
Conclusion
In this work, four new Cd(II)-based MOFs constructed from a neutral rigid tripodal ligand 1,3,5-tris(1-imidazolyl)benzene and p-H2BDC-R, m-H2BDC-R ligands with different functionalized groups have been obtained under solvothermal conditions. The complexes display structural diversity and topologies. Hence, this work further implies that the substituent groups of ligand play an important role in formation of MOFs with diverse structures. The way to introduce substituent groups to induce complicated structures may enrich the concept of the rational design and construction of functional MOFs with interesting properties.
Acknowledgements
This work was financially supported by the National Natural Science Foundation of China (grant nos 21331002 and 21401099). This work was also supported by a Project Funded by the Priority Academic Program Development of Jiangsu Higher Education Institutions.
References
-
(a) M. O'Keeffe and O. M. Yaghi, Chem. Rev., 2012, 112, 675 CrossRef PubMed;
(b) H.-X. Deng, S. Grunder, K. E. Cordova, C. Valente, H. Furukawa, M. Hmadeh, F. Gándara, A. C. Whalley, Z. Liu, S. Asahina, H. Kazumori, M. O'Keeffe, O. Terasaki, J. F. Stoddart and O. M. Yaghi, Science, 2012, 336, 1018 CrossRef CAS PubMed;
(c) Y. Kang, F. Wang, J. Zhang and X.-H. Bu, J. Am. Chem. Soc., 2012, 134, 17881 CrossRef CAS PubMed;
(d) H.-L. Jiang, D.-W. Feng, T.-F. Liu, J.-R. Li and H.-C. Zhou, J. Am. Chem. Soc., 2012, 36, 14690 CrossRef PubMed.
-
(a) Q.-K. Liu, J.-P. Ma and Y.-B. Dong, J. Am. Chem. Soc., 2010, 132, 7005 CrossRef CAS PubMed;
(b) P.-Y. Wu, C. He, J. Wang, X.-J. Peng, X.-Z. Li, Y.-L. An and C.-Y. Duan, J. Am. Chem. Soc., 2012, 134, 1499 Search PubMed;
(c) N. Yanai, T. Uemura, M. Inoue, R. Matsuda, T. Fukushima, M. Tsujimoto, S. Isoda and S. Kitagawa, J. Am. Chem. Soc., 2012, 134, 4501 CrossRef CAS PubMed.
-
(a) L.-Q. Ma, C. Abney and W.-B. Lin, Chem. Soc. Rev., 2009, 38, 1248 RSC;
(b) F.-J. Song, C. Wang, J. M. Falkowski, L.-Q. Ma and W.-B. Lin, J. Am. Chem. Soc., 2010, 132, 15390 CrossRef CAS PubMed;
(c) M. Yoon, R. Srirambalaji and K. Kim, Chem. Rev., 2012, 112, 196 CrossRef PubMed;
(d) Y. Zhao, K. Chen, J. Fan, T. Okamura, Y. Lu, L. Luo and W.-Y. Sun, Dalton Trans., 2014, 2252 RSC.
-
(a) M. M. Wanderley, C. Wang, C.-D. Wu and W.-B. Lin, J. Am. Chem. Soc., 2012, 134, 9050 CrossRef CAS PubMed;
(b) Y.-J. Cui, Y.-F. Yue, G.-D. Qian and B.-L. Chen, Chem. Rev., 2012, 112, 1126 CrossRef CAS PubMed;
(c) L. Luo, K. Chen, Q. Liu, Y. Lu, T. Okamura, G.-C. Lv, Y. Zhao and W.-Y. Sun, Cryst. Growth Des., 2013, 13, 2312 CrossRef CAS.
-
(a) B. Zhao, X.-Y. Chen, P. Cheng, D.-Z. Liao, S.-P. Yan and Z.-H. Jiang, J. Am. Chem. Soc., 2004, 126, 15394 CrossRef CAS PubMed;
(b) Z. Su, M. Chen, T. Okamura, M.-S. Chen, S.-S. Chen and W.-Y. Sun, Inorg. Chem., 2011, 50, 985 CrossRef CAS PubMed;
(c) L. E. Kreno, K. Leong, O. K. Farha, M. Allendorf, R. P. V. Duyne and J. T. Hupp, Chem. Rev., 2012, 112, 1105 CrossRef CAS PubMed.
-
(a) X.-L. Zhao and W.-Y. Sun, CrystEngComm, 2014, 16, 3247 RSC;
(b) Y.-X. Sun and W.-Y. Sun, Chin. Chem. Lett., 2014, 25, 823 CrossRef CAS PubMed;
(c) C. Pettinari, N. Masciocchi, L. Pandolfo and D. Pucci, Chem.–Eur. J., 2010, 16, 1106 CrossRef CAS PubMed;
(d) M. Chen, S.-S. Chen, T. Okamura, Z. Su, M.-S. Chen, Y. Zhao, W.-Y. Sun and N. Ueyama, Cryst. Growth Des., 2011, 11, 1901 CrossRef CAS;
(e) Y. Zhang, B.-B. Guo, L. Li, S.-F. Liu and G. Li, Cryst. Growth Des., 2013, 13, 367 CrossRef CAS.
