DOI:
10.1039/C5RA05307B
(Paper)
RSC Adv., 2015,
5, 64046-64054
Determination of DNA adenine methylation in genomes of mammals and plants by liquid chromatography/mass spectrometry†
Received
25th March 2015
, Accepted 17th July 2015
First published on 21st July 2015
Abstract
DNA adenine methylation (N6-methyl-2′-deoxyadenosine, m6dA) plays important functional roles in prokaryotes and protists, including regulation of gene transcription, DNA replication and repair, and the restriction-modification system. However, there is no definitive evidence supporting the presence of DNA adenine methylation in genomic DNA of higher eukaryotes, such as mammals and plants, where DNA cytosine methylation (5-methylcytosine) instead is well recognized as an important epigenetic mark that has regulatory roles in various biological processes. In the current study, we developed a Dpn I cleavage coupled with size-exclusion ultrafiltration method, with which we discovered the wide-spread existence of m6dA in genomic DNA of higher eukaryotes, including human cells, rat tissues, and plants besides bacteria and protists by employing high-resolution mass spectrometry analysis. And the contents of m6dA vary in different cell types with the range of 0.00006–0.00077% (m6dA dA−1). Moreover, similar to N6-methyladenosine (m6A) in RNA, m6dA contents significantly decreased in type 2 diabetes mellitus (T2DM) patients compared to control subjects, indicating m6dA plays important roles in the pathogenesis of T2DM as m6A. In addition, knockdown of cellular fat mass and obesity-associated (FTO) protein increased the m6dA content, while overexpression of cellular FTO decreased m6dA content in DNA, suggesting m6dA and m6A may share the same demethylase of FTO. The demonstration of the universal presence of DNA adenine methylation constitutes the first and essential step toward understanding of m6dA functions in higher eukaryotes.
Introduction
Accumulating evidence suggests that DNA adenine methylation (N6-methyl-2′-deoxyadenosine, m6dA) plays various functional roles in prokaryotes.1 DNA adenine methylation is related to the host-specific restriction-modification (R-M) system. The R-M system is taken as a defense mechanism against bacteriophage infection in bacteria, in which methylation of bacterial DNA protects it from the action of the corresponding restriction endonuclease digestion; whereas unmethylated sites of foreign DNA such as bacteriophage DNA are cleaved.2,3 In addition, DNA adenine methylation can regulate gene transcription through modulating the binding of regulatory proteins to DNA.4,5 Moreover, DNA adenine methylation was found to be required for the control of initiation of DNA replication6,7 and was involved in DNA mismatch repair.8
In addition to the wide existence in prokaryotes, DNA adenine methylation has also been found to be present in DNA of several protist, including genera Chlamydomonas, Chlorella, Oxytricha, Paramecium and Tetrahymena.9–11 One previous study also reported the existence of m6dA in DNA of human, Drosophila and mealybug by immunochemical method;12 however, the result is not conclusive since the possible contamination of RNA and the less specificity of used antibodies. To the best of our knowledge, there is no other definitive evidence supporting for the presence of DNA adenine methylation in genomic DNA of higher eukaryotes, such as mammals and plants. During the revision of this manuscript, three papers have been published for the discovery of m6dA in eukaryotes, indicating that m6dA may serve as a novel potential epigenetic mark.13–15 Instead, DNA cytosine methylation (5-methylcytosine, 5-mC) in genomic DNA of mammals and plants is well recognized as an important epigenetic mark that has regulatory roles in various biological processes.16–19 Properly established and maintained DNA cytosine methylation patterns are crucial for the normal functions of living cells.20
The assumption that DNA adenine methylation does not occur in mammals and plants is based on the use of low-sensitivity methods with a detection limit of 0.01–0.1% (m6dA dA−1), including radioactive labeling method, HPLC-UV method, and micellar electrokinetic chromatography-laser-induced fluorescence method.21–23 The sensitivity may not be enough to discover m6dA in DNA if it occurs in a rare population in higher eukaryotes. Nevertheless, it should be pointed out that an overall content of modified nucleosides less than 0.0001% may be biologically significant if it occurs in specific gene regulatory elements, such as recently discovered 5-hydroxymethtylcytosine (5-hmC).24,25 Hence, according to the size of the human genome (∼3 × 109 bp), the presence of a few hundred m6dA may be sufficient to play a crucial role in the regulation of biological processes, which points to the importance of highly specific and sensitive methods to investigate the presence of m6dA in DNA of higher eukaryotes. In addition, the essential roles of DNA adenine methyltransferases in bacterial viability and virulence underscore the importance for the investigation of the presence and biological functions of DNA adenine methylation in higher eukaryotes. Therefore, the knowledge of the DNA adenine methylation status in eukaryotic DNA is necessary for development of antibiotics targeting bacterial DNA adenine methyltransferases.
Unlike DNA adenine methylation, RNA adenine methylation (N6-methyladenosine, m6A) has long been identified to exist in both prokaryotes and eukaryotes with high abundance.26–28 The cell-type and cell-state-dependent m6A distribution indicates that m6A modifications are highly dynamic.29,30 Recent studies have also demonstrated that m6A plays critical roles in DNA transcription, RNA stability, translational regulation and microRNA maturation, and thus involves in a variety of biological processes including adipogenesis, spermatogenesis, development, carcinogenesis, circadian rhythm and stem cell renewal.31–34 Considering 5-methylcytosine that is widely viewed as the fifth base in genomic DNA exists in both DNA (5-methyl-2′-deoxycytidine) and RNA (5-methylcytidine), we hypothesize that m6dA may also occur in DNA as m6A in RNA in higher eukaryotes (Fig. 1). With respect to the essential roles of m6dA in DNA in prokaryotes and protist and the wide existence of m6A in RNA of higher eukaryotes, here we explored m6dA modification in DNA of higher eukaryotes by employing high-resolution mass spectrometry analysis. Our results demonstrated that m6dA is widely present in higher eukaryotes, including mammals and plants. And m6dA contents vary largely in different cell lines and tissues. In our previous study, we demonstrated the m6A contents in peripheral blood RNA from type 2 diabetes mellitus (T2DM) patients were significantly lower compared to the healthy controls.35 Here we further discovered that m6dA from peripheral blood DNA also significantly decreased in T2DM patients compared to the healthy controls. In addition, knockdown and overexpression of cellular fat mass and obesity-associated protein (FTO) changed m6dA contents in DNA and m6A contents in RNA, suggesting both m6dA and m6A may share the same demethylase of FTO.
