DOI:
10.1039/C5RA05604G
(Paper)
RSC Adv., 2015,
5, 41401-41411
The importance of Au⋯π(aryl) interactions in the formation of spherical aggregates in binuclear phosphane gold(I) complexes of a bipodal thiocarbamate dianion: a combined crystallographic and computational study, and anti-microbial activity†
Received
30th March 2015
, Accepted 21st April 2015
First published on 22nd April 2015
Abstract
Binuclear phosphanegold(I) complexes of a bipodal thiocarbamate dianion, (R3PAu)2L, R = Et (1), Ph (2) and Cy (3), where LH2 is {1,4-[MeOC(
S)N(H)]2C6H4}, have been synthesised, and characterised spectroscopically (NMR and IR) and by X-ray crystallography. The gold atoms are linearly coordinated within a P-,S-donor set, and are oriented toward the central ring to form intramolecular Au⋯π(aryl) interactions, rather than the intramolecular Au⋯O interactions normally observed in mononuclear analogues. This phenomenon has been investigated by theory (LC-ωPBE-XDM) for 1 which revealed that the geometry optimised species with two Au⋯π(aryl) interactions is more stable by at least 12 kcal mol−1 compared to conformations having one or more Au⋯O interactions instead. The disk diffusion, minimum inhibitory concentration (MIC) and minimum bactericidal concentration (MBC) methods were used to observe the inhibitory effect of complexes 1–3. The disk diffusion results demonstrated that 1 exhibited a broad spectrum of anti-bacterial activity toward 24 strains of Gram-positive and Gram-negative bacteria. By contrast, the anti-bacterial activity of 2 and 3 was limited to Gram-positive bacteria. Further evaluation showed that 1 exhibited marked bactericidal activity against B. cereus, B. subtilis, E. faecalis, L. monocytogenes, S. aureus, S. saprophyticus and methicillin resistant S. aureus cf. standard antibiotics tetracycline and chloramphenicol.
Introduction
The structural diversity and the fascinating architectures they sustain have ensured that aurophilic (Au⋯Au) interactions have garnered the attention of chemists for decades.1 The aggregation of gold compounds in this fashion arises from relativistic effects which are at a maximum for gold compared to other heavy elements.2 Over and above aesthetics, interest in gold compounds/aurophilic interactions stems from their luminescence properties, in both the solid-state and in solution.3 By contrast to aurophilic interactions, the recognition of supramolecular association based on Au⋯π(aryl) interactions is still in its infancy,4a despite them being often crucial in the reaction mechanisms related to the burgeoning field of gold-based catalysis.5 It is only in recent years that bibliographic surveys have shown that Au⋯π(aryl) interactions provide readily identifiable points of contact between molecules in their crystal structures, operating in isolation of other supramolecular synthons.4
The themes of aurophilic and Au⋯π(aryl) interactions as well as luminescence feature prominently in the chemistry of phosphanegold(I) thiocarbamates, where the thiolate anion is derived from thiocarbamide molecules of the general formula RN(H)C(
S)OR′, R,R′ = alkyl and/or aryl.6 In the overwhelming majority of crystal structures of phosphanegold(I) thiocarbamates, the thiolate ligand is oriented so that the oxygen atom is located in close proximity to the gold atom. However, by careful choice of R and R′ substituents of the thiolate anion and by using isomeric tolylphosphane ligands, it proved possible to orient the thiolate so that the aryl ring was proximate to the gold atom instead.6c Crystal engineering considerations notwithstanding, phosphanegold(I) thiocarbamates also exhibit promising biological activity.
Gold(I) compounds are used clinically in the treatment of severe forms of rheumatoid arthritis, being a member of the disease modifying anti-rheumatic drugs (DMARDS) class of anti-arthritic agents.7a–c Spurred by the observation that patients undergoing chrysotherapy, appeared to suffer reduced rates of malignant disease,7d both gold(I) and gold(III) compounds are under intense investigation as potential anti-tumour drugs.8 In the context of the present study, phosphanegold(I) thiocarbamates of the general formula Ph3PAu[SC(OR)
NPh], R = Me, Et and iPr, exhibit significant cytotoxicity toward HT-29 colon cancer cells, have been shown to function through both intrinsic and extrinsic apoptotic pathways, and to be topoisomerase I inhibitors.9 Gold compounds are also under active evaluation as anti-microbial agents.10 Recently, another series of phosphanegold(I) thiocarbamates, namely Ph3PAu[SC(OR)
N(p-tol)], R = Me, Et and iPr, have proven to be very effective against a panel of Gram-positive bacteria.11
It was primarily the biological context that led to the synthesis of the title binuclear compounds, (R3PAu)2L, R = Et (1), Ph (2) and Cy (3), where LH2 is the bipodal molecule {1,4-[MeOC(
S)N(H)]2C6H4}. Herein, their characterization, including by X-ray crystallography and Density Functional Theory (DFT) calculations is described along with an evaluation of their potential anti-microbial activity.
Experimental section
Materials, methods and Instruments
All chemicals and solvents were used as purchased without purification. All reactions were carried out under ambient conditions. Elemental analyses were performed on a Perkin Elmer PE 2400 CHN Elemental Analyser. Melting points were determined on a Krüss KSP1N melting point meter. 1H and 13C{1H} NMR spectra were recorded in both DMSO-d6 and CDCl3 solutions for LH2, and CDCl3 solutions for 1–3 on a Bruker Avance 400 MHz NMR spectrometer with chemical shifts relative to tetramethylsilane. 31P{1H} NMR spectra were recorded in CDCl3 solution on the same instrument but with the chemical shifts recorded relative to 85% aqueous H3PO4 as the external reference; abbreviations for NMR assignments: s, singlet; d, doublet; m, multiplet; dq, doublet of quartets; dt, doublet of triplets. For the relaxation experiments on 1, a solution of the compound (20 mg) was prepared in CDCl3 (4.5 cm) in a 5 mm NMR tube (Wilmad). The solution was degassed through five freeze–pump–thaw cycles and sealed under vacuum. The NMR measurements were carried out on a JEOL ECX500 NMR spectrometer. The 13C spin–lattice relaxation time (T1) was obtained by using the two-pulse inversion–recovery (IR) method.12 Initially, an approximate T1 value was obtained before a more precise measurement was made using 10 IR intervals in a range up to 1.5T1. Subsequently, a final measurement was made to confirm the value T1 = 0.69 s at 20 °C. Then, the NOE measurements were made in two consecutive experiments: one with complete proton decoupling with a relaxation delay of 6.0 s and an acquisition time of 1.04 s, and the other experiment with gated-decoupling in which the NOE was suppressed but with the remaining conditions the same as in the first experiment. The pulse angle was 90°. Good signal intensity was obtained with 1024 scans. The total integrated intensity of the signal was obtained with the spectrometer's data processing software. The ratio of the signal intensities in the two experiments gives the NOE value, usually given as NOE = 1 + η, where η is the NOE enhancement factor. IR spectra were measured on a Perkin Elmer Spectrum 400 FT Mid-IR/Far-IR spectrophotometer from 4000 to 400 cm−1; abbreviations: vs, very strong; s, strong; m, medium; br, broad. Thermogravimetric analyses were performed on a Perkin Elmer TGA 4000 Thermogravimetric Analyzer in the range of 35–850 °C at the rate of 10 °C min−1. Powder X-ray diffraction (PXRD) data were recorded with a PANalytical Empyrean XRD system with Cu-Kα1 radiation (λ = 1.54056 Å) in the 2θ range 5 to 50°. The comparison between experimental and calculated (from CIF's) PXRD patterns were performed with X'Pert HighScore Plus.13
Synthesis of LH2. LH2 was prepared in similar manner as described in the literature for mono-functional analogues14 whereby p-phenylene diisothiocyanate (2.50 mmol, 0.48 g; Sigma-Aldrich) was reacted in MeOH (100 ml) in the presence of two mole equivalents of NaOH, yielding a pale-yellow powder. Yield: 0.596 g (93%). mp 209.0–210.0 °C. Anal. calc. for C10H12N2O2S2: C, 46.85; H, 4.72; N, 10.93. Found: C, 46.71; H, 4.64; N, 10.94%. IR (ν, cm−1): 3219 (br) ν(N–H), 1454 (s) ν(C–N), 1140 (s) ν(C–O), 1046 (s) ν (C
S). 1H NMR (DMSO-d6): δ 11.10 [s, br, 2H, NH], 7.59 [s, br, 2H, aryl-H], 7.30 [s, br, 2H, aryl-H], 3.99 [s, 6H, OCH3] ppm. 1H NMR (CDCl3): δ 8.32 [s, br, 2H, NH], 7.56 [s, br, 2H, aryl-H], 7.22 [s, br, 2H, aryl-H], 4.12 [s, 6H, OCH3] ppm. 13C{1H} NMR (DMSO-d6): δ 189.1, 188.4 [Cq], 135.8, 134.8 [Cipso], 123.7 and 122.6 [Cortho], 58.3, 56.8 [OCH3] ppm.
