DOI:
10.1039/C5RA05051K
(Review Article)
RSC Adv., 2015,
5, 49521-49533
Doped graphene: synthesis, properties and bioanalysis
Received
22nd March 2015
, Accepted 8th May 2015
First published on 8th May 2015
Abstract
Graphene has attracted an enormous amount of interest because of its excellent properties. The relatively versatile possibilities in the doping of graphene sheets afford them with tuneable electronic properties, and provide a simple but efficient approach in tuning their catalytic activity at an atomic level. Recently, a lot of efforts have been carried out to further tailor the electronic and catalytic properties of graphene by doping, realizing the precise structure control and superior characteristics of graphene, which also broadens their applications in nanoelectronics and optoelectronics. In this review, the synthesis methods developed during the last decade for the fabrication of doped graphene with excellent properties are summarized. Moreover, as a rising and brilliant research direction, the bioanalysis applications based on doped graphene also were demonstrated.
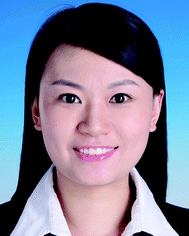 Wenyan Zhang | Wenyan Zhang received her bachelor’s degree in Chemistry from Capital Normal University in 2012. She is currently a graduate M.Sc student in Analytical Chemistry under the supervision of Dr Yang Liu at Tsinghua University. Her research focused on graphene-based materials for bioanalysis. |
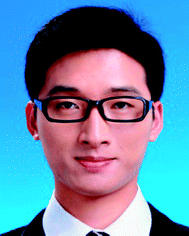 Longfei Wu | Longfei Wu received his bachelor’s degree in Metallurgical Engineering from Central South University in 2011. He received his M.Sc degree in Analytical Chemistry from Tsinghua University in 2014. His research focused on graphene-based materials for electrocatalytic applications. In September 2014, he started his PhD study at Technical University of Eindhoven. |
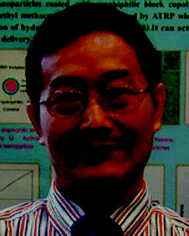 Zhaolong Li | Prof. Zhaolong Li received his BSc in 1978 from Lanzhou University, and his PhD from Lanzhou University in 1995. After that, he joined the Chemistry Department of Tsinghua University. His research interests include focuses on physical organic chemistry, peptide self-formation from active amino acids or highly coordinated phosphorus. |
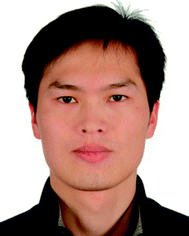 Yang Liu | Prof. Yang Liu received his BSc in 2001 from Huazhong University of Science and Technology, and his PhD from Changchun Institute of Applied Chemistry, Chinese Academy of Sciences, China in 2006. After postdoctoral experience at the University of California at Riverside and Oakland University, he joined the Chemistry Department of Tsinghua University in 2009. His research interests include focuses on electroanalytical chemistry, nanomaterial electrochemistry and biosensors. He has published more than 60 papers in international peer-reviewed journals, cited over 2300 times. |
1. Introduction
Graphene is a two-dimensional monolayer of sp2-hybridized carbon atoms arranged on a honeycomb lattice. Since its discovery in 2004,1 graphene has aroused an enormous amount of interest because of its unique physical and chemical properties such as superior mechanical strength, good thermal conductivity,2 high surface area,3 excellent electron transfer rate and ease of functionalization.4,5 These properties provide possibilities for many potential applications including Field-Effect Transistors (FETs), supercapacitors and sensors. The properties and morphologies of graphene obtained via different methods are distinct from each other. For example, graphene made from chemical vapor deposition (CVD), a bottom-up approach, is generally single and few layer sheets and possesses a large surface area, high-quality and outstanding electrical conductivity and optical transparency. Besides, graphene made from exfoliation, a top-down approach, is easy to implementation with high yields.2 In addition, the properties of graphene are reflected by its structure. As the s, px and py atomic orbitals on each carbon atom in graphene form three strong σ bonds with three other surrounding atoms, the remaining pz orbital on each carbon atom overlaps with that of neighbouring carbon atoms to produce a filled band of π orbitals (the valence band) and an empty band of π* orbitals (the conduction band). When the valence bands (VBs) and conduction bands (CBs) touch at the Brillouin zone corners, the Fermi level of graphene locates near the Dirac point, shown in Fig. 1A. Consequently, pristine graphene is a zero band gap semimetal,6 which limits its potential use in electronic and biosensing applications. Hence, many methods have been proposed to tune the band gap by shifting the Dirac point relative to the Fermi level. When the Dirac point is above (below) the Fermi level, p-type (n-type) doped graphene can be obtained (Fig. 1B).7,8,106 Among these methods, chemical doping is an effective approach.