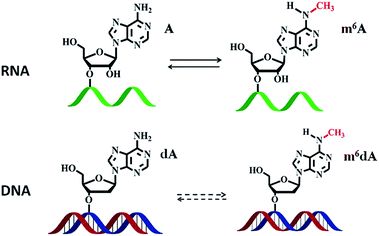 |
| Fig. 1 RNA adenine methylation and DNA adenine methylation. | |
Experimental
Chemicals and reagents
2′-Deoxycytidine (dC), 2′-deoxyguanosine (dG), 2′-deoxyadenosine (dA), thymidine (T), cytidine (rC), guanosine (rG), adenosine (rA), uridine (rU) were purchased from Sigma-Aldrich (Beijing, China). N6-Methyl-deoxyadenosine (m6dA) was obtained from Berry & associates Inc. (Michigan, USA). N6-Methyladenosine (m6A) was from Hanhong Chemical Co., Ltd (Shanghai, China). S1 nuclease and calf intestinal alkaline phosphatase (CIAP) were from Takara Biotechnology (Dalian, China). Phosphodiesterase I was purchased from Sigma-Aldrich (St. Louis, MO, USA). Chromatographic grade methanol was purchased from Merck (Darmstadt, Germany). Agarose, tris hydroxymethyl aminomethane (Tris), ethylenediaminetetraacetic acid (EDTA), boric acid, chloroform and formic acid (FA) were purchased from Sinopharm Chemical Reagent Co., Ltd (Shanghai, China). The water used throughout the study was purified by a Milli-Q apparatus (Millipore, Bedford, MA). Stock solutions of these nucleosides were prepared in Milli-Q water at a concentration of 4 mmol L−1.
Biological samples and DNA extraction
Human leukemia Jurkat T cells (Jurkat-T), human embryonic kidney cells (293T), GW5100 E. coli cells were obtained from the Cell Bank of Type Culture Collection, Chinese Academy of Sciences, and cultured according to previously described method.36,37 Ciprofloxacin (10 μg ml−1) was added in the culture medium to inhibit the possible contamination of Mycoplasma. Genomic DNA was extracted using DNAiso Reagent (Takara Biotechnology, Dalian, China) according to the manufacture recommended procedure.
Male Sprague-Dawley rat (4 weeks old) was obtained from the Center for Animal Experiment/ABSL-3 Laboratory of Wuhan University and sacrificed to collect tissues and stored under −80 °C. Genomic DNA was extracted using E.Z.N.A.® Tissue DNA kit (Omega Bio-Tek Inc., Norcross, GA, USA) according to the manufacture recommended procedure.
Plant samples, including Oryza sativa and Zea mays were used in this study. The culturing conditions were according to our previously reported method.38 Plant genomic DNA was isolated using E.Z.N.A.® plant DNA kit (Omega Bio-Tek Inc., Norcross, GA, USA) according to the manufacture recommended procedure.
Fresh peripheral blood samples of 15 T2DM patients and 15 participants randomly selected from healthy individuals who had no history of endocrine disease or chronic disease were stored at 4 °C in the presence of EDTA as an anticoagulant. An aliquot of 500 μL of blood was subjected to extraction of DNA using the E.Z.N.A.® blood DNA kit (Omega Bio-Tek Inc., Norcross, GA, USA) following the manufacture recommended procedure. Written informed consent was obtained from all subjects. This study was approved by the ethics committee of Zhongnan Hospital of Wuhan University and conducted in accordance with the Helsinki Declaration.
Knockdown and overexpression of FTO by transfection
FTO siRNA were synthesized by Takara Biotechnology (Dalian, China). The target FTO mRNA sequence is 5′-AAAUAGCCGCUGCUUGUGAGA-3′ according to previous report.39 A non-sense siRNA with the sequence 5′-UUCUCCGAACGUGUCACGUTT-3′ was used as the control. For the overexpression of FTO, we constructed a vector pcDNA3.1-FTO, and the empty vector pcDNA3.1 was served as the control. Lipofectamine RNAiMAX (Invitrogen) and lipofectamine 2000 (Invitrogen) were used to perform siRNA and plasmid transfection, respectively, according to the manufacturer's instructions. The mRNA expression level of FTO after knockdown and overexpression was determined by quantitative real-time PCR (CFX96 Touch™, BIORAD) according to our previously described method.35
Enzymatic digestion of DNA
The extracted genomic DNA was first digested by Dpn I restriction enzyme followed by ultrafiltration to remove the possible contamination of bacterial DNA (Fig. 2). Typically, a 50 μL mixture including 6 μg genomic DNA, 5 μL 10 × cutsmart buffer (New England Biolabs, Ipswich, WA), 80 units of Dpn I restriction enzyme (4 μL, New England Biolabs, Ipswich, WA) and 41 μL H2O was incubated at 37 °C for 2 h. Then the reaction solution was transferred to a 100 kDa cut-off centrifugal filter (Millipore, Bedford, MA) and centrifuged at 8000 × g for 3 min. The centrifugal filter was washed 10 times with 200 μL of water for each time. The DNA retained on the filter was then desorbed by water.