Synthesis of (R3PAu)2L, R = Et (1), Ph (2) and Cy (3). The methods employed for the preparation and recrystallization of 1–3 were similar, so the preparation of the Et3P derivative (1) is described in detail as a representative example. The R3PAuCl, R = Et, Ph and Cy, precursors employed in the synthesis were prepared following standard procedures by reducing KAuCl4 (Sigma-Aldrich) using one mole excess sodium sulfite (Merck), and then further reacted with one mole equivalent of either Et3P (Merck), Ph3P (Merck) or Cy3P (Sigma-Aldrich), to yield the respective precursor.
(Et3PAu)2L (1). NaOH (0.50 mmol) in water (5 ml) was added to a suspension of Et3PAuCl (0.50 mmol) in acetonitrile (20 ml) followed by addition of LH2 (0.25 mmol) in acetonitrile (20 ml). The resulting mixture was stirred for 3 h at 50 °C. Extraction followed with dichloromethane (100 ml) and an equivalent volume of acetonitrile added. The solution was left for slow evaporation at room temperature, giving colourless crystals after 2 weeks. Yield: 0.206 g (93%). mp 174.0–176.0 °C. Anal. calc. for C22H40Au2N2O2P2S2: C, 29.87; H, 4.56; N, 3.17. Found: C, 29.92; H, 4.53; N, 3.04%. IR (cm−1): 1421 (m) ν(C
N), 1123 (vs) ν(C–O), 1094 (s) ν(C–S). 1H NMR (CDCl3): δ 6.72 [s, 4H, aryl-H], 3.81 [s, 6H, OCH3], 1.70 [dq, 12H, CH2P, 3JHH = 7.75, 2JPH = 9.82 Hz], 1.06 [dt, 18H, CH3CH2P, 3JHH = 7.62, 3JPH = 18.32 Hz] ppm. 13C{1H} NMR (CDCl3): δ 165.3 [Cq], 145.7 [aryl, Cipso], 122.8 [aryl, Cortho], 55.2 [OCH3], 17.6 [d, CH2P 1JCP = 33.3 Hz], 8.8 [s, CH3CH2P] ppm. 31P{1H} NMR (CDCl3): δ 35.6 ppm.
(Ph3PAu)2L (2). A similar synthetic procedure as employed for 1 was used for the synthesis of 2 except that the gold precursor was replaced by Ph3PAuCl. Colourless crystals. Yield: 0.255 g (87%). mp 181.5–183.0 °C. Anal. calc. for C46H40Au2N2O2P2S2: C, 47.11; H, 3.44; N, 2.39. Found: C, 47.25; H, 3.31; N, 2.41%. IR (cm−1): 1434 (s) ν(C
N), 1143 (s) ν(C–O), 1101 (s) ν(C–S). 1H NMR (CDCl3): δ 7.49–7.41 [m, 30H, Ph3P], 6.41 [s, 4H, aryl-H], 3.73 [s, 6H, OCH3] ppm. 13C{1H} NMR (CDCl3): δ 163.2 [Cq], 145.7 [aryl, Cipso], 134.4 [d, o-Ph3P, 2JCP = 13.9 Hz], 131.4 [d, p-Ph3P, 4JCP = 2.2 Hz], 129.6 [d, i-Ph3P, JCP = 57.0 Hz], 128.9 [d, m-Ph3P, 3JCP = 11.5 Hz], 122.4 [aryl, Cortho], 55.0 [OCH3] ppm. 31P{1H}NMR (CDCl3): δ 38.1 ppm.
(Cy3PAu)2L (3). The synthesis was as for 1 but using Cy3PAuCl. Colourless crystals. Yield: 0.275 g (91%). mp 180.0–181.0 °C. Anal. calc. for C46H76Au2N2O2P2S2: C, 45.69; H, 6.34; N, 2.32. Found: C, 45.57; H, 6.40; N, 2.24%. IR (cm−1): 1444 (s) ν(C
N), 1130 (s) ν(C–O), 1093 (m) ν(C–S). 1H NMR (CDCl3): δ 6.84 [s, 4H, aryl-H], 3.82 [s, 6H, OCH3], 1.98–1.20 [m, 66H, Cy3P] ppm. 13C{1H} NMR (CDCl3): δ 163.9 [Cq], 145.4 [aryl, Cipso], 122.4 [aryl, Cortho], 54.8 [OCH3], 33.3 [d, 1-Cy3P, JCP = 27.8 Hz], 30.7 [s, 3-Cy3P], 27.0 [d, 2-Cy3P, 3JCP = 11.9 Hz], 25.9 [s, 4-Cy3P] ppm. 31P{1H}NMR (CDCl3): δ 56.5 ppm.
X-ray data collection and structure determination
Intensity data were measured at 100 K on an Agilent Technologies SuperNova Dual CCD with an Atlas detector fitted with Mo Kα radiation (λ = 0.71073 Å). Data processing and absorption correction were accomplished with CrysAlis PRO.15a With the use of SHELX programs15b integrated into WinGX,15c the structures were solved by direct methods and refined on F2 by full-matrix least-squares with anisotropic displacement parameters for all non-hydrogen atoms. The C-bound H atoms were placed on stereochemical grounds and refined in the riding model approximation with Uiso = 1.2–1.5Ueq(carrier atom). A weighting scheme of the form w = 1/[σ2(Fo2) + (aP)2 + bP] where P = (Fo2 + 2Fc2)/3 was introduced in each case. For 1, the residual electron density peaks were located 0.95 and 0.96 from the Au atom, and for 2, the peaks were 0.90 and 0.86 from the Au atom. Details of cell data, X-ray data collection and structure refinement are given in Table 1. The programs ORTEP-3 for Windows,15c PLATON,15d DIAMOND15e and QMol15f were also used in the analysis.
Table 1 Crystal data, data collection and refinement parameters for compounds 1–3
|
1 |
2 |
3 |
Formula |
C22H40Au2N2O2P2S2 |
C46H40Au2N2O2P2S2 |
C46H76Au2N2O2P2S2 |
Formula weight |
884.56 |
1172.79 |
1209.08 |
Crystal size (mm) |
0.10 × 0.20 × 0.25 |
0.20 × 0.20 × 0.30 |
0.20 × 0.30 × 0.30 |
Crystal system |
Monoclinic |
Triclinic |
Monoclinic |
Space group |
P21/c |
P![[1 with combining macron]](https://www.rsc.org/images/entities/char_0031_0304.gif) |
P21/n |
a/Å |
6.8843(6) |
8.9980(4) |
9.7522(3) |
b Å |
11.6056(7) |
9.0501(4) |
12.2227(3) |
c/Å |
17.7118(10) |
14.2542(6) |
19.9917(5) |
α/° |
90 |
107.851(4) |
90 |
β/° |
94.244(6) |
100.439(4) |
93.683(3) |
γ/° |
90 |
100.556(4) |
90 |
V/Å3 |
1411.23(17) |
1050.66(8) |
2378.05(11) |
Z |
2 |
1 |
2 |
Dx/g cm−3 |
2.082 |
1.854 |
1.689 |
μ/mm−1 |
10.667 |
7.190 |
6.355 |
θ range/° |
2.9–27.5 |
3.0–27.5 |
2.8–27.5 |
Reflections measured |
15 284 |
12 872 |
26 714 |
Independent reflections; Rint |
3218; 0.030 |
4751; 0.054 |
5408; 0.032 |
Reflections with I > 2σ(I) |
2955 |
4433 |
4894 |
Number of parameters |
149 |
254 |
254 |
R(F) [I > 2σ(I) reflections] |
0.025 |
0.024 |
0.021 |
a, b in weight scheme |
0.031, 3.103 |
0.008, 0 |
0.010, 1.698 |
wR(F2) (all data) |
0.064 |
0.044 |
0.041 |
GOF(F2) |
1.08 |
0.97 |
1.05 |
Δρmax,min (e Å−3) |
1.71, −1.46 |
1.12, −1.31 |
0.59, −0.61 |
Powder X-ray diffraction
Powder X-ray patterns (PXRD) measured on the bulk material for each of 1–3 were compared with the simulated patterns calculated based on the CIF's obtained for the experimental crystal structures.13 There is a high agreement between the patterns, ESI Fig. S1,† suggesting the single crystal results are representative of the bulk materials.