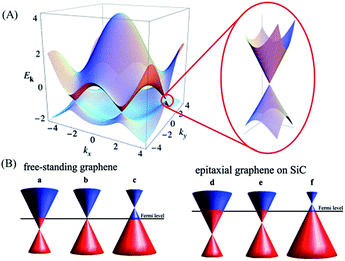 |
| Fig. 1 (A) Left: electronic dispersion in the honeycomb lattice. Right: zoom-in of the energy bands close to one of the Dirac points. (B) A schematic diagram of the position of the Dirac point and the Fermi level as a function of doping. Left: n-type doped, pristine and p-type doped free-standing graphene (a–c). Right: n-type doped, pristine and p-type doped epitaxial graphene grown on silicon carbide (SiC) (d–f). Reprinted with permission from ref. 6, copyright (2011) Royal Society of Chemistry. | |
Chemical doping can be classified into two categories: surface transfer doping and substitutional doping.9 Surface transfer doping is achieved by the charge transfer between graphene and dopants adsorbed on the surface of graphene. While the highest occupied molecular orbital (HOMO) of a dopant is above the Fermi level of graphene, dopants donate electrons to the graphene layer, resulting in the Dirac points below the Fermi level of graphene and obtaining n-type doping. While the lowest unoccupied molecular orbital (LUMO) of the dopant is below the Fermi level of graphene, dopants withdraw electrons from the graphene layer, resulting in the Dirac points above the Fermi level of graphene and obtaining p-type doping.6 In most cases, this route doesn’t destroy the chemical bonds of graphene. As for substitutional doping, heteroatoms such as nitrogen, boron, and sulfur, replace the carbon atoms in the skeleton and break the structure of graphene. Atoms with fewer valence electrons than carbon, like boron, will lead to p-type doped graphene, and atoms with more valence electrons than carbon, like nitrogen, will lead to n-type doped graphene.
After doping, the graphene exhibits more fascinating photo/electronic properties. Doping will influence the charge distribution of carbon atoms in graphene, which makes graphene a good substrate for FET fabrication.10 Moreover, the defects induced during the doping process are generally the active centres in graphene for the surface chemical reaction. Thus, the doped graphene usually exhibits excellent catalytic behaviours, which shows great promise in bioanalysis.11–14
In this review, we summarize recent works in the preparation of doped graphene and demonstrate its unique properties, which may give valuable guidance for the preparation of novel doped graphene nanomaterials with controlled structures and tuneable properties (Fig. 2). Furthermore, we demonstrate the bioanalysis applications of doped graphene for the determination of small molecules, proteins and cells. Finally, we discuss the future prospects of doped graphene.
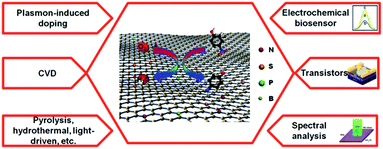 |
| Fig. 2 Synthesis methods of doped graphene and its applications. | |
2. Synthesis of doped graphene
2.1 Surface transfer doping
For surface transfer doping, molecules with electron withdrawing groups adsorbed on the surface of graphene lead to p-type doped graphene, while molecules with electron donating groups adsorbed on the surface of graphene induce n-type doped graphene. As graphene has a high surface area, it is easy for molecules to be adsorbed, including gases, organic molecules and metal atoms.