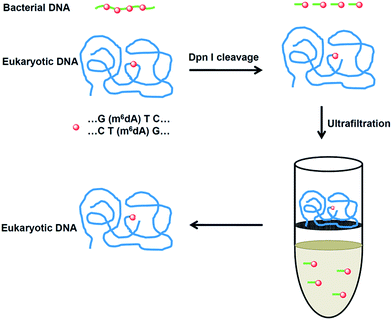 |
| Fig. 2 Schematic diagram for the elimination of possible contamination of bacterial DNA by Dpn I cleavage coupled with size-exclusion ultrafiltration. | |
The resulting genomic DNA was enzymatically digested according to previously described method.40 Briefly, genomic DNA (5 μg in 16 μL H2O) was first denatured by heating at 95 °C for 5 min and then chilling on ice for 2 min. After adding 2 μL of S1 nuclease buffer (30 mM CH3COONa, pH 4.6, 280 mM NaCl, 1 mM ZnSO4) and 360 units of S1 nuclease (2 μL), the mixture (20 μL) was then incubated at 37 °C for 16 h. To the solution was subsequently added 10 μL of alkaline phosphatase buffer (50 mM Tris-HCl, 10 mM MgCl2, pH 9.0), 0.005 units of venom phosphodiesterase I (5 μL), 30 units of alkaline phosphatase (1 μL) and 64 μL H2O. And then the incubation was continued at 37 °C for an additional 4 h followed by extraction with equal volume of chloroform twice. The resulting aqueous layer was collected and lyophilized to dryness and then reconstituted in 100 μL water followed by analysis with liquid chromatography-electrospray ionization-tandem mass spectrometry (LC-ESI-MS/MS) analysis.
LC-ESI-MS/MS analysis
Analysis of the mammals and plants samples was performed on LC-ESI-MS/MS system consisting of an AB 3200 QTRAP mass spectrometer (Applied Biosystems, Foster City, CA, USA) with an electrospray ionization source (Turbo Ionspray) and a Shimadzu LC-20AD HPLC (Tokyo, Japan) with two LC-20AD pumps, a SIL-20A autosampler, a CTO-20AC thermostatted column compartment, and a DGU-20A3 degasser. Data acquisition and processing were performed using AB SCIEX Analyst 1.5 Software (Applied Biosystems, Foster City, CA). The HPLC separation was performed on a Hisep C18-T column (150 mm × 2.1 mm i.d., 5 μm, Weltech Co., Ltd, Wuhan, China) with a flow rate of 0.2 mL min−1 at 35 °C. FA in water (0.1%, v/v, solvent A) and FA in methanol (0.1% v/v, solvent B) were employed as mobile phase. A gradient of 5 min 5% B, 10 min 5–30% B, 5 min 30–50% B, 3 min 50–5% B and 17 min 5% B was used.
The mass spectrometry detection was performed under positive electrospray ionization mode. The target nucleosides were monitored by multiple reaction monitoring (MRM) mode using the mass transitions (precursor ions → product ions) of dC (228.4 → 112.2), T (243.3 → 127.2), dA (252.4 → 136.2), dG (268.4 → 152.4), m6dA (266.1 → 150.2), rC (244.4 → 112.2), rU (245.4 → 113.1), rA (268.4 → 136.2), rG (284.5 → 152.2), m6A (282.2 → 150.1). The MRM parameters of all nucleosides were optimized to achieve maximal detection sensitivity (Table S1, ESI†).
Investigation of the retention behavior of m6dA
To confirm m6dA in genomic DNA of higher eukaryotes, the retention behavior of suspected m6dA from genomic DNA of 293T cells was compared with m6dA standard using different columns. Besides the reversed-phase Hisep C18-T column, Hisep OTS column (mix-mode C8-SO3, 150 mm × 2.1 mm i.d., 5 μm, Weltech Co., Ltd, Wuhan, China) and HILIC pNA-sil column (150 mm × 2.1 mm i.d., 5 μm)41 were also used. As for the Hisep OTS column, solvent A (2.5 mM HCOONH4 and 0.01% FA in H2O) and solvent B (2.5 mM HCOONH4 and 0.01% FA in CH3OH) were used as mobile phase. A gradient of 9 min 3–13% B, 1 min 13–40% B, 20 min 40–80% B, 2 min 80% B, 2 min 80–3% B, 10 min 3% B was used. As for the HILIC pNA-sil column, H2O/ACN (15/85, v/v) containing 0.01% FA was used as mobile phase for isocratic elution. A flow rate of 0.2 mL min−1 at 35 °C was used for both columns.
Determination of m6dA by high-resolution mass spectrometry
High-resolution mass spectrometry analysis was performed using LTQ XL Orbitrap mass spectrometer (Thermo Fisher Scientific). The HPLC separation was performed on a Zorbax SB-C18 column (2.1 mm × 150 mm, 5 μm, Agilent). Methanol/water was used as mobile phase with the flow rate of 0.2 mL min−1, which was delivered using an Agilent 1100 HPLC pump. A gradient of 2 min 5% methanol, 8 min 5–100% methanol, 5 min 100–5% methanol and 7 min 5% methanol was employed for the separation. A full MS scan and a MS/MS scan were used to elucidate the structural information of m6dA.
Calculation of the percentage of DNA and RNA adenine methylation
m6dA content in DNA and m6A content in RNA were calculated using the following expression:
where Mm6dA (or m6A) is the molar quantity of m6dA or m6A, and MdA (or rA) is the molar quantity of dA or rA determined in DNA or RNA sample.