Computational study
The calculations were run using Gaussian09 (ref. 16) and the LC-ωPBE17 functional combined with the exchange-hole dipole moment (XDM) model dispersion correction,18–20 implemented in the postg program.21 In a previous contribution, it was shown that LC-ωPBE-XDM gives excellent performance in the treatment of aurophilicity,22 so it is a reasonable choice to study interactions involving gold. Three conformations were examined for 1: (i) the same conformation as in the crystal structure, with two Au⋯π(aryl) contacts, (ii) a mixed conformation with one Au⋯π(aryl) and one Au⋯O contact, i.e. where one Au⋯π(aryl) interaction of the experimental structure was replaced by a contact between gold and a thiocarbamate-O atom, and (iii) the fully extended, rod-like conformations with two Au⋯O interactions. The geometry of each conformer was relaxed using the 6-31+G* basis set for all s- and p-block elements and aug-cc-pVDZ-PP scalar relativistic pseudo-potential and basis set combination for Au.23 Despite the modest size of this basis set, it has been shown in previous work that just one set of diffuse and polarization functions is sufficient to model non-covalent interactions with an accuracy relatively close to the basis-set limit.24
Anti-microbial assay
Bacterial strains. The standard reference strains of bacteria used in the present study were Aeromonas hydrophila (A. hydrophila) ATCC 35654, Acinetobacter baumannii (A. baumannii) ATCC 19606, Bacillus cereus (B. cereus) ATCC 10876, Bacillus subtilis (B. subtilis) ATCC 6633, Citrobacter freundii (C. reundii) ATCC 8090, Enterobacter cloacae (E. cloacae) ATCC 35030, Enterobacter aerogenes (E. aerogenes) ATCC 13048, Enterococcus faecalis (E. faecalis) ATCC 29212, Enterococcus faecium (E. faecium) ATCC 19434, Escherichia coli (E. coli) ATCC 25922, Klebsiella pneumoniae (K. pneumoniae) ATCC 700603, Listeria monocytogenes (L. monocytogenes) ATCC 19117, Proteus mirabilis (P. mirabilis) ATCC 25933, Proteus vulgaris (P. vulgaris) ATCC 13315, Pseudomonas aeruginosa (P. aeruginosa) ATCC 27853, Salmonella paratyphi A (S. paratyphi A) ATCC 9150, Salmonella typhimurium (S. typhimurium) ATCC 14028, Shigella flexneri (S. flexneri) ATCC 12022, Shigella sonnei (S. sonnei) ATCC 9290, Staphylococcus aureus (S. aureus) ATCC 25923, methicillin resistant Staphylococcus aureus (MRSA) ATCC 43300, Staphylococcus saprophyticus (S. saprophyticus) ATCC 15305, Stenotrophomonas maltophilia (S. maltophilia) ATCC 13637 and Vibrio parahaemolyticus (V. parahaemolyticus) ATCC 17802. All bacterial strains were purchased from the American Type Culture Collection (ATCC, Manassas, VA, USA).
Anti-bacterial activity. The anti-bacterial activity of 1–3 was evaluated using the Kirby–Bauer disc diffusion method in accordance with the National Committee for Clinical Laboratory Standards (NCCLS) guidelines. The bacterial cultures were adjusted to 0.5 McFarland standard turbidity (which corresponds to approximately 108 CFU ml−1) by adding Mueller-Hinton broth (Difco, USA). This suspension was then swabbed onto the surface of Mueller-Hinton agar (Difco, USA) plates. The tested compounds were dissolved in DMSO to a test concentration of 2 mg ml−1. Sterile 6 mm filter paper discs were aseptically placed on Mueller-Hinton agar surfaces and 5 μl of the dissolved compounds were immediately added to discs. DMSO was used as a negative control whereas standard anti-biotic paper disc was used as the positive control. All plates were incubated at 37 °C for 24 h. The anti-bacterial activity was evaluated by measuring the diameter of the inhibition zone against the test bacterial strains. Each trial was performed in triplicate.
Minimum inhibitory concentration (MIC) and minimum bactericidal concentration (MBC) determination. The MIC was determined by the broth micro-dilution method according to the NCCLS guidelines. An inoculum suspension with a density 105 CFU ml−1 of exponentially growing bacterial cells was added into each well. The test compounds were serially two-fold diluted in DMSO and then added to each well of a 96-well microplate. The 96-well microplates were incubated at 37 °C for 24 h. All tests were performed in triplicate. Four controls comprising medium with standard anti-biotic (positive control), medium with DMSO (solvent control), medium with inoculum bacterial cells (negative control) and medium with broth only (negative growth control) were included in each test. The bacterial growth was detected by adding 50 μl of a 0.2 mg ml−1 p-iodonitrotetrazolium violet (INT) indicator solution into each of the microplate wells and incubated at 37 °C for 30 min. under aerobic agitation. The INT changed from clear to red in the presence of bacterial activity. Where bacterial growth was inhibited, the suspension in the well remained clear after incubation with INT. The lowest concentration of the tested compound which completely inhibited bacterial growth was taken as the MIC. After MIC determination of each tested compound, an aliquot of 100 μl from each well which showed no visible growth was spread onto MHA at 37 °C for 24 h. The MBC is defined as the lowest concentration of the tested compound at which bacteria are killed.
Results and discussion
Syntheses and spectroscopy
The reaction of p-phenylene di-isothiocyanate with MeOH in the presence of base yielded bipodal LH2 in high yield. The 1H and, especially, 13C NMR of LH2 showed unexpected features in that chemically equivalent sites gave multiple resonances. Accordingly, a variable temperature 1H NMR study in DMSO-d6 solution (to attain a wider temperature range) was conducted with the results summarised in Table 2. Two resonances were observed for the ring protons when equivalence might have been expected. The splitting is ascribed to the formation of intramolecular methyl-C–H⋯π(aryl) interactions rendering the protons inequivalent. Upon heating the solution, the two signals coalesced into a single resonance, most likely due to rapid exchange, ESI Fig. S2a.† While no splitting was observed for the resonance due to the methyl protons, marked sharpening of the signal was evident as manifested in the W1/2 values of 29.32 Hz at 28 °C cf. 2.29 Hz at 78 °C, indicative of a rapid exchange process, ESI Fig. S2b.† This was accompanied by a small downfield shift. The N-bound proton shifted upfield with increasing temperature consistent with reduced hydrogen bonding with the solvent. Evidence that the reduction in hydrogen bonding was a two-step process was found in the appearance of a shoulder for the methyl-proton at 68 °C consistent with the appearance of a minor, new species. The two environments arise at high temperature as one N-bound proton is involved in hydrogen bonding with the solvent and the other is not, cf. both participating in hydrogen bonding at low temperature.
Table 2 Variable temperature 1H NMR data (δ, ppm; W1/2, band width at half-height) for LH2 recorded in DMSO-d6 solution
Temp. (°C) |
NH |
aryl-H |
Ome |
W1/2 (Hz) |
28 |
11.08 |
7.59, 7.29 |
3.98 |
29.32 |
38 |
11.04 |
7.57, 7.31 |
3.98 |
12.40 |
48 |
10.99 |
7.41 |
3.98 |
6.52 |
58 |
10.95 |
7.44 |
3.99 |
4.95 |
68 |
10.90 |
7.44 |
3.99 (sh, 3.98) |
3.17 |
78 |
10.86 |
7.44 |
4.00 (sh, 3.98) |
2.29 |
Upon deprotonation and coordination of LH2, the 1H NMR resonance at 8.32 (CDCl3) ascribed to N–H no longer persists in the spectra of 1–3. By contrast to the spectrum recorded for LH2, all chemically equivalent nuclei resonated at the same field strength. Also noteworthy are the significant upfield shifts in the resonances due to aryl-H of the central ring, i.e. to 6.72, 6.41 and 6.84 for 1–3, respectively cf. 7.56 and 7.22 observed for LH2. This observation is correlated with the presence of intramolecular Au⋯π(aryl) interactions as delineated by X-ray crystallography (see below). The key finding of the 13C{1H} NMR spectra was that the four phenyl hydrogen-bearing nuclei, ortho and meta, are equivalent and appear as a single resonance in CDCl3 solution. Similarly, the quaternary ipso carbons are also equivalent. In the 31P NMR, a single resonance was observed for 1–3 at 35.6, 38.1 and 56.5, respectively, which are deshielded with respect to the uncoordinated Et3P (−20.0), Ph3P (−5.2) and Cy3P (9.2) molecules measured under the same conditions. The relaxation attributes of 1, being representative of the series, were also investigated.