-
(a) M. Du, Z.-H. Zhang, Y.-P. You and X.-J. Zhao, CrystEngComm, 2008, 10, 306 RSC;
(b) J. Chen, C.-P. Li and M. Du, CrystEngComm, 2011, 13, 1885 RSC;
(c) V. Colombo, C. Montoro, A. Maspero, G. Palmisano, N. Masciocchi, S. Galli, E. Barea and J. A. R. Navarro, J. Am. Chem. Soc., 2012, 134, 12830 CrossRef CAS PubMed.
- M. Eddaoudi, J. Kim, N. Rosi, D. Vodak, J. Wachter, M. O'Keeffe and O. M. Yaghi, Science, 2002, 295, 469 CrossRef CAS PubMed.
-
(a) Z. Wang and S. M. Cohen, J. Am. Chem. Soc., 2007, 129, 12368 CrossRef CAS PubMed;
(b) K. K. Tanabe and S. M. Cohen, Angew. Chem., Int. Ed., 2009, 48, 7424 CrossRef CAS PubMed;
(c) K. L. Mulfort, O. K. Farha, C. L. Stern, A. A. Sarjeant and J. T. Hupp, J. Am. Chem. Soc., 2009, 131, 3866 CrossRef CAS PubMed;
(d) M. Kim, J. A. Boissonnault, P. V. Dau and S. M. Cohen, Angew. Chem., Int. Ed., 2011, 50, 12193 CrossRef CAS PubMed;
(e) S. M. Cohen, Chem. Rev., 2012, 112, 970 CrossRef CAS PubMed;
(f) H.-L. Jiang, D. Feng, T.-F. Liu, J.-R. Li and H.-C. Zhou, J. Am. Chem. Soc., 2012, 134, 14690 CrossRef CAS PubMed.
-
(a) Z. Wang and S. M. Cohen, Chem. Soc. Rev., 2009, 38, 1315 RSC;
(b) K. K. Tanabe and S. M. Cohen, Chem. Soc. Rev., 2011, 40, 498 RSC.
- H. Deng, C. J. Doonan, H. Furukawa, R. B. Ferreira, J. Towne, C. B. Knobler, B. Wang and O. M. Yaghi, Science, 2010, 327, 846 CrossRef CAS PubMed.
-
(a) C. Volkringer and S. M. Cohen, Angew. Chem., Int. Ed., 2010, 49, 4644 CrossRef CAS PubMed;
(b) S. Biswas, T. Ahnfeldt and N. Stock, Inorg. Chem., 2011, 50, 9518 CrossRef CAS PubMed.
- M. Kim, J. A. Boissonnault, C. A. Allen, P. V. Dau and S. M. Cohen, Dalton Trans., 2012, 6277 RSC.
-
(a) S. J. Garibay and S. M. Cohen, Chem. Commun., 2010, 46, 7700 RSC;
(b) M. Kandiah, M. H. Nilsen, S. Usseglio, S. Jakobsen, U. Olsbye, M. Tilset, C. Larabi, E. A. Quadrelli, F. Bonino and K. P. Lillerud, Chem. Mater., 2010, 22, 6632 CrossRef CAS.
-
(a) C.-P. Li, Q. Yu, Z.-H. Zhang and M. Du, CrystEngComm, 2010, 12, 834 RSC;
(b) C.-P. Li, J. Chen, Q. Yu and M. Du, Cryst. Growth Des., 2010, 10, 1623 CrossRef CAS;
(c) F. Guo, B.-Y. Zhu, M.-L. Liu, X.-L. Zhang, J. Zhang and J.-P. Zhao, CrystEngComm, 2013, 15, 6191 RSC;
(d) M. Du, C.-P. Li, C.-S. Liu and S.-M. Fang, Coord. Chem. Rev., 2013, 257, 1282 CrossRef CAS PubMed;
(e) Z. Yin, Y.-L. Zhou, M.-H. Zeng and M. Kurmoo, Dalton Trans., 2015, 5258 RSC.
- J.-A. Hua, Y. Zhao, Q. Liu, D. Zhao, K. Chen and W.-Y. Sun, CrystEngComm, 2014, 16, 7536 RSC.