Statistical analysis
Statistical analyses were performed using SPSS 19.0 software (SPSS Inc., Chicago, USA). Student's unpaired t-test was used to assess the differences of DNA and RNA adenine methylation. All p values were two-sided, and p < 0.05 were considered to be statistically significant.
Results and discussion
The fundamental role of m6dA in bacteria raises fascinating questions on its phyletic distribution and possible existence in higher mammals and plants. Previous reports suggested no detectable m6dA was observed in DNA of mammals or plants, which, however, could be due to the less sensitive detection methods used as well as the low abundance of m6dA in DNA of higher eukaryotes.1 It is worth noting that an overall content even less than 0.0001% of modified nucleosides in DNA can be biologically significant. Therefore, it is necessary to reinvestigate the existence of m6dA in genomic DNA of higher eukaryotes with highly sensitive detection method.
Discovery of m6dA in genomic DNA of mammalian cells by LC-ESI-MS/MS
Here we developed a sensitive LC-ESI-MS/MS method to explore m6dA modification in DNA of mammals and plants. In this respect, we monitored m6dA from the sample of digested genomic DNA of 293T cells by LC-ESI-MS/MS under MRM mode. The result showed a suspected peak that had a similar retention time as m6dA standard was observed (Fig. 3), suggesting the possible existence of m6dA in genomic DNA of 293T cells.
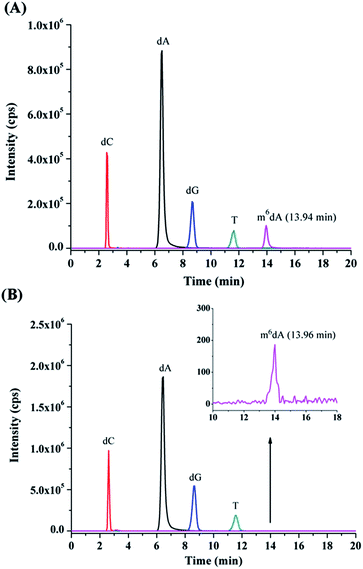 |
| Fig. 3 The MRM chromatograms of nucleosides. (A) Standard nucleosides. (B) Genomic DNA from 293T cells after enzymatic digestion. Shown in inset is the enlargement chromatogram of m6dA. Separation column, reversed-phase Hisep C18-T column. | |
Because m6dA modification that exists in genomic DNA of bacteria may contaminate cultured eukaryotic cells and tissues, elimination of bacterial contamination is therefore essential to avoid the false positive result. To this end, taken the advantage of Dpn I selective cleavage of G(m6dA)TC sites in DNA,42,43 bacterial DNA can be cut into short fragments since adenines in bacteria DNA are heavily modified to m6dA. And only the methylated adenine (m6dA) in the recognition sites will be cleaved by Dpn I, while normal adenine in GATC sites cannot be degraded. On the other side, due to the extremely low abundance of m6dA in genomic DNA of mammals and plants, the DNA of mammals and plants remains in large-size after Dpn I digestion. Therefore, the small DNA fragments of bacteria can be subsequently removed from large-size genomic DNA of mammals and plants using size-exclusion ultrafiltration (Fig. 2). In this respect, the agarose gel electrophoresis results showed that after Dpn I digestion, most of the fragments of bacterial DNA are smaller than 2500 bp (Fig. 4, lane 3), suggesting efficient cleavage of bacterial DNA. While no obvious change of genomic DNA of 293T cells was observed after Dpn I digestion (Fig. 4, lane 4 and lane 5), which is due to the relatively low abundance of m6dA existing in large-size genomic DNA of mammals and plants. These results indicated that Dpn I could efficiently cleave bacterial DNA but not eukaryotic DNA.
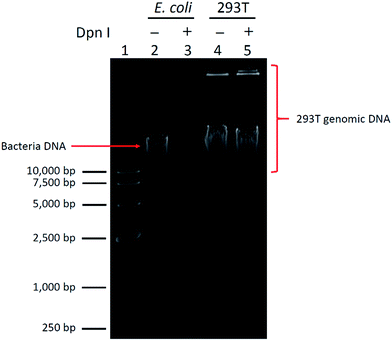 |
| Fig. 4 Agarose gel electrophoresis analysis of DNA samples digested by Dpn I restriction enzyme. After Dpn I restriction enzyme cleavage, the DNA samples were loaded into a 0.8% agarose gel for electrophoresis with 0.5 × TBE at 120 V for 1 h at room temperature. The gel was then staining by GelRed (Biotium, Inc., USA) and analysed using the Pharos FX Molecular Imager (Bio-Rad, USA). Lane 1, DNA marker; lane 2, bacterial DNA; lane 3, bacterial DNA digested by Dpn I; lane 4, 293T genomic DNA; lane 5, 293T genomic DNA digested by Dpn I. | |
Then after Dpn I digestion, the small bacterial DNA fragments could be efficiently removed by size exclusion ultrafiltration, while the large-size eukaryotic DNA retained on the filter. As shown in Fig. S1A and S1B,† m6dA is undetectable after Dpn I digestion and size-exclusion ultrafiltration even using 100 ng bacterial DNA. In addition, the signal of m6dA in 5 μg genomic DNA of 293T cells (Fig. S1C, ESI†) is much lower than that of 100 ng bacterial DNA (Fig. S1A, ESI†). Therefore, the bacterial DNA contamination in 293T cells should be less than 100 ng if 293T cells were contaminated by bacteria. Because 100 ng bacterial DNA can be efficiently removed (comparing Fig. S1A and S1B, ESI†), the Dpn I cleavage coupled with size-exclusion ultrafiltration method demonstrated to be capable to effectively eliminate bacterial DNA contamination in the determination of m6dA in eukaryotic cells. And therefore the signal observed in 293T cells after Dpn I cleavage coupled with size-exclusion ultrafiltration treatment should be from the genomic DNA of 293T cells (Fig. S1D, ESI†). Therefore, all the following DNA samples were treated with Dpn I cleavage coupled with size-exclusion ultrafiltration to eliminate possible bacterial DNA contamination.