In the 13C{1H} NMR spectrum of 1, the ortho and meta carbons of the central ring resonated at δ = 122.84 ppm. At 20 °C in CDCl3 solution, the observed 13C spin-lattice relaxation time (T1) for this resonance was 0.69 s, and the NOE = 2.88; the NOE enhancement factor, η, was 1.88. As this η value is very near the maximum value of 1.988 for a 13C nucleus, the dominant relaxation mechanism is the dipole–dipole relaxation mechanism (Tdd1), for which the rate of this dipolar relaxation (1/Tdd1), is proportional to the correlation time.12,25 The observed T1 is very low for phenyl nuclei in solution and is consistent with very low mobility of the phenyl group in the molecule. Hence, the observed 13C relaxation data are consistent with severe hindrance in the internal rotation of the phenyl group.
The IR spectra of compounds 1–3 confirmed the absence of broad absorption at 3219 cm−1, due to ν(N–H) in LH2. Further, the red shifts of the ν(C–N) absorption to ∼1400 cm−1, and blue shifts of ν(C
S) to ∼1100 cm−1 in 1–3 when compared to LH2 provide further evidence for complexation.
Crystallography
The crystal structures of 1–3 were established by X-ray crystallography: the molecular structures are shown in Fig. 1 and selected geometric parameters are collated in Table 3. The molecule in 1, Fig. 1a, is located about a centre of inversion and features a linear gold atom geometry defined by the phosphane-P and thiolate-S atoms. As usual for this type of structure,6 the Au–S bond length is longer than that of Au–P. As the crystal structure of the precursor thiocarbamide, LH2, is not available, the comparison between the geometric parameters defining the thiolate ligand in 1 will be made with PhN(H)C(
S)OMe.26 The most notable observations is the elongation of the C1–S1 and contraction of the C1–N1 bond lengths in 1, Table 1, compared with 1.6708(11) and 1.3288(15) Å, respectively in the uncoordinated molecule.26 These changes are consistent with L2− coordinating each gold atom as a thiolate ligand. The rearrangement of electron density in the anion also results in significant differences in bond angles whereby the S1–C1–O1 angle has contracted by about 16°, and the S1–C1–N1 and O1–C1–N1 angles have expanded by about 10 and 6°, respectively, observations readily correlated with the increased electron density in the C1–N1 bond. The molecular structures of 2 and 3 have essentially the same features as described for 1, as highlighted in the overlay diagram shown in Fig. 2 that shows only small conformational variations. According to a search of the Cambridge Structural Database (CSD),27 there are no precedents for binuclear structures based on bipodal thiocarbamates or analogues of higher denticity.
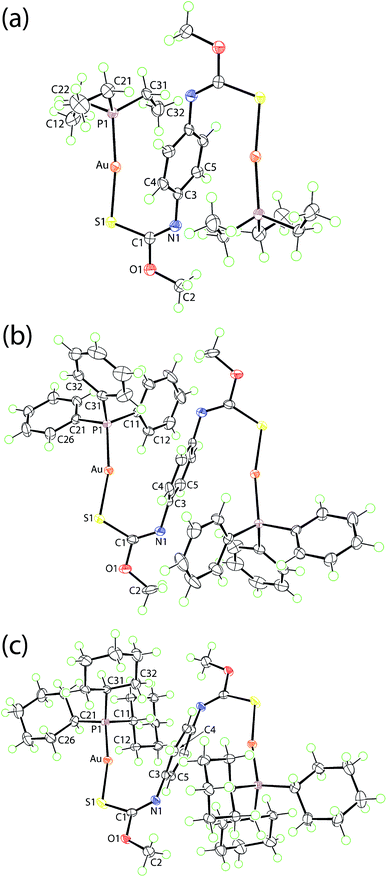 |
| Fig. 1 Perspective views of the molecules of (a) 1, (b) 2 and (c) 3. Displacement ellipsoids are drawn at the 70% probability level and hydrogen atoms are shown as small spheres of arbitrary radii. The unlabelled atoms in (a) are related by the symmetry operation 1 − x, 2 − y, −z, and in (b) and (c) by 1 − x, 1 − y, −z. | |
Table 3 Selected bond lengths (Å) and angles (°) for 1–3
|
1 |
2 |
3 |
α is the angle between the normal to the plane through the central ring and the vector passing through the centroid (Cg) of the aryl ring to the Au atom. |
Au–S1 |
2.3152(10) |
2.3058(9) |
2.3019(6) |
Au–P1 |
2.2663(10) |
2.2593(9) |
2.2695(6) |
C1–S1 |
1.762(4) |
1.761(3) |
1.756(3) |
C1–O1 |
1.372(5) |
1.356(4) |
1.362(3) |
C1–N1 |
1.258(5) |
1.268(4) |
1.263(3) |
S1–Au–P1 |
172.13(4) |
167.79(3) |
168.63(2) |
Au–S1–C1 |
107.07(14) |
107.37(12) |
111.77(9) |
C1–N1–C3 |
123.5(4) |
122.3(3) |
124.8(2) |
S1–C1–O1 |
108.5(3) |
108.1(2) |
106.82(17) |
S1–C1–N1 |
132.8(3) |
131.3(3) |
134.3(2) |
O1–C1–N1 |
118.7(4) |
120.6(3) |
118.8(2) |
Au⋯Cg(aryl)a |
3.26 |
3.32 |
3.55 |
αa |
22.7 |
21.6 |
30.7 |
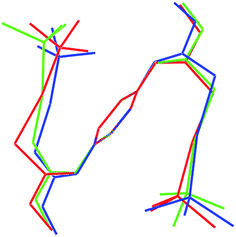 |
| Fig. 2 Overlay diagram of 1 (red image), 2 (green) and 3 (blue). No hydrogen atoms are shown and only the α-carbon atoms of the phosphane ligands are included. The molecules have been overlapped so that the central rings are coincident. | |
The crystal packing of 1 is devoid of directional intermolecular interactions and may be described as comprising columns of molecules parallel to the a-axis, ESI Fig. S3a.† Weak C–H⋯π(aryl) interactions are noted in the crystal packing of 2, and methylene-C–H⋯O contacts are seen in 3. Despite these and the disparity in space groups, Table 1, the packing for each of 2 and 3 is as described for 1, i.e. comprising columns of molecules aligned along the a-axis, ESI Fig. S3b and c.†
The linear coordination geometries for gold deviate by up to 12° from the ideal, deviations that are ascribed to the presence of intramolecular Au⋯π(aryl) interactions, Table 3. Such deviations are usually observed in related mononuclear structures of [MeOC(S)
NR]− but more often than not due to intramolecular Au⋯O interactions. Indeed, of the 31 structures available in the CSD27 with the general formula, R3PAu[SC(OMe)
NR′], and diphosphane analogues,6a,28 27 feature Au⋯O interactions in the range 2.862(3) Å for R = Ph & R′ = p-tol,6c to 3.172(8) Å for R = Cy & R′ = p-NO2Ph.6a The remaining structures, each with isomeric tol3P ligands, were reported to form intramolecular Au⋯π(aryl) interactions in the absence of steric hindrance and by judicious combinations of R and R′ groups.6c Given that in no circumstances were intramolecular Au⋯π(aryl) interactions formed for R3P ligands with R = Et, Ph or Cy, the observation of these interactions in 1–3 was unexpected. The fickle nature of the formation of Au⋯π(aryl) interactions is probably best illustrated in the polymorphs of dppm(AuCl)2 where in one form, an intramolecular Au⋯Au interaction is formed within an A-frame arrangement,29a whereas in the other polymorph, an intramolecular Au⋯π(aryl) interaction was observed instead.29b In order to probe further the nature of the Au⋯π(aryl) interactions formed in 1–3, 1 was subjected to a computational study.