Water vapor in the environment can induce p-type doping. Yavari et al.15 reported that the band gap of graphene can be opened when it is exposed to an absolute humidity level. Meanwhile, the amount of water adsorbed on the graphene surface can be controlled precisely by adjusting the absolute humidity level. As this effect was reversible, the band gap reduced to about 0.029 eV in a vacuum. Schedin et al.16 reported that a NO2 molecule is also a strong electron acceptor, which can accept electrons from graphene to obtain p-type doping. Besides, Br2 and I2 are also more electronegative than graphene, inducing p-type doping of graphene.17 As p-type graphene can be easily prepared under air and oxygen atmospheres, it is imperative for the complementary circuit to develop n-type semiconducting graphene.18 Molecules with strong donating groups, such as ethanol, NH3 and CO, can induce n-type doped graphene.16
Other than gas molecules, organic molecules also have the ability to tune the band gap of graphene. Tetrafluoro-tetracyanoquinodimethane (F4-TCNQ) is a strong electron acceptor, which can induce p-type doping by being adsorbed on the graphene surface.19 From synchrotron-based high-resolution photoemission spectroscopy (PES), it was revealed clearly that electrons transfer from graphene to F4-TCNQ. Meanwhile, the electron accumulation and depletion only formed at the F4-TCNQ/graphene interface. Tetracyanoethylene (TCNE)20 and tetrasodium 1,3,6,8-pyrenetetrasulfonic acid (TPA)21 can also adsorb on graphene with p-type doping. Avouris’ group22 immersed graphene for 3 h in an ethanol solution containing a quantity of PEI at 300 K. As PEI is an amine-rich and electron donating polymer, graphene was n-type doped. Moreover, Wei et al.23 doped graphene utilizing a solution of 2-(2-methoxyphenyl)-1,3-dimethyl-2,3-dihydro-1H-benzoimidazole (o-MeO-DMBI). By varying the amount of o-MeO-DMBI, it is found that graphene can be controllably tuned from p-type to ambipolar and finally to n-type transport behaviours, as shown in Fig. 3. The concentration of the o-MeO-DMBI solution has an influence on the relative position between the Fermi level and the Dirac point.
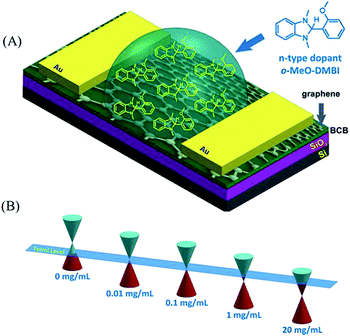 |
| Fig. 3 (A) Chemical structure of o-MeO-DMBI and the schematic illustration of a o-MeO-DMBI doped CVD-grown graphene transistor by the solution process. (B) Schematic illustration of the shifts in the Fermi level toward the Dirac point varied with the o-MeO-DMBI solution concentration. Reprinted with permission from ref. 23, copyright (2011) American Chemical Society. | |
In addition, metal atoms can also adsorb on graphene to open its band gap. Giovannetti et al.24 used DFT calculations to study the chemical doping of graphene induced by adsorption of metal on its surface. Due to the difference of the work function between graphene and metal atoms, the electrons transfer to equilibrate the Fermi levels when metal atoms adsorb on graphene. For metal–graphene equilibrium separations, graphene is n-type doped with Al, Ag, and Cu, while p-type doped with Au and Pt, as shown in Fig. 4A. Other than the work function, a graphene–metal chemical interaction also makes a contribution to the charge redistribution at the graphene–metal interface. The chemical interaction depends on the distance between graphene and metal atoms, which is mapped in Fig. 4B.
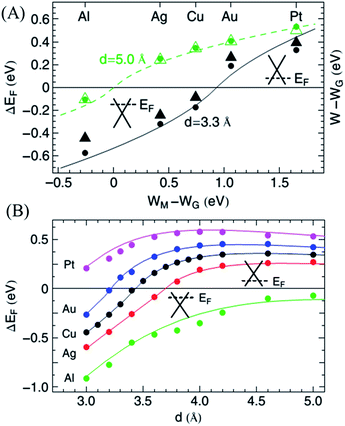 |
| Fig. 4 (A) Calculated Fermi energy shift with respect to the conical point, ΔEF (dots), and change in the work function, W − WG (triangles), as a function of WM − WG, the difference between the clean metal and graphene work functions. (B) Fermi level shifts, ΔEF (d), as a function of the graphene–metal surface distance. The dots give the calculated DFT results, and the solid lines give the results obtained from the model. Reprinted with permission from ref. 24, copyright (2008) American Physical Society. | |
2.2 Substitutional doping
Various atoms such as nitrogen, sulfur, phosphorus, boron or transition metals have been introduced into graphene, which change the electron density and broaden its applications in nanodevices and biosensors. Depending on the synthetic process, doped graphene can be prepared by two different methods: direct synthesis and post treatment. The direct synthesis approaches such as chemical vapor deposition (CVD), solvothermal and arc discharge are believed to produce homogeneous doping effects, while post treatments like thermal annealing or plasma treatment may result in surface doping only.25 The methods developed recently for doped graphene fabrication are summarized in Table 1.