To further confirm the identity of the observed peak, we compared the retention time of m6dA standard and the suspected m6dA from genomic DNA of 293T cells using different columns in the LC-ESI-MS/MS analysis. In this respect, a Hisep OTS column and a HILIC pNA-sil column were used besides the reversed-phase C18 column. The retention time on Hisep OTS column was 23.47 min for m6dA standard (Fig. 5A) and 23.46 min for the suspected m6dA peak from genomic DNA of 293T cells (Fig. 5B). Likewise, the retention time on HILIC pNA-sil column was 3.29 min for m6dA standard (Fig. 5C) and 3.32 min for the suspected m6dA peak from genomic DNA of 293T cells (Fig. 5D). The high consensus on retention times of m6dA standard and the suspected m6dA peak from genomic DNA of 293T cells under different HPLC separation mode revealed that these two peaks could represent the same compound.
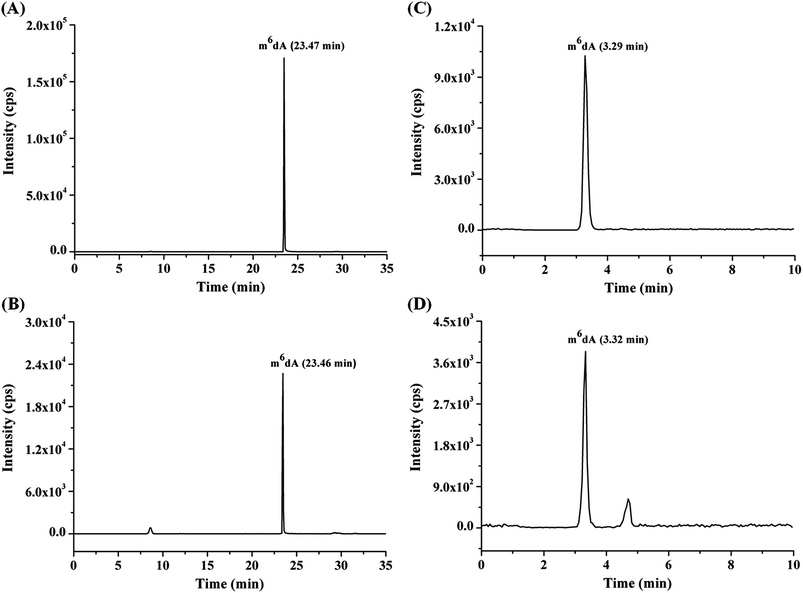 |
| Fig. 5 Retention of the m6dA on different separation columns. (A) MRM chromatogram of m6dA standard on mix-mode C8-SO3 column. (B) MRM chromatogram of putative m6dA in genomic DNA of 293T cells on mix-mode C8-SO3 column. (C) MRM chromatogram of m6dA standard on HILIC pNA-sil column. (D) MRM chromatogram of putative m6dA in genomic DNA of 293T cells on HILIC pNA-sil column. | |
Validation of m6dA in genomic DNA of higher eukaryotes by high-resolution mass spectrometry analysis
We further compared the fragmentation pattern of the m6dA standard and the putative m6dA in genomic DNA of 293T cells using high-resolution LTQ XL Orbitrap mass spectrometer. The results showed that two ions of m/z 266.1243 and m/z 150.2387, which represent the parent ion of m6dA and its product ion, were observed for m6dA standard under full scan mode (Fig. 6A). As expected, two ions of m/z 266.1237 and m/z 150.2392 were observed for the putative m6dA in genomic DNA of 293T cells under full scan mode (Fig. 6B). And further fragmentation of m6dA base ion was conducted. The results showed that fragmentation patterns of m6dA standard and putative m6dA in genomic DNA of 293T cells were identical (Fig. 6C and D), which further confirmed the existence of m6dA in genomic DNA of 293T cells.
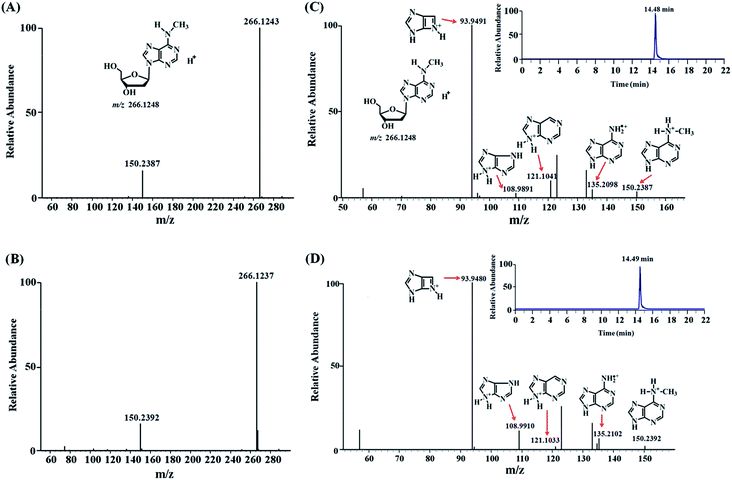 |
| Fig. 6 Analysis of m6dA by high-resolution mass spectrometry. (A) Full scan spectrum of m6dA standard. (B) Full scan spectrum of putative m6dA in genomic DNA of 293T cells. (C) Product ion spectrum of m6dA standard. (D) Product ion spectrum of putative m6dA in genomic DNA of 293T cells. | |
Widespread existence of m6dA in genomic DNA of higher eukaryotes
To quantify the content of m6dA in genomic DNA of higher eukaryotes, we first made the calibration curve, which was constructed by plotting the mean peak area ratio of m6dA dA−1 versus the mean molar ratio of m6dA dA−1 based on data obtained from triplicate measurements. The results showed that good linearities within the range of 0.00005–0.002% and 0.002–0.5% of m6dA dA−1 were obtained with correlation coefficients (R) being greater than 0.99 (Table 1). Limit of detection (LOD) and limit of quantification (LOQ) for m6dA were calculated as the amounts of the analyte at signal/noise ratios of 3 and 10, respectively. The LOD and LOQ were 0.42 fmol and 1.38 fmol for m6dA, respectively (Table 1), with which as low as 0.00001% m6dA (m6dA dA−1) can be determined using 5 μg genomic DNA.