Optimised molecular structures
Phosphanegold(I) thiolates sometimes present unexpected structures.30 For example, in structures of the type R3PAu[SC(
S)OR′], R,R′ = alkyl/aryl, intramolecular Au⋯O interactions are usually formed giving rise to compact structures, rather than the intramolecular Au⋯S contacts expected from hard acid/soft base considerations. The latter interactions lead to open, rod-like molecular structures.30a–c In a similar vein, in a related species, i.e. Cy3PAu[SC6H4-2-CO2H], in one polymorph the anticipated eight-membered {…HOCO}2 synthon is formed, linking molecules in the crystal structure to form rod-type conformations. However, in each of the three other polymorphs, intramolecular O–H⋯S(thiolate) hydrogen bonds are formed instead, leading to spherical molecules.30d,e The unexpected structures were rationalised in terms of global crystal packing considerations,31 in that the packing of spherical aggregates is more efficient that the packing of “rods”.30
Geometry optimisation calculations were performed on 1 with the energy minimised structure having a conformation very similar to the experimentally observed structure. As highlighted in Fig. 3, there has been a flattening in the N
C–S–Au part of the geometry optimised molecule as manifested in the N1–C1–S1–Au dihedral angle of −0.9°, cf. 14.8(5)° in the experimental structure. Conformational differences are also noted in the phosphane-bound ethyl groups. Further, the calculations show that the conformation presenting two intramolecular Au⋯π(aryl) contacts, as is experimentally observed in the crystal structure of 1, is more stable by 12.2 kcal mol−1 with respect to the conformation featuring one Au⋯π(aryl) contact and one Au⋯O contact, and by 23.6 kcal mol−1 than the rod-like conformer with two Au⋯O interactions, Fig. 4. These results shown the Au⋯π(aryl) interaction is much stronger than Au⋯O, and roughly additive.
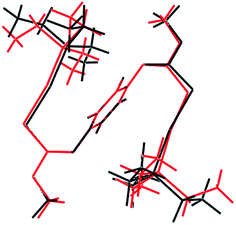 |
| Fig. 3 Overlay diagram of the experimental molecular structure of 1 (red image) and the energy minimised structure (black). The molecules have been overlapped so that the central rings are coincident. | |
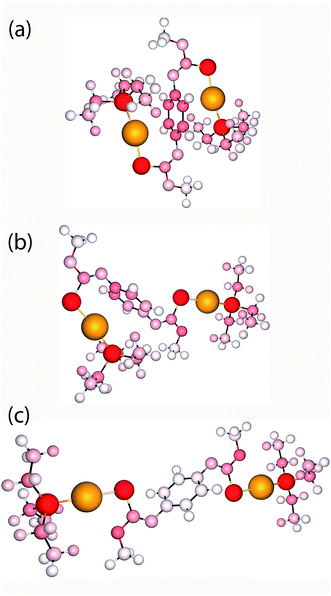 |
| Fig. 4 Calculated structures for 1: (a) the compact, spherical, conformation with two intramolecular Au⋯π(aryl) interactions, (b) intermediate structure with one Au⋯π(aryl) and one Au⋯O contact, and (c) the open, rod-like, conformation with two Au⋯O interactions. Bader′s delocalization indices between gold (in orange) and all other individual atoms are given as a colour scale ranging from zero (white) to 0.30 (red). | |
The Bader delocalization indices (DI's)32 were calculated at the LC-ωPBE-XDM level between gold and the rest of the atoms in the three studied conformations. Intermolecular DI's are an excellent tool to evaluate the degree of electron delocalization (charge transfer) between interacting fragments.33 For the conformations featuring Au⋯π(aryl) interactions, there is a significant amount of electron sharing between the gold atoms and the central ring, indicating the existence of orbital interactions between the gold atom and the π-system, and justifying the high stability of this contact, Fig. 4. The DI between the two gold atoms and the aryl ring is 0.56 in the compact conformer and 0.29 in the mixed Au⋯π(aryl)/Au⋯O conformer. The degree of electron delocalization between Au and the thiocarbamate-O atom in the Au⋯O contact is relatively small in comparison (0.10), although larger than the DI with the atoms adjacent to the oxygen, which indicates an interaction weaker than Au⋯π(aryl), in agreement with the energies. In addition, the S–Au–P angle is linear for the Au⋯O contact but bends slightly (170°) when a Au⋯π(aryl) contact is present, indicating a steric clash between the P-bound ethyl groups and the central ring. Despite this steric clash, the large stabilization caused by electron delocalization across the intramolecular Au⋯π(aryl) contact is clearly responsible for the conformation adopted in the crystal structure.
Thermogravimetric analysis
Traces for the thermogravimetric analysis for 1–3 are given in ESI Fig. S4.† The decomposition pathways are quite similar for 1–3 and the following sequence is proposed. For 1, three discernible steps were resolved: step 1 – onset temperature 181.9 °C to end 226.9 °C resulted in a weight loss of 73.3% cf. 70.7% corresponding to the loss of 2Et3P. The second step (226.9–441.8 °C) saw a weight loss of 18.0% cf. 21.5%, correlated with loss of (2L–2S) leaving 2AuS (obs., calcd weight remaining 52.7% cf. 51.8%). The final step (441.8–806.1 °C) correlated with a weight loss of 7.2% cf. 7.3% expected for the loss of 2S with 2Au remaining (obs., calcd weight remaining 45.4% cf. 44.5%). The difference between the decomposition pathway for 1 and those for 2 and 3 is that there is no clear distinction between the first two steps for 2 and 3. Thus, for 2, step 1, between 163.5 and 346.7 °C, is accompanied by a weight loss of 55.2% cf. with 60.9% for the concomitant loss calculated for 2Ph3P and (2L–2S) (obs., calcd weight remaining for 2AuS 44.7% cf. 39.1%), and step 2, between 346.7 and 815.2 °C, having a weight loss of 9.8% cf. with the theoretical value of 5.5% expected for the loss of 2S leaving Au (obs., calcd weight remaining for 2Au 35.0% cf. 33.6%). The values for step 1 in the decomposition of 3 are onset-end temperatures 160.7 and 422.6 °C with 62.7% weight loss cf. with calculated 62.1% for the loss 2Cy3P and (2L–2S) (obs., calcd weight remaining for 2AuS 37.2% cf. 37.8%), and for step 2, 422.6 to 815.2 °C with 3.6% weight loss cf. with calculated 5.3% for the loss 2S (obs., calcd weight remaining for 2Au 33.6% cf. 32.6%).