Table 1 Synthesis methods of doped graphene and its applications
Synthesis methods |
Precursors |
Doping elements |
Properties |
Application |
CVD |
CH4/NH3, Cu film on Si substrate as catalyst |
N (1.2–8.9 at%) |
N-type semiconductor, lower conductivity |
FET ref. 14 |
NH3/CH4/H2/Ar (10 : 50 : 65 : 200), Ni film on SiO2/Si substrate as catalyst |
N (4 at%) |
Electrocatalytic activities |
ORR ref. 15 |
Pyridine, Cu foil as catalyst |
N (∼2.4 at%) |
N-type semiconductor, monolayer |
FET ref. 16 |
Boron powder, Cu foil as catalyst |
B (0.5 at%) |
P-type semiconductor, visible transmittance of 95.7%, Hall effect |
Solar cells ref. 17 |
Methane, boric acid powder, nitrogen gas, Cu foil as catalyst |
N (1.38 at%) |
P-type semiconductor |
Ref. 18 |
B (0.62 at%) |
Melamine diborate, nickel foam as catalyst |
N (4.5 at%) |
3D, electrocatalytic activities, thermal stabilities |
ORR ref. 19 |
B (3 at%) |
Graphite oxide, NH3/Ar |
N (2.0–2.8 at%) |
N-type semiconductor, electrocatalytic activities |
ORR ref. 20 |
GO, NH3/Ar (10% NH3) |
N (∼3–5 at%) |
N-type semiconductor |
FET ref. 21 |
Thermal annealing |
Glucose, melamine |
N (8.3–29.9 at%) |
Electrocatalytic activities |
ORR ref. 22 |
GO, boric acid, NH3 |
N |
Electrocatalytic activities |
ORR ref. 23 |
B |
Thermally exfoliated GO, (NH4)3PO4 |
N (2.9 at%) |
High capacitance retention ratio of >80% |
Ultracapacitor ref. 24 |
P (4.3 at%) |
GO, benzyl disulfide |
S (1.3–1.53 at%) |
Electrocatalytic activities |
ORR ref. 12 |
GO, CNT, diphenyl diselenide |
Se (1.05 at%) |
Electrocatalytic activities |
ORR ref. 25 |
GO, 1-methyl-3-(3-trimethoxysilyl)-propylimidazole chloride |
N (1.97–2.40 at%) Si (7.6–5.42 at%) |
P-type semiconductor, low adsorption energy |
Gas sensing ref. 26 |
Solvothermal approach |
Li3N/CCl4 (NG1) or N3C3Cl3/Li3N/CCl4 (NG2) |
N (4.5 at% (NG1) or 16.4 at% (NG2)) |
N-type semiconductor (NG), p-type semiconductor (NG2), electrocatalytic activities |
ORR ref. 27 |
BBr3, CCl4, potassium as catalyst |
B (0.51 at%) |
P-type semiconductor |
FET ref. 28 |
Arc discharge method |
Graphite/H2/He/pyridine (NG1) or graphite/H2/He/NH3 (NG2) or transformation of nano-diamond/He/pyridine (NG3) graphite/H2/He/B2H6 (BG1) or boron-packed graphite/H2/He (BG2) |
N: 0.6 at% (NG1), 1 at% (NG2), 1.4 at% (NG3) B: 1.2 at% (BG1), 3.1 at% (BG2) |
|
Ref. 29 |
Plasma treatment |
Chemically synthesized graphene, N2, plasma |
N (0.11–1.35 at%) |
Electrocatalytic activities |
Biosensor ref. 9 |
Graphene nanoribbons (GNR), NH3 plasma |
N |
N-type semiconductor |
FET ref. 29 |
2.2.1 Chemical vapor deposition (CVD). CVD is one of the most promising methods to prepare doped graphene due to its distinct advantages such as high-quality, large-scale, easy transfer and low cost.26 In this method, metals (i.e. Cu or Ni) are typically needed to grow doped graphene, which act as both the substrate and the catalysts. The precursors are the mixtures of the carbon source gas and doping elements containing gas, which are then dissociated and recombine into graphene by means of precipitation on the surface of the substrate at high temperatures. In this way, several doped graphenes have been prepared. Despite the advantages of the CVD method, the high temperature during the doping process may also result in an increased defect density or low device performance. Recently, rapid-heating plasma chemical vapor deposition (RH-PCVD) has been reported by Kato et al.,27 which grows carrier-density-controlled graphene on a SiO2 substrate at 600 °C, at a much lower temperature than that of thermal CVD graphene growth (900–1000 °C). This transport-type tuning of the doped graphene was applied to fabricate FETs, in which the charge neutral point can be shifted from the positive to the negative gate bias regions. In addition, Xue et al.28 developed a novel method to grow highly N-doped tetragonal-shaped single crystal graphene (NTSG) arrays by self-assembly of pyridine molecules on a Cu surface at 300 °C under ambient pressure. N-doped graphene in this method was tetragonal-shaped, almost single layer and single crystal with a N content of 16.7%, and realized a typical n-type electron doping performance in air.The doping efficiency of graphene is related to parameters including the catalyst, the precursor, flow rate, temperature, pressure and reaction time.29–34 Controlling the ratio between carbons and doping element sources can regulate the doping efficiency. However, two precursors may give rise to the uncontrollable growth of doped graphene because of the difference between the source of carbon and the doping elements. As a result, a sole precursor, containing both carbon and doping elements for doped graphene fabrication, was developed recently that can simultaneously introduce chemical species in a controlled manner for the large-scale growth of uniform and high-quality doped graphene.28,35,36 Wang et al.36 synthesized boron-doped graphene on a Cu surface by CVD with phenylboronic acid as the carbon and boron source. Under a hydrogen atmosphere, the phenylboronic acid was decomposed into carbonaceous, boron and oxygen species represented by grey, red and yellow, respectively, which is shown in Fig. 5A. The oxygen species were removed by the formation of H2O or COx. Then the B-doped graphene film was gradually grown on the copper surface via the graphitization of adsorbed carbonaceous and boron species. In addition, the thickness and the number of doped graphene layers can be controlled by adjusting the flow time of the precursor.37
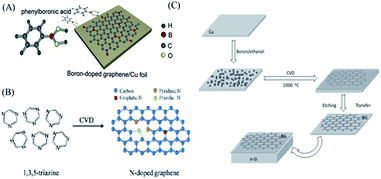 |
| Fig. 5 (A) Schematic diagram of CVD growth of boron-doped graphene. (B) Structures of 1,3,5-triazine molecules and N-doped graphene. (C) Schematic of BG preparation. Reprinted with permission from ref. 35, copyright (2013) American Chemical Society and from ref. 41, copyright (2012) Wiley, respectively. | |
The carbon source plays a critical role in the quality of the doped graphene. The conventional carbon source is CH4 or CxHy. Recently, some organics and polymers, such as pyridine,38 1,3,5-triazine35 or polypyrrole,39 have also been used. Lu et al.35 transferred the conversion of the organic molecule triazine into N-doped graphene via the CVD method with a Cu catalyst. As it is demonstrated in Fig. 5B, doping of graphitic N atoms preserved the high-quality properties of graphene without destroying the graphene crystal structure significantly.40 However, the incorporations of pyridinic and pyrrolic N increased the amount of disorder in the system with structural defects including bonding disorder and vacancies (short-range scatterers). Li et al.41 had successfully incorporated B atoms into graphene sheets with the use of boron powder as the precursor. They regarded the copper foil as a substrate to produce the BG films with high transparency on the macroscale. The scheme is exhibited in Fig. 5C. The as-prepared B-doped graphene showed p-type semiconductor features.
Moreover, Chen’s group103 fabricated nitrogen and sulfur co-doped nanoporous graphene by CVD. They utilized nanoporous Ni as both the template and substrate, with pyridine and thiophene as the carbon sources, and the nitrogen and sulfur sources, respectively. As sulfur and nitrogen atoms exhibit different electron density distributions, the Fermi level of the doped graphene is negatively shifted to 0.6 eV above the Dirac point, which could obtain high electrical conductivity. Meanwhile, the coupling of S and N dopants introduced geometric defects to the graphene lattice, and the Gibbs free energy of H* absorption was tuned and showed an outstanding hydrogen evolution reaction (HER) catalytic ability.
2.2.2 Solvothermal approach. Solvothermal synthesis has been widely used for nanomaterials preparation because of its distinct advantages such as simple operation, mild synthesis conditions, and capability to deliver relatively large quantities.42 Generally, the dopant-containing precursors act as both the reducing and doping agent in solvothermal processes.42,107 For instance, Han et al.43 presented a novel B-doped chemically modified graphene by reduction of graphene oxide with a borane (BH3)–tetrahydrofuran (THF) adduct under reflux via a one-pot process. In addition, N-doped graphene has been reported via a one-step reaction of tetrachloromethane (CCl4) with lithium nitride (Li3N).44 By mixing CCl4 with Li3N at 250 °C for 10 h, or cyanuric chloride (N3C3Cl3) with Li3N and CCl4 at 350 °C for 6 h, doped graphene with a nitrogen content in the range of 4.5–16.4 at% was obtained. On the other hand, a one-pot solvothermal process was also reported by using graphene oxide (GO) and ammonia boron trifluoride (NH3BF3) as precursors via a hydrothermal reaction, and three-dimensional (3D) nitrogen and boron co-doped monolithic graphene aerogels were obtained.45 The as-prepared 3D doped aerogels show interconnected frameworks with a macroporous architecture, which greatly facilitate ion diffusion and electron transport in the bulk electrode.
2.2.3 Arc discharge method. Arc discharge has the advantages of large-scale, high-quality and purity over the CVD method, and has been widely used to prepare graphene sheets and carbon nanotubes by evaporating the carbon source and graphite at high temperature.42,46–48 Rao et al.48 prepared B- and N-doped graphene by performing the arc discharge of graphite electrodes in the presence of hydrogen (H2), helium (He), diborane (B2H6) or H2, He, pyridine vapor. However, the dopant content was at very low levels (0.5–1.5 at%). Afterwards, Liu et al.49 introduced a catalytic arc discharge method by using flake graphite as the carbon source and ZnO or ZnS as catalysts to synthesize N-doped graphene, in which the nitrogen content was as high as 4.92 at%. Moreover, the doped graphene synthesized via this method is higher quality and possesses fewer layers than that prepared through many other approaches.
2.2.4 Thermal annealing. Thermal annealing refers to the method utilizing high temperatures to incorporate various dopants into graphene.108,109 There are usually two approaches. One is to anneal graphene or graphene oxide in a dopant-containing gaseous environment. For example, Dai’s group50 developed a facile approach to fabricate a B/N co-doped graphene by thermal annealing of GO in the presence of boric acid and ammonia at 1000 °C. The BCN graphene exhibited superior electrocatalytic abilities to the commercial Pt/C electrocatalyst.Another approach is the direct pyrolysis of dopant-containing hydrocarbons or polymers in the presence of graphene, which can introduce various atoms to graphene. Ding et al.51 used aniline as a precursor to fabricate the doped graphene in layered montmorillonite, a quasi-closed flat nanoreactor, which can selectively synthesise pyridinic- and pyrrolic-nitrogen-doped graphene. This method can also realize the co-doping of two or more elements without any side products. Liang et al.52 used melamine and benzyl disulfide (BDS) as N and S precursors, respectively, and incorporated them with a GO/SiO2 nanocomposite, in which the SiO2 spheres were used as the structural template. Finally, N- and S-co-doped graphene was obtained after annealing the mixture of melamine/BDS/GO/SiO2 at high temperature. The properties of the as-prepared doped graphene are highly dependent on the pyrolysis temperatures, and an excessively low or high temperature can lead to low electronic conductivity or a remarkable loss of active sites. The presented N and S dual-doped mesoporous graphene can be used as a metal-free catalyst for oxygen reduction with high efficiency. Zheng et al.102 studied the electronic properties of different heteroatoms (N, B, O, S, P and F) in doped graphene using density functional theory (DFT) calculations. They found that N and O were negatively charged, acted as electron acceptors for the adjacent C. In contrast, F, S, B, and P were positively charged, acted as electron donors. Based on the theoretical prediction, they selected N and P atoms, which had the most noticeable differences in the charge population, to design a dual-doped graphene by annealing chemically exfoliated GO powder with a melamine (N source) and triphenylphosphine (P source) mixture at 950 °C in an Ar atmosphere. The content of the dopant was 4.60 at% of N and 1.63 at% of P, respectively. With the synergistic effect, the doped graphene was applied to enhance the HER, exhibiting a significantly improved electrochemical performance.
The shortcoming of the above methods is the relatively high working temperature. The direct thermal annealing process generally results in an irreversible stacking of graphene due to the strong π-interactions. To solve this problem, Yang et al.53 confined a GO sheet by a sandwich-like, ultrathin and porous silica shell to facilitate the thermal reaction and prevent the irreversible re-aggregation of graphene at high temperature, which can achieve nitrogen and sulfur-doped graphene with high surface areas.
2.2.5 Plasma treatment. Plasma treatment is a facile approach to incorporate foreign atoms and groups into scaffold surfaces.54 By exposing materials to an atmosphere containing doping elements under plasma treatment, the initial atoms can be partly replaced by the foreign atoms. An N-doped graphene was fabricated by the plasma treatment of graphene in a nitrogen atmosphere.11 It was found that the plasma treatment not only introduced nitrogen atoms into the host graphene but also increased the content of oxygen. In this case, the nitrogen content in graphene can be regulated by changing the plasma exposure time. In addition, Choi’s group55 prepared the N-doped graphene by firstly reducing GO with hydrogen plasma treatment and then N atom doping was realized by exposing the reduced GO (rGO) to nitrogen plasma. A mild NH3 plasma-treated graphene doping method was developed by Dai’s group.56 It was demonstrated that the position of the doping atom can be controlled by the plasma treatment conditions, and the mild-plasma caused doping defects near the edge of graphene while harsh-plasma damaged the centre of graphene.With plasmonic effects, metal-based subwavelength antennas injected non-equilibrium hot electrons into the nearby graphene structure under resonant illumination and effectively doped the graphene.57 Hence, Fang et al. obtained n-type doping of graphene by plasmon-generated hot electrons, which can be a good candidate for optoelectronic devices and sensors.
There are many other methods to dope graphene with high efficiency. Nicolle et al.58 explored the behaviour of different numbers of graphene layers supported on SiO2 under high pressure for capacitance-induced substrate-mediated charge transfer, in which n-type doped graphene was fabricated. Kim et al.59 modulated p-type doping of graphene by non-covalently latching light switchable dipolar molecules with a hole concentration of ∼5 × 1012 cm−2.
3. Properties of doped graphene
Graphene is a zero band gap semiconductor that possesses extraordinary properties such as high mobility of charge carriers, an anomalous quantum Hall effect, and superior thermal/electrical conductivity.60–64 However, the absence of a true band gap is a major drawback for the application in electronic devices (e.g. FETs). Chemical doping not only opens the band gap but also generates specific charge carriers (holes and electrons).65 Hence, doped graphene has different properties compared with graphene, shown in Table 2. The incorporation of different heteroatoms into graphene can result in the transformation of graphene into a p- or n-type semiconductor, respectively, which makes the use of a graphene nanosheet as a semiconducting channel between the source and drain in FETs possible.66 We exhibit the doping mechanism in graphene in Fig. 3. Graphene is unique for its zero-gap band structure with a zero density of states (DOS). Chemical doping can induce the desired rigid band. The Fermi level was shifted away from the Dirac point by electrons (or holes), shown as an energy shift in the carbon-projected density of states (C-PDOS). Hole (p) and electron (n) doping can be achieved by contacting the carbon layer with different metals.67,68 For n-type dopants with a HOMO (EH), the ionization potential, Ip, decreases as electrons are removed, while charges flow to the graphene from the dopants near the Fermi energy of graphene. For p-type dopants with a LUMO (EL), the electron affinity increases as electrons are added to the valence level, while charges flow to the dopants from the graphene near the Fermi energy of graphene.67 Different doping atoms can result in various bond types that have profoundly different effects on the carrier concentration or band gap etc. It has been reported that n-type semiconducting graphene is produced by nitrogen atoms donating their lone pair of electrons to the graphene system network. The lone pair of electrons in nitrogen are closely related to high electron mobility. Hence, Some et al.66 selected phosphorus with a higher donating ability of lone pair electrons to prepare much stronger n-type graphene than can be prepared with nitrogen. Otherwise, the content of dopants also strongly influenced electrochemical activity. Zhang et al.70 found that a lower N content can’t render enough electrochemical activity of N-doped graphene. However, a higher N content was able to create more active sites with low conductivity and more sites prone to poisoning. For this reason, a suitable N content with an acceptable conductivity and a considerable number of active sites is closely related to the ORR activity for doped graphene. Meanwhile, Panchakarla et al.71 also reported that the concentration of B and N affected semiconducting electronic properties.
Table 2 The properties of pristine graphene and doped graphene
|
Work function (eV) |
Resistance (Ω sq−1) |
Visible transmittance |
Hall coefficient (HC, m2 C−1) |
Ref. |
Pristine graphene |
4.2 |
1100 |
96.7% (550 nm) |
|
46 |
Nitric-doped |
|
416 |
89% |
+26.8 |
58 |
Hydrazine-doped |
|
517 |
89% |
−94.6 |
58 |
PEI-doped |
|
397 |
89% |
−38 |
58 |
AuCl3-doped |
5.0 |
500 |
94.5% (550 nm) |
|
59 |
IrCl3-doped |
4.9 |
600 |
92.5% (550 nm) |
|
59 |
MoCl3-doped |
4.8 |
720 |
86.2% (550 nm) |
|
59 |
RhCl3-doped |
5.14 |
620 |
∼90% (550 nm) |
|
59 |
The dopants also play an important role in magnetoresistance (MR). Rein et al.105 clarified the influence of nitrogen doping on the magnetotransport of single layer graphene by CVD. Compared to undoped graphene, there was a 6-fold increase in the charge carrier concentration up to 4 × 1013 cm−2 for doped graphene at room temperature. Meanwhile, due to the Lorentz MR, a positive high field MR was found on graphene. In contrast, a negative MR is observed for doped graphene at both 2.3 K and 279 K, which was ascribed to the defect boundary scattering, leading to the reversed MR.
It is known that the energy level is different between a graphene electrode and other active layers, which has prevented the application of graphene in light emitting diodes, organic light emitting diodes, or organic solar cells. This problem can be solved by modulation of the work function in the graphene layer with doping of high work function metals. Kwon et al.72 investigated six metal chlorides as p-type dopants to tune the work function of graphene. The results are listed in Table 3. It can be concluded from Table 3 that doping graphene with metal chlorides can greatly decrease its sheet resistance and transmittance. They found that RhCl3 was the strongest p-dopant due to the highest work function.
Table 3 Electronic properties of doped graphene
|
Pristine graphene |
Doped graphene |
Heteroatoms |
Application |
Ref. |
Carrier mobility (cm2 V−1 s−1) |
2 × 105 |
Up to 11.5 & 12.4 (hole & electron) |
N |
FET |
74 |
Dirac point (V) |
0 |
−16 |
N |
FET |
74 |
Resistance (Ω) |
103 |
102 to 108 |
N |
ORR |
45 |
Raman scattering |
|
10 times greater than graphene |
N |
Molecule sensing |
76 |
Similar to electronic properties, the optical properties of graphene can also be tuned by chemical doping. Bult et al.69 demonstrated chemically doped p-type and n-type graphene transparent conducting electrodes that possessed 89% visible transmittance, which are well-suited as anodes or cathodes for a variety of optoelectronic applications. It has also been reported that the photoluminescence of rGO can be quenched by doping.73 Meanwhile, the UV-vis absorption spectrum of N-doped graphene quantum dots (N-GQDs) showed a blue-shift and emitted intense blue luminescence.74,75 In addition, N-doped graphene can also enhance the Raman scattering of RhB molecules.76
4. Applications in bioanalysis
Compared to pristine graphene, the results indicate that the unoccupied DOS changes after doping, suggesting charge transfer between carbons to the dopants, which is beneficial for the electric conductivity of the materials.77 Secondly, doping can alter the surface oxygen-containing groups on graphene to improve the resistance of graphene further. Thirdly, doping graphene increases the specific surface area, which makes it easier to decorate molecules on it. Moreover, doping introduces more defects to the graphene, resulting in more catalytic sites to enhance the catalytic properties of graphene towards molecules.78,79 Hence, doped graphene has attracted an enormous amount of interest in bioanalysis applications. According to the analytical targets, we discussed the application in the following aspects, including small molecule, protein and cell sensors.
4.1 Small molecule sensors
Hydrogen