Table 1 Linearity, LOD and LOQ of m6dA by LC-ESI-MS/MS analysis
Linear range (m6dA dA−1, %) |
Regression line |
R2 |
LOD (fmol) |
LOQ (fmol) |
Slope |
Intercept |
0.00005–0.002 |
0.1506 |
0.000087 |
0.9983 |
0.42 |
1.38 |
0.002–0.5 |
0.1271 |
0.000134 |
0.9997 |
|
|
We then further quantified m6dA in genomic DNA of various higher eukaryotes, including 2 human cell lines, 7 rat tissues and 2 kinds of plants. After Dpn I cleavage, ultrafiltration, and enzymatic digestion, the resulting nucleosides were subjected to LC-ESI-MS/MS analysis. Our results showed that m6dA can be detected in all the examined samples with m6dA contents ranging from 0.00006 to 0.00077% (m6dA dA−1) (Table 2). Shown in Fig. 7 are the typical MRM chromatograms of nucleosides of 293T cells, rat heart tissue and Oryza sativa leaves. These results demonstrated that m6dA widely exists in higher eukaryotes, which constituted the first and essential step toward understanding the functions of DNA adenine methylation in higher eukaryotes.
Table 2 m6dA contents in genomic DNA of mammals and plants
|
Sample name |
m6dA dA−1, % |
Human cells |
293T |
0.00017 ± 0.00001 |
Jurkat-T |
0.00023 ± 0.000001 |
Rat tissues |
Heart |
0.00032 ± 0.00003 |
Liver |
0.00013 ± 0.00001 |
Spleen |
0.00009 ± 0.00001 |
Lung |
0.00008 ± 0.000003 |
Kidney |
0.00006 ± 0.00001 |
Brain |
0.00013 ± 0.00002 |
Subcutis |
0.00013 ± 0.00002 |
Plants |
Leaves of Oryza sativa |
0.00077 ± 0.000004 |
Kernels of Zea mays |
0.00015 ± 0.00003 |
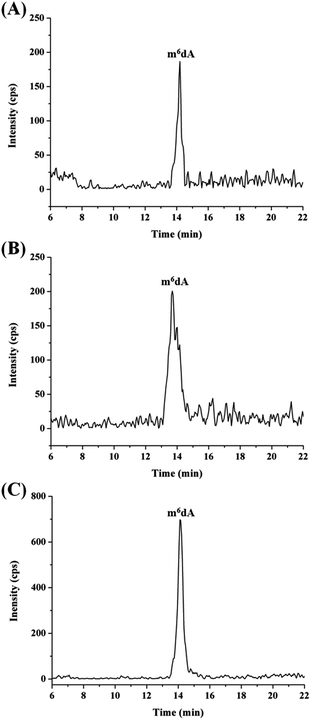 |
| Fig. 7 Typical MRM chromatograms for determination of m6dA in genomic DNA of mammals and plants. (A) 293T cells. (B) Rat heart tissue. (C) Oryza sativa leaves. | |
m6dA contents decreased in genomic DNA of diabetic patients
Previously Jia et al. reported that the methyl group of m6A in RNA can be enzymatically removed by FTO protein.39 In addition, they found that FTO protein also can remove the methyl group of m6dA in synthetic DNA, indicating m6A and m6dA may share the same demethylase of FTO due to their similar chemical structure. On the other side, our recent study demonstrated that m6A contents in RNA from the peripheral blood cells of type 2 diabetes mellitus (T2DM) patients were significantly lower compared to the healthy controls and T2DM can be characterized by the content of m6A.35 The decreased m6A in T2DM patients resulted from the significantly increased expression of FTO that is responsible for the demethylation of m6A. In this respect, since the m6A demethylase of FTO could also function on m6dA in synthetic DNA,39 we hypothesize that m6dA contents in DNA from the peripheral blood cells of T2DM patients may also decrease like m6A in RNA compared to the healthy controls.
In this respect, we then analyzed m6A and m6dA contents in RNA and DNA, respectively, from 15 T2DM patients and 15 control subjects by LC-ESI-MS/MS (Table S2 in ESI†). The mean content of m6A in RNA of T2DM patients (0.12 ± 0.02%) is significantly lower compared to the controls (0.18 ± 0.04%, p = 8.9 × 10−7) (Fig. 8A), which is consistent with our previous study.35 As expected, the mean content of m6dA in DNA of T2DM patients (0.00028 ± 0.00003%) is also significantly lower compared to the controls (0.00033 ± 0.00006%, p = 0.004) (Fig. 8B), indicating that the decrease of both m6A and m6dA could be due to the increased level of FTO in T2DM patients.