Evaluation of anti-bacterial activity
A total of 24 strains of pathogen were screened in the present study, and the results of the disk diffusion assay are presented in the Table 4. Compounds 1–3 exhibited variable degrees of anti-bacterial activity against the clinically important pathogens tested; LH2 showed no inhibitory activity toward the tested bacteria. Of the series, 1 was the most active compound with complete inhibitory activity against all tested Gram-positive and Gram-negative pathogens, except P. aeruginosa, which is only partially inhibited by 1. A partial zone of inhibition is defined as incomplete inhibition of bacterial growth and the formation of a film of bacteria on the surface agar. The Gram-positive bacteria were most susceptible toward 1, with greater inhibition zones, ranging from 18 to 30 mm, compared with Gram-negative bacteria, with reduced inhibition zones, ranging from 7 to 23 mm. Mild anti-bacterial activities against several Gram-positive bacteria were observed for 2 and 3, with clear zones of inhibition ranging from 7 to 10 mm. Compound 2 was active against all the tested Gram-positive bacteria except B. cereus. By contrast, 3 exhibited the lowest activity of the series, being potent in only five of eight strains of Gram-positive bacteria, i.e. B. subtilis, E. faecium, L. monocytogenes, MRSA and S. saprophyticus. Clearly, in this preliminary anti-bacterial assay, the tested Gram-positive bacteria are more susceptible than Gram-negative bacteria. This finding is also supported by studies which reported that Gram-negative bacteria are less susceptible to anti-microbial agents34 and is consistent with results obtained recently for mononcuclear phosphanegold(I) analogues.11 The presence of the outer lipopolysaccharide layer in Gram-negative bacteria inhibits the access of most drugs to intracellular targets in bacteria and renders Gram-negative bacteria less susceptible to drugs than Gram-positive bacteria, which lack an outer membrane.35
Table 4 Anti-bacterial activity measured by zone of inhibition (mm) of 1–3, LH2 and standard anti-bioticsc
Microorganism |
1 |
2 |
3 |
LH2 |
Standard anti-biotics |
Tetracycline. Chloramphenicol. The diameter of inhibition zones in millimetres (mm) were measured in the disc after 24 h incubation;—, no zone of inhibition; (T), partial zone of inhibition. |
Gram-positive bacteria |
B. cereus ATCC10876 |
22 |
— |
— |
— |
11a |
B. subtilis ATCC6633 |
27 |
10 |
8 |
— |
24a |
E. faecalis ATCC29212 |
20 |
9 |
— |
— |
11a |
E. faecium ATCC19434 |
18 |
9 |
8 |
— |
16b |
L. monocytogenes ATCC19117 |
20 |
9 |
8 |
— |
27a |
S. aureus (MRSA) ATCC43300 |
23 |
9 |
7 |
— |
14b |
S. aureus ATCC25923 |
30 |
9 |
— |
— |
18b |
S. saprophyticus ATCC15305 |
30 |
9 |
8 |
— |
29a |
![[thin space (1/6-em)]](https://www.rsc.org/images/entities/char_2009.gif) |
Gram-negative bacteria |
A. baumannii ATCC19606 |
13 |
— |
— |
— |
16a |
A. hydrophilla ATCC35654 |
8 |
— |
— |
— |
22a |
C. freundii ATCC8090 |
8 |
— |
— |
— |
26a |
E. aerogenes ATCC13048 |
7 |
— |
— |
— |
20a |
E. cloacae ATCC35030 |
8 |
— |
— |
— |
20a |
E. coli ATCC25922 |
10 |
— |
— |
— |
18a |
K. pneumonia ATCC700603 |
8 |
— |
— |
— |
13a |
P. aeruginosa ATCC27853 |
14 (T) |
— |
— |
— |
14a |
P. mirabilis ATCC25933 |
8 |
— |
— |
— |
14b |
P. vulgaris ATCC13315 |
14 |
— |
— |
— |
19b |
S. typhimurium ATCC14028 |
9 |
— |
— |
— |
24a |
S. paratyphiA ATCC9150 |
9 |
— |
— |
— |
22a |
S. flexneri ATCC12022 |
12 |
— |
— |
— |
18a |
S. sonnei ATCC9290 |
10 |
— |
— |
— |
19a |
S. maltophilia ATCC13637 |
9 |
— |
— |
— |
23b |
V. parahaemolyticus ATCC17802 |
23 |
— |
— |
— |
30a |
Using the broth micro-dilution method, the anti-bacterial sensitivity of 1–3 was quantitatively assessed by determining the minimum inhibitory concentration (MIC), defined as the highest dilution at which no bacterial growth was detected, Table 5. A lower MIC value indicates a better anti-microbial agent as a smaller amount of trial compound is required to inhibit bacterial growth. Compound 1 exhibited excellent inhibitory activity toward all susceptible Gram-positive bacteria compared with 2 and 3, and standard anti-biotics (tetracycline and chloramphenicol), with lowest MIC values in the range 0.10–0.39 μg ml−1. The most susceptible Gram-positive bacteria strains toward 1 were B. cereus, L. monocytogenes, MRSA, S. aureus and S. saprophyticus (MIC's = 0.10 μg ml−1) follow by B. subtilis and E. faecium (MIC's = 0.20 μg ml−1) and E. faecalis (MIC = 0.39 μg ml−1). However, tetracycline and chloramphenicol showed equal or, normally, greater anti-bacterial activity toward Gram-negative bacteria compared to 1, with lower MIC values in the range 0.10–12.5 μg ml−1. Compound 2 exhibited more promising MIC values (0.78–6.25 μg ml−1) than 3 (6.25–50.00 μg ml−1) toward susceptible Gram-positive bacteria. In addition, 2 displayed greater activity compared with tetracycline and chloramphenicol against E. faecalis, E. faecium, L. monocytogenes, MRSA and S. aureus, with lower MIC values in the range 1.56–6.25 μg ml−1.
Table 5 MIC and MBC of 1–3, LH2 and standard anti-biotics against selected Gram-positive and Gram-negative bacteriac
Microorganism |
1 |
2 |
3 |
Standard anti-biotics |
MIC |
MBC/MIC |
MIC |
MBC/MIC |
MIC |
MBC/MIC |
MIC |
MBC/MIC |
Tetracycline. Chloramphenicol. MIC – minimum inhibitory concentration (μg ml−1); MBC/MIC ratio for bacteriostatic or bactericidal activity; – not applicable; ND – not determined as the bacterium had grown across all tested dilution (MBC > 100 μg ml−1). |
Gram-positive bacteria |
B. cereus ATCC10876 |
0.10 |
1 |
— |
— |
— |
— |
6.25a |
1 |
B. subtilis ATCC6633 |
0.20 |
1 |
0.78 |
1 |
25.00 |
1 |
0.20a |
1 |
E. faecalis ATCC29212 |
0.39 |
1 |
6.25 |
1 |
— |
— |
12.50a |
1 |
E. faecium ATCC19434 |
0.20 |
32 |
3.13 |
4 |
6.25 |
4 |
12.50b |
ND |
L. monocytogenes ATCC19117 |
0.10 |
1 |
1.56 |
1 |
6.25 |
1 |
12.50a |
1 |
S. aureus (MRSA) ATCC43300 |
0.10 |
2 |
1.56 |
1 |
50.00 |
1 |
12.50b |
1 |
S. aureus ATCC25923 |
0.10 |
2 |
3.13 |
1 |
— |
— |
12.50b |
1 |
S. saprophyticus ATCC15305 |
0.10 |
2 |
1.56 |
1 |
6.25 |
2 |
0.39a |
ND |
![[thin space (1/6-em)]](https://www.rsc.org/images/entities/char_2009.gif) |
Gram-negative bacteria |
A. baumannii ATCC19606 |
6.25 |
1 |
— |
— |
— |
— |
0.39a |
32 |
A. hydrophilla ATCC35654 |
6.25 |
2 |
— |
— |
— |
— |
0.39a |
1 |
C. freundii ATCC8090 |
25.00 |
4 |
— |
— |
— |
— |
0.78a |
32 |
E. aerogenes ATCC13048 |
25.00 |
4 |
— |
— |
— |
— |
1.56a |
32 |
E. cloacae ATCC35030 |
25.00 |
1 |
— |
— |
— |
— |
1.56a |
ND |
E. coli ATCC25922 |
12.50 |
1 |
— |
— |
— |
— |
0.39a |
ND |
K. pneumoniae ATCC700603 |
50.00 |
ND |
— |
— |
— |
— |
12.50a |
ND |
P. aeruginosa ATCC27853 |
— |
— |
— |
— |
— |
— |
6.25a |
ND |
P. mirabilis ATCC25933 |
12.50 |
2 |
— |
— |
— |
— |
12.50b |
8 |
P. vulgaris ATCC13315 |
3.13 |
4 |
— |
— |
— |
— |
12.50b |
4 |
S. typhimurium ATCC14028 |
12.50 |
1 |
— |
— |
— |
— |
1.56a |
32 |
S. paratyphi a ATCC9150 |
12.50 |
1 |
— |
— |
— |
— |
1.56a |
32 |
S. flexneri ATCC12022 |
6.25 |
1 |
— |
— |
— |
— |
0.78a |
32 |
S. sonnei ATCC9290 |
6.25 |
2 |
— |
— |
— |
— |
0.78a |
32 |
S. maltophilia ATCC13637 |
3.13 |
4 |
— |
— |
— |
— |
3.13b |
ND |
V. parahaemolyticus ATCC17802 |
1.56 |
1 |
— |
— |
— |
— |
0.10a |
1 |
The zone of inhibition and MIC tests may reflect temporary inhibition of bacterial growth (bacteriostatic) or bacterial killing (bactericidal) by a test compound. The bactericidal properties of 1–3 against susceptible strains were further analyzed by the minimum bactericidal concentration (MBC) assay and summarized as MBC/MIC ratios in Table 5. An anti-microbial agent is bactericidal if the MBC is not more than fourfold higher than the MIC, i.e. MBC/MIC ≤ 4. By contrast, the anti-microbial agent is bacteriostatic if the MBC is more than four-fold greater than the MIC, i.e. MBC/MIC ≤ 4.36 Compounds 2 and 3 were shown to be bactericidal (MBC/MIC ≤ 4) toward the susceptible Gram-positive bacteria strains. For 1, bactericidal activity was observed against all susceptible Gram-positive and Gram-negative bacteria with the exception of E. faecium (MBC/MIC = 32) and K. pneumoniae (MBC/MIC = ND). These results suggest that 1–3 are considered bactericidal agents but the activity of 1 is dependent on the specific bacterial strain.