-
(a) J. Fan, L. Gan, H. Kawaguchi, W.-Y. Sun, K.-B. Yu and W.-X. Tang, Chem.–Eur. J., 2003, 9, 3965 CrossRef CAS PubMed;
(b) J. Fan, W.-Y. Sun, T. Okamura, W.-X. Tang and N. Ueyama, Inorg. Chem., 2003, 42, 3168 CrossRef CAS PubMed;
(c) W. Zhao, Y. Song, T. Okamura, J. Fan, W.-Y. Sun and N. Ueyama, Inorg. Chem., 2005, 44, 3330 CrossRef CAS PubMed.
- SAINT, Program for Data Extraction and Reduction, Bruker AXS, Inc., Madison, WI, 2001 Search PubMed.
- G. M. Sheldrick, SADABS, University of Göttingen, Göttingen, Germany Search PubMed.
- G. M. Sheldrick, SHELXS-97, Program for the Crystal Structure Solution, University of Göttingen, Göttingen, Germany, 1997 Search PubMed.
-
(a) Y.-F. Bi, S.-C. Du and W.-P. Liao, Chem. Commun., 2011, 47, 4724 RSC;
(b) Y.-F. Bi, X.-T. Wang, W.-P. Liao, X.-F. Wang, X.-W. Wang, H.-J. Zhang and S. Gao, J. Am. Chem. Soc., 2009, 131, 11650 CrossRef CAS PubMed;
(c) C. P. Pradeep, M. F. Misdrahi, F.-Y. Li, J. Zhang, L. Xu, D.-L. Long, T.-B. Liu and L. Cronin, Angew. Chem., Int. Ed., 2009, 48, 8309 CrossRef CAS PubMed.
-
(a) J.-J. Jang, L. Li, T. Yang, D.-B. Kuang, W. Wang and C.-Y. Su, Chem. Commun., 2009, 2387 RSC;
(b) P. Cui, Z. Chen, D.-L. Gao, B. Zhao, W. Shi and P. Cheng, Cryst. Growth Des., 2010, 10, 4370 CrossRef CAS;
(c) H.-Y. He, J.-M. Dou, D.-C. Li, H.-Q. Ma and D.-F. Sun, CrystEngComm, 2011, 13, 1509 RSC;
(d) C. J. Adams, M. F. Haddow, M. Lusi and A. Guy Orpen, CrystEngComm, 2011, 13, 4324 RSC;
(e) X.-Y. Lu, J.-W. Ye, W. Li, W.-T. Gong, L.-J. Yang, Y. Lin and G.-L. Ning, CrystEngComm, 2012, 14, 1337 RSC.
- A. T. Balaban, From Chemical Topology to Three-Dimensional Geometry, Plenum Press, New York, 1997 Search PubMed.
-
(a) V. A. Blatov, IUCr CompComm Newsletter, 2006, 7, 4;
(b) V. A. Blatov, TOPOS, A Multipurpose Crystallochemical Analysis with the Program Package, Samara State University, Russia, 2009 Search PubMed;
(c) V. A. Blatov, A. P. Shevchenko and D. M. Proserpio, Cryst. Growth Des., 2014, 14, 3576 CrossRef CAS.
-
(a) S.-S. Chen, J. Fan, T. Okamura, M.-S. Chen, Z. Su, W.-Y. Sun and N. Ueyama, Cryst. Growth Des., 2010, 10, 812 CrossRef CAS;
(b) Y.-P. He, Y.-X. Tan and J. Zhang, CrystEngComm, 2012, 14, 6359 RSC;
(c) H. Xu, W.-W. Bao, Y. Xu, X.-L. Liu, X. Shen and D.-R. Zhu, CrystEngComm, 2012, 14, 5720 RSC.
-
(a) M. O'Keeffe, M. A. Peskov, S. J. Ramsden and O. M. Yaghi, Acc. Chem. Res., 2008, 41, 1782 CrossRef PubMed;
(b) M. O'Keeffe and O. M. Yaghi, Chem. Rev., 2012, 112, 675 CrossRef PubMed;
(c) M. Li, D. Li, M. O'Keeffe and O. M. Yaghi, Chem. Rev., 2014, 114, 1343 CrossRef CAS PubMed.
- D. Rankine, A. Avellaneda, M. R. Hill, C. J. Doonan and C. J. Sumby, Chem. Commun., 2012, 48, 10328 RSC.
-
(a) Z. Su, J. Xu, J. Fan, D.-J. Liu, Q. Chu, M.-S. Chen, S.-S. Chen, G.-X. Liu, X.-F. Wang and W.-Y. Sun, Cryst. Growth Des., 2009, 9, 2801 CrossRef CAS;
(b) Z. Su, J. Fan, T. Okamura, M.-S. Chen, S.-S. Chen, W.-Y. Sun and N. Ueyama, Cryst. Growth Des., 2010, 10, 1911 CrossRef CAS;
(c) Z. Su, M.-S. Chen, J. Fan, M. Chen, S.-S. Chen, L. Luo and W.-Y. Sun, CrystEngComm, 2010, 12, 2040 RSC;
(d) L. Wang, Z.-H. Yan, Z.-Y. Xiao, D. Guo, W.-Q. Wang and Y. Yang, CrystEngComm, 2013, 15, 5552 RSC;
(e) Z. Su, Z.-B. Wang and W.-Y. Sun, Inorg. Chem. Commun., 2010, 13, 1278 CrossRef CAS PubMed.