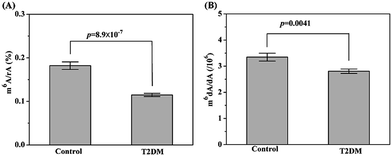 |
| Fig. 8 Quantification and statistical analysis of RNA adenine methylation and DNA adenine methylation in T2DM patients (n = 15) and control subjects (n = 15). (A) The average contents of m6A in RNA of T2DM patients and control subjects. (B) The average contents of m6dA in DNA of T2DM patients and control subjects. | |
m6dA contents change in FTO knockdown and overexpression cell line
Here we also evaluated the m6dA contents change in FTO knockdown as well as overexpression cell line. The RT-qPCR results showed that FTO expression levels were significantly lower in FTO knockdown cells and significantly higher in FTO overexpression cells (Fig. S2†). As expected, the results showed m6dA contents increased in FTO knockdown cell line and decreased in FTO overexpression cell line (Fig. 9A). In addition, m6A from RNA also exhibited the similar trend of contents change as that of m6dA (Fig. 9B). The results further suggested that m6dA and m6A may share the same demethylase of FTO. On the other side, the m6dA contents change induced by FTO knockdown and overexpression further supported the real existence of m6dA in genomic DNA of mammalian cells because m6dA contents will not change with FTO knockdown or overexpression if m6dA is from bacterial DNA.
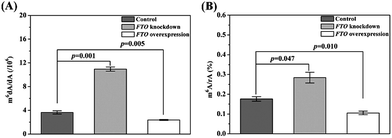 |
| Fig. 9 FTO regulates m6dA and m6A contents in DNA and RNA, respectively. (A) m6dA contents change upon FTO gene knockdown and overexpression. (B) m6A contents change upon FTO gene knockdown and overexpression. | |
Conclusion
Like DNA cytosine methylation (5-mC) that has long been recognized as an important epigenetic modification in genomes, DNA adenine methylation (m6dA) may also play critical regulatory roles in cellular processes. By developing a Dpn I cleavage coupled with size-exclusion ultrafiltration method, we were able to efficiently eliminate the possible bacterial DNA contamination in eukaryotic DNA. Using the developed Dpn I cleavage coupled with size-exclusion ultrafiltration method and high-resolution mass spectrometry analysis, we demonstrated the universal presence of DNA adenine methylation in higher eukaryotes in the current study, which constitutes the first and essential step toward understanding the functions of DNA adenine methylation in higher eukaryotes. In future study, the genome-wide localization analysis of DNA adenine methylation could offer new insight on their functions in cellular and pathological processes.
Acknowledgements
The authors thank the financial support from the National Basic Research Program of China (973 Program) (2012CB720601) and the National Natural Science Foundation of China (21205091, 81271919, 81472023). And we thank Dr Hao-Yang Wang (Shanghai Institute of Organic Chemistry, Chinese Academy of Sciences) for the help on the interpretation of the high-resolution mass spectrometry of m6dA.
References
- D. Ratel, J. L. Ravanat, F. Berger and D. Wion, BioEssays, 2006, 28, 309–315 CrossRef CAS PubMed.
- G. Bertani and J. J. Weigle, J. Bacteriol., 1953, 65, 113–121 CAS.
- S. E. Luria and M. L. Human, J. Bacteriol., 1952, 64, 557–569 CAS.
- T. Oshima, C. Wada, Y. Kawagoe, T. Ara, M. Maeda, Y. Masuda, S. Hiraga and H. Mori, Mol. Microbiol., 2002, 45, 673–695 CrossRef CAS PubMed.
- F. Barras and M. G. Marinus, Trends Genet., 1989, 5, 139–143 CrossRef CAS PubMed.
- E. Boye and A. Lobner-Olesen, Cell, 1990, 62, 981–989 CrossRef CAS PubMed.
- D. W. Russell and N. D. Zinder, Cell, 1987, 50, 1071–1079 CrossRef CAS PubMed.
- M. G. Marinus, A. Poteete and J. A. Arraj, Gene, 1984, 28, 123–125 CrossRef CAS PubMed.
- S. Hattman, C. Kenny, L. Berger and K. Pratt, J. Bacteriol., 1978, 135, 1156–1157 CAS.
- E. E. Capowski, J. M. Wells, G. S. Harrison and K. M. Karrer, Mol. Cell. Biol., 1989, 9, 2598–2605 CrossRef CAS PubMed.
- S. Hattman, Biochemistry, 2005, 70, 550–558 CAS.
- C. W. Achwal, C. A. Iyer and H. S. Chandra, FEBS Lett., 1983, 158, 353–358 CrossRef CAS PubMed.
- Y. Fu, G. Z. Luo, K. Chen, X. Deng, M. Yu, D. Han, Z. Hao, J. Liu, X. Lu, L. C. Dore, X. Weng, Q. Ji, L. Mets and C. He, Cell, 2015, 161, 879–892 CrossRef CAS PubMed.
- G. Zhang, H. Huang, D. Liu, Y. Cheng, X. Liu, W. Zhang, R. Yin, D. Zhang, P. Zhang, J. Liu, C. Li, B. Liu, Y. Luo, Y. Zhu, N. Zhang, S. He, C. He, H. Wang and D. Chen, Cell, 2015, 161, 893–906 CrossRef CAS PubMed.
- E. L. Greer, M. A. Blanco, L. Gu, E. Sendinc, J. Liu, D. Aristizabal-Corrales, C. H. Hsu, L. Aravind, C. He and Y. Shi, Cell, 2015, 161, 868–878 CrossRef CAS PubMed.
- G. Egger, G. Liang, A. Aparicio and P. A. Jones, Nature, 2004, 429, 457–463 CrossRef CAS PubMed.
- B. F. Yuan, Adv. Clin. Chem., 2014, 67, 151–187 Search PubMed.