The broad spectrum of activity exhibited by 1 (R = Et) against Gram-positive and Gram-negative bacteria, and the specific activity against Gram-positive bacteria exhibited by 2 and 3 suggests a key role for the phosphane ligand. This is vindication of an earlier study against the same panel of 24 pathogens but where the thiolate was a dithiocarbamate ligand, i.e. R3PAu[S2CN(iPr)CH2CH2OH], for R = Et, Ph and Cy.37 In this study a similar observation was made in that the R = Et derivative had broad range activity against both Gram-positive and Gram-negative bacteria, and the activity of the R = Ph and Cy species was limited to Gram-positive bacteria.37 Perhaps once thought as being too expensive for development as anti-microbial agents, the emerging resistance to conventional therapies suggest that gold compounds may play a clinical role, especially against virulent strains of bacteria such as MRSA.
Conclusions
Three new binuclear phospanegold(I) compounds of a bipodal ligand have been characterised. Crystallography shows the uniform adoption of a spherical aggregate featuring unexpected intramolecular Au⋯π(aryl) interactions. Theory shows these interactions to be attractive, providing energies of stabilization to the molecular structure of 1 greater than 12 kcal mol−1 compared to putative Au⋯O interactions. Compounds 1–3 exhibited, predominantly, a bactericidal effect on Gram-positive (1–3) and Gram-negative (only 1) pathogens, with 1 perhaps having potential clinical benefits over standard anti-biotics in bacteriostatic therapy for immune-compromised patients, i.e. with cancer and neutropenia, by prompt elimination of the pathogen to therefore reduce the likelihood of the spread of infection.
Acknowledgements
Support from the Ministry of Higher Education, Malaysia, and the University of Malaya, for High Impact Research grant (UM.C/HIR-MOHE/SC/12) is gratefully acknowledged. AOR acknowledges support from the Spanish Malta/Consolider initiative (no. CSD2007-00045) and the Alberta Innovates Technology Futures (AITF) for funding.
References
- e.g. S. S. Pathaneni and G. R. Desiraju, J. Chem. Soc., Dalton Trans., 1993, 319 RSC; M. J. Katz, K. Sakai and D. B. Leznoff, Chem. Soc. Rev., 2008, 37, 1884 RSC; H. Schmidbaur and A. Schier, Chem. Soc. Rev., 2008, 37, 1931 RSC; H. E. Abdou, A. A. Mohamed, J. P. Fackler, A. Burini, R. Galassi, J. M. Lopez-de-Luzuriaga and M. E. Olmos, Coord. Chem. Rev., 2009, 253, 1661 CrossRef CAS PubMed; H. Schmidbaur and A. Schier, Chem. Soc. Rev., 2012, 41, 370 RSC; E. R. T. Tiekink, Coord. Chem. Rev., 2014, 275, 130 CrossRef PubMed.
- P. Pyykkö and Y.-F. Zhao, Angew. Chem., Int. Ed. Engl., 1991, 30, 604 CrossRef CAS PubMed; P. Pyykkö, Inorg. Chim. Acta, 2005, 358, 4113 CrossRef PubMed; H. Schmidbaur and A. Schier, Chem. Soc. Rev., 2008, 37, 1931 RSC; S. Sculforta and P. Braunstein, Chem. Soc. Rev., 2011, 40, 2741 RSC.
- e.g. A. L. Balch, Struct. Bonding, 2007, 123, 1 CrossRef CAS; V. W.-W. Yam and E. C.-C. Cheng, Top. Curr. Chem., 2007, 281, 269 CrossRef; E. R. T. Tiekink and J.-G. Kang, Coord. Chem. Rev., 2009, 253, 1627 CrossRef PubMed; X. He and V. W.-W. Yam, Coord. Chem. Rev., 2011, 255, 2111 CrossRef PubMed; E. E. Langdon-Jones and S. J. A. Pope, Chem. Commun., 2014, 50, 10343 RSC.
-
(a) I. Caracelli, J. Zukerman-Schpector and E. R. T. Tiekink, Gold Bull., 2013, 46, 81 CrossRef CAS;
(b) J. Zukerman-Schpector and E. R. T. Tiekink, CrystEngComm, 2009, 11, 1176 RSC;
(c) E. R. T. Tiekink and J. Zukerman-Schpector, CrystEngComm, 2009, 11, 2701 RSC.
- e.g.
(a) B. K. Min and C. M. Friend, Chem. Rev., 2007, 107, 2709 CrossRef CAS PubMed;
(b) T. Takei, T. Akita, I. Nakamura, T. Fujitani, M. Okumura, K. Okazaki, J. H. Huang, T. Ishida and M. Haruta, Adv. Catal., 2012, 55, 1 CAS;
(c) M. Pan, J. L. Gong, G. B. Dong and C. B. Mullins, Acc. Chem. Res., 2014, 47, 750 CrossRef CAS PubMed.
-
(a) S. Y. Ho, E. C.-C. Cheng, E. R. T. Tiekink and V. W.-W. Yam, Inorg. Chem., 2006, 45, 8165 CrossRef CAS PubMed;
(b) S. Y. Ho and E. R. T. Tiekink, CrystEngComm, 2007, 9, 368 RSC;
(c) F. S. Kuan, S. Y. Ho, P. P. Tadbuppa and E. R. T. Tiekink, CrystEngComm, 2008, 10, 548 RSC.
-
(a) S. J. Berners-Price and A. Filipovska, Metallomics, 2011, 3, 863 RSC;
(b) W. F. Kean and I. R. L. Kean, Inflammopharmacology, 2008, 16, 112 CrossRef CAS PubMed;
(c) E. R. T. Tiekink, Gold Bull., 2003, 36, 117 CrossRef CAS;
(d) J. F. Fries, D. Bloch, P. Spitz and D. M. Mitchell, Am. J. Med., 1985, 78, 56 CrossRef CAS.
- E. R. T. Tiekink, Critical Reviews in Oncology/Hematology, 2002, 42, 225 CrossRef CAS; I. Ott, Coord. Chem. Rev., 2009, 253, 1670 CrossRef PubMed; C. Gabbiani, M. A. Cinellu, L. Maiore, L. Massai, F. Scaletti and L. Messori, Inorg. Chim. Acta, 2012, 393, 115 CrossRef PubMed; N. Cutillas, G. S. Yellol, C. de Haro, C. Vicente, V. Rodriguez and J. Ruiz, Coord. Chem. Rev., 2013, 257, 2784 CrossRef PubMed; C. Nardon, S. M. Schmitt, H. J. Yang, J. Zuo, D. Fregona and Q. P. Dou, PLoS One, 2014, 9, e84248 Search PubMed; B. Bertrand and A. Casini, Dalton Trans., 2014, 4209 RSC.
- C. I. Yeo, K. K. Ooi, A. Md Akim, K. P. Ang, Z. A. Fairuz, S. N. B. A. Halim, S. W. Ng, H.-L. Seng and E. R. T. Tiekink, J. Inorg. Biochem., 2013, 127, 24 CrossRef CAS PubMed.
- B. Đ. Glišić and M. I. Djuran, Dalton Trans., 2014, 5950 RSC.
- C. I. Yeo, J.-H. Sim, C.-H. Khoo, Z.-J. Goh, K.-P. Ang, Y.-K. Cheah, Z. A. Fairuz, S. N. B. A. Halim, S. W. Ng, H.-L. Seng and E. R. T. Tiekink, Gold Bull., 2013, 46, 145 CrossRef CAS.
- R. K. Harris, Nuclear Magnetic Resonance Spectroscopy, Longman, Harlow, 1986 Search PubMed.
- X'Pert HighScore Plus, ed. B. V. Almelo, PANalytical, The Netherlands, 2009 Search PubMed.
-
(a) V. J. Hall, G. Siasios and E. R. T. Tiekink, Aust. J. Chem., 1993, 46, 561 CrossRef CAS;
(b) S. Y. Ho, R. P. A. Bettens, D. Dakternieks, A. Duthie and E. R. T. Tiekink, CrystEngComm, 2005, 7, 682 RSC;
(c) F. S. Kuan, F. Mohr, P. P. Tadbuppa and E. R. T. Tiekink, CrystEngComm, 2007, 9, 574 RSC.