-
(a) Q. Ye, Y.-M. Song, G.-X. Wang, K. Chen, D.-W. Fu, P. W. H. Chan, J.-S. Zhu, S.-D. Huang and R.-G. Xiong, J. Am. Chem. Soc., 2006, 128, 6555 Search PubMed;
(b) S. I. Ohkoshi, H. Tokoro, T. Matsuda, H. Takahashi, H. Irie and K. Hashimoto, Angew. Chem., Int. Ed., 2007, 46, 3238 CrossRef CAS PubMed;
(c) K. Nakagawa, H. Tokoro and S. Ohkoshi, Inorg. Chem., 2008, 47, 10810 CrossRef CAS PubMed;
(d) T. Okubo, R. Kawajiri, T. Mitani and T. Shimoda, J. Am. Chem. Soc., 2005, 127, 17598 CrossRef CAS PubMed;
(e) C.-F. Wang, D.-P. Li, X. Chen, X.-M. Li, Y.-Z. Li, J.-L. Zuo and X.-Z. You, Chem. Commun., 2009, 6940 RSC;
(f) W. Zhang and R.-G. Xiong, Chem. Rev., 2012, 112, 1163 CrossRef CAS PubMed.
-
(a) G. D. Santis, L. Fabbrizzi, M. Licchelli, A. Poggi and A. Taglietti, Angew. Chem., Int. Ed., 1996, 35, 202 CrossRef PubMed;
(b) V. W. W. Yam and K. K. W. Lo, Chem. Soc. Rev., 1999, 28, 323 RSC;
(c) J. E. McGarrah, Y. J. Kim, M. Hissler and R. Eisenberg, Inorg. Chem., 2001, 40, 4510 CrossRef CAS;
(d) J.-C. Dai, X.-T. Wu, Z.-Y. Fu, C.-P. Cui, S.-M. Hu, W.-X. Du, L.-M. Wu, H.-H. Zhang and R.-Q. Sun, Inorg. Chem., 2002, 41, 1391 CrossRef CAS PubMed.
-
(a) L. Li, J. Fan, T. Okamura, Y.-Z. Li, W.-Y. Sun and N. Ueyama, Supramol.
Chem., 2004, 16, 361 CAS;
(b) L. Zhang, Z.-J. Li, Q.-P. Lin, Y.-Y. Qin, J. Zhang, P.-X. Yin, J.-K. Cheng and Y.-G. Yao, Inorg. Chem., 2009, 48, 6517 CrossRef CAS PubMed.
-
(a) X.-L. Wang, Y.-F. Bi, G.-C. Liu, H.-Y. Lin, T.-L. Hu and X.-H. Bu, CrystEngComm, 2008, 10, 349 RSC;
(b) L. Zhang, Z.-J. Li, Q.-P. Lin, Y.-Y. Qin, J. Zhang, P.-X. Yin, J.-K. Cheng and Y.-G. Yao, Inorg. Chem., 2009, 48, 6517 CrossRef CAS PubMed.
- Y.-W. Li, H. Ma, Y.-Q. Chen, K.-H. He, Z.-X. Li and X.-H. Bu, Cryst. Growth Des., 2012, 12, 189 CAS.
-
(a) M. D. Allendorf, C. A. Bauer, R. K. Bhakta and R. J. T. Houk, Chem. Soc. Rev., 2009, 38, 1330 RSC;
(b) G.-Z. Liu, S.-H. Li, X.-L. Li, L.-Y. Xin and L.-Y. Wang, CrystEngComm, 2013, 15, 4571 RSC;
(c) H.-R. Fu, F. Wang and J. Zhang, Dalton Trans., 2014, 4668 RSC.
Footnote |
† Electronic supplementary information (ESI) available: Selected bond lengths and angles for 1–4 (Table S1), hydrogen bonding data for 4 (Table S2), Cd(II)-tib networks in 1 and 2 (Fig. S1), building units in 1–4 (Fig. S2), coordination modes of tib in 2 and 3 (Fig. S3), illustrations of 3D net of 2 and 3 (Fig. S4), 3D structure of 4 (Fig. S5), PXRD (Fig. S6) and TGA (Fig. S7). CCDC 1057692–1057695. For ESI and crystallographic data in CIF or other electronic format see DOI: 10.1039/c5ra06072a |
|
This journal is © The Royal Society of Chemistry 2015 |
Click here to see how this site uses Cookies. View our privacy policy here.