- Y. Tang, X. D. Gao, Y. Wang, B. F. Yuan and Y. Q. Feng, Anal. Chem., 2012, 84, 7249–7255 CrossRef CAS PubMed.
- B. F. Yuan and Y. Q. Feng, TrAC, Trends Anal. Chem., 2014, 54, 24–35 CrossRef CAS.
- A. Rottach, H. Leonhardt and F. Spada, J. Cell. Biochem., 2009, 108, 43–51 CrossRef CAS PubMed.
- P. D. Lawley, A. R. Crathorn, S. A. Shah and B. A. Smith, Biochem. J., 1972, 128, 133–138 CrossRef CAS PubMed.
- B. F. Vanyushin, S. G. Tkacheva and A. N. Belozersky, Nature, 1970, 225, 948–949 CrossRef CAS PubMed.
- A. M. Krais, M. G. Cornelius and H. H. Schmeiser, Electrophoresis, 2010, 31, 3548–3551 CrossRef CAS PubMed.
- M. Munzel, D. Globisch and T. Carell, Angew. Chem., Int. Ed. Engl., 2011, 50, 6460–6468 CrossRef PubMed.
- M. R. Branco, G. Ficz and W. Reik, Nat. Rev. Genet., 2012, 13, 7–13 CAS.
- G. Jia, Y. Fu and C. He, Trends Genet., 2013, 29, 108–115 CrossRef CAS PubMed.
- C. M. Wei, A. Gershowitz and B. Moss, Cell, 1975, 4, 379–386 CrossRef CAS PubMed.
- R. Desrosiers, K. Friderici and F. Rottman, Proc. Natl. Acad. Sci. U. S. A., 1974, 71, 3971–3975 CrossRef CAS.
- K. D. Meyer, Y. Saletore, P. Zumbo, O. Elemento, C. E. Mason and S. R. Jaffrey, Cell, 2012, 149, 1635–1646 CrossRef CAS PubMed.
- D. Dominissini, S. Moshitch-Moshkovitz, S. Schwartz, M. Salmon-Divon, L. Ungar, S. Osenberg, K. Cesarkas, J. Jacob-Hirsch, N. Amariglio, M. Kupiec, R. Sorek and G. Rechavi, Nature, 2012, 485, 201–206 CrossRef CAS PubMed.
- J. M. Fustin, M. Doi, Y. Yamaguchi, H. Hida, S. Nishimura, M. Yoshida, T. Isagawa, M. S. Morioka, H. Kakeya, I. Manabe and H. Okamura, Cell, 2013, 155, 793–806 CrossRef CAS PubMed.
- S. Schwartz, S. D. Agarwala, M. R. Mumbach, M. Jovanovic, P. Mertins, A. Shishkin, Y. Tabach, T. S. Mikkelsen, R. Satija, G. Ruvkun, S. A. Carr, E. S. Lander, G. R. Fink and A. Regev, Cell, 2013, 155, 1409–1421 CrossRef CAS PubMed.
- Y. Fu, D. Dominissini, G. Rechavi and C. He, Nat. Rev. Genet., 2014, 15, 293–306 CrossRef CAS PubMed.
- X. Wang, Z. Lu, A. Gomez, G. C. Hon, Y. Yue, D. Han, Y. Fu, M. Parisien, Q. Dai, G. Jia, B. Ren, T. Pan and C. He, Nature, 2014, 505, 117–120 CrossRef PubMed.
- F. Shen, W. Huang, J. T. Huang, J. Xiong, Y. Yang, K. Wu, G. F. Jia, J. Chen, Y. Q. Feng, B. F. Yuan and S. M. Liu, J. Clin. Endocrinol. Metab., 2015, 100, E148–E154 CrossRef CAS PubMed.
- Y. Tang, J. M. Chu, W. Huang, J. Xiong, X. W. Xing, X. Zhou, Y. Q. Feng and B. F. Yuan, Anal. Chem., 2013, 85, 6129–6135 CrossRef CAS PubMed.
- X. W. Xing, Y. L. Liu, M. Vargas, Y. Wang, Y. Q. Feng, X. Zhou and B. F. Yuan, PLoS One, 2013, 8, e72993 CAS.
- Y. Tang, J. Xiong, H. P. Jiang, S. J. Zheng, Y. Q. Feng and B. F. Yuan, Anal. Chem., 2014, 86, 7764–7772 CrossRef CAS PubMed.
- G. Jia, Y. Fu, X. Zhao, Q. Dai, G. Zheng, Y. Yang, C. Yi, T. Lindahl, T. Pan, Y. G. Yang and C. He, Nat. Chem. Biol., 2011, 7, 885–887 CrossRef CAS PubMed.
- M. L. Chen, F. Shen, W. Huang, J. H. Qi, Y. Wang, Y. Q. Feng, S. M. Liu and B. F. Yuan, Clin. Chem., 2013, 59, 824–832 CAS.
- X. T. Peng, B. F. Yuan and Y. Q. Feng, J. Sep. Sci., 2011, 34, 3123–3130 Search PubMed.
- P. Liu, X.-H. Yang, Q. Wang, J. Huang, J.-B. Liu, Y. Zhu, L.-L. He and K.-M. Wang, Chin. Chem. Lett., 2014, 25, 1047–1051 CrossRef CAS.
- X. W. Xing, F. Tang, J. Wu, J. M. Chu, Y. Q. Feng, X. Zhou and B. F. Yuan, Anal. Chem., 2014, 86, 11269–11274 CrossRef CAS PubMed.
Footnote |
† Electronic supplementary information (ESI) available: See DOI: 10.1039/c5ra05307b |
|
This journal is © The Royal Society of Chemistry 2015 |