-
(a) CrysAlisPro, Agilent Technologies, Santa Clara, CA, USA, 2014 Search PubMed;
(b) G. M. Sheldrick, Acta Crystallogr., Sect. A: Found. Crystallogr., 2008, 64, 112 CrossRef CAS PubMed;
(c) L. J. Farrugia, J. Appl. Crystallogr., 2012, 45, 849 CrossRef CAS;
(d) A. L. Spek, Acta Crystallogr., Sect. D: Biol. Crystallogr., 2009, 65, 148 CrossRef CAS PubMed;
(e) DIAMOND, Visual Crystal Structure Information System, Version 3.1, CRYSTAL IMPACT, Postfach 1251, D-53002 Bonn, Germany, 2006 Search PubMed;
(f) J. Gans and D. Shalloway, J. Mol. Graphics Modell., 2001, 19, 557 CrossRef CAS.
- M. J. Frisch, G. W. Trucks, H. B. Schlegel, G. E. Scuseria, M. A. Robb, J. R. Cheeseman, G. Scalmani, V. Barone, B. Mennucci, G. A. Petersson, H. Nakatsuji, M. Caricato, X. Li, H. P. Hratchian, A. F. Izmaylov, J. Bloino, G. Zheng, J. L. Sonnenberg, M. Hada, M. Ehara, K. Toyota, R. Fukuda, J. Hasegawa, M. Ishida, T. Nakajima, Y. Honda, O. Kitao, H. Nakai, T. Vreven, J. A. Montgomery Jr, J. E. Peralta, F. Ogliaro, M. Bearpark, J. J. Heyd, E. Brothers, K. N. Kudin, V. N. Staroverov, R. Kobayashi, J. Normand, K. Raghavachari, A. Rendell, J. C. Burant, S. S. Iyengar, J. Tomasi, M. Cossi, N. Rega, J. M. Millam, M. Klene, J. E. Knox, J. B. Cross, V. Bakken, C. Adamo, J. Jaramillo, R. Gomperts, R. E. Stratmann, O. Yazyev, A. J. Austin, R. Cammi, C. Pomelli, J. W. Ochterski, R. L. Martin, K. Morokuma, V. G. Zakrzewski, G. A. Voth, P. Salvador, J. J. Dannenberg, S. Dapprich, A. D. Daniels, Ö. Farkas, J. B. Foresman, J. V. Ortiz, J. Cioslowski and D. J. Fox, Gaussian 09, Revision A.1, Gaussian, Inc., Wallingford CT, 2009 Search PubMed.
- O. A. Vydrov and G. E. Scuseria, J. Chem. Phys., 2006, 125, 234109 CrossRef PubMed; O. A. Vydrov, J. Heyd, A. V. Krukau and G. E. Scuseria, J. Chem. Phys., 2006, 125, 074106 CrossRef PubMed.
- A. D. Becke and E. R. Johnson, J. Chem. Phys., 2007, 127, 154108 CrossRef PubMed.
- E. R. Johnson and A. D. Becke, J. Chem. Phys., 2006, 124, 174104 CrossRef PubMed.
- A. Otero-de-la-Roza and E. R. Johnson, J. Chem. Phys., 2013, 138, 204109 CrossRef CAS PubMed.
- http://gatsby.ucmerced.edu/.
- A. Otero-de-la-Roza, J. D. Mallory and E. R. Johnson, J. Chem. Phys., 2014, 140, 18A504 CrossRef PubMed.
- K. A. Peterson and C. Puzzarini, Theor. Chem. Acc., 2005, 114, 283 CrossRef CAS.
- E. R. Johnson, A. Otero-de-la-Roza, S. G. Dale and G. A. DiLabio, J. Chem. Phys., 2013, 139, 214109 CrossRef PubMed.
- S. Ng, F. M. Salleh, Y. Xie and H. F. Schaefer III, J. Phys. Org. Chem., 2012, 25, 1374 CrossRef CAS PubMed.
- S. Y. Ho, C. S. Lai and E. R. T. Tiekink, Acta Crystallogr., Sect. E: Struct. Rep. Online, 2003, 59, o1155 CAS.
- C. R. Groom and F. H. Allen, Angew. Chem., Int. Ed., 2014, 53, 662 CrossRef CAS PubMed.
- P. P. Tadbuppa and E. R. T. Tiekink, Acta Crystallogr., Sect. E: Struct. Rep. Online, 2007, 63, m1101 CAS; P. P. Tadbuppa and E. R. T. Tiekink, Acta Crystallogr., Sect. E: Struct. Rep. Online, 2009, 65, m1557 Search PubMed; P. P. Tadbuppa and E. R. T. Tiekink, Acta Crystallogr., Sect. E: Struct. Rep. Online, 2009, 65, m1558 Search PubMed; P. P. Tadbuppa and E. R. T. Tiekink, Acta Crystallogr., Sect. E: Struct. Rep. Online, 2009, 65, m1646 Search PubMed; P. P. Tadbuppa and E. R. T. Tiekink, Acta Crystallogr., Sect. E: Struct. Rep. Online, 2009, 65, m1700 Search PubMed; P. P. Tadbuppa and E. R. T. Tiekink, Acta Crystallogr., Sect. E: Struct. Rep. Online, 2010, 66, m450 Search PubMed; P. P. Tadbuppa and E. R. T. Tiekink, Acta Crystallogr., Sect. E: Struct. Rep. Online, 2010, 66, m664 Search PubMed.
-
(a) H. Schmidbaur, A. Wohlleben, F. Wagner, O. Orama and G. Huttner, Chem. Ber., 1977, 110, 1748 CrossRef CAS PubMed;
(b) P. C. Healy, Acta Crystallogr., Sect. E: Struct. Rep. Online, 2003, 59, m1112 CAS.
-
(a) G. Siasios and E. R. T. Tiekink, Z. Kristallogr., 1993, 204, 95 CrossRef CAS PubMed;
(b) G. Siasios and E. R. T. Tiekink, Z. Kristallogr., 1993, 205, 261 CrossRef CAS PubMed;
(c) E. R. T. Tiekink and I. Haiduc, Prog. Inorg. Chem., 2005, 54, 127 CrossRef CAS PubMed;
(d) P. D. Cookson and E. R. T. Tiekink, J. Coord. Chem., 1992, 26, 313 CrossRef CAS PubMed;
(e) D. R. Smyth, B. R. Vincent and E. R. T. Tiekink, Cryst. Growth Des., 2001, 1, 113 CrossRef CAS.
- J. D. Dunitz and A. Gavezzotti, Acc. Chem. Res., 1999, 32, 677 CrossRef CAS; J. D. Dunitz and A. Gavezzotti, Chem. Soc. Rev., 2009, 38, 2622 RSC; J. D. Dunitz and A. Gavezzotti, Cryst. Growth Des., 2012, 12, 5873 Search PubMed.
- R. F. W. Bader and M. E. Stephens, J. Am. Chem. Soc., 1975, 97, 7391 CrossRef CAS; R. F. W. Bader, A. Streitwieser, A. Neuhaus, K. E. Laidig and P. Speers, J. Am. Chem. Soc., 1996, 118, 4959 CrossRef.
- A. Otero de la Roza, E. R. Johnson and G. A. DiLabio, J. Chem. Theory Comput., 2014, 10, 5436 CrossRef CAS.
- K. S. O. Ferraz, N. F. Saliva, J. G. D. Silva, L. F. D. Miranda, C. F. D. Romeiro, E. M. Souza-Fagundes, I. C. Mendes and H. Beraldo, Eur. J. Med. Chem., 2012, 53, 98 CrossRef CAS PubMed.
- P. Burn, Trends Biochem. Sci., 1998, 13, 79 CrossRef.
- M. E. Levison, Infect. Dis. Clin., 2004, 18, 451 CrossRef PubMed.
- J.-H. Sim, N. S. Jamaludin, C.-H. Khoo, Y.-K. Cheah, S. N. B. A. Halim, H.-L. Seng and E. R. T. Tiekink, Gold Bull., 2014, 47, 225 CrossRef CAS.
Footnote |
† Electronic supplementary information (ESI) available. CCDC 1041500–1041502. For ESI and crystallographic data in CIF or other electronic format see DOI: 10.1039/c5ra05604g |
|
This journal is © The Royal Society of Chemistry 2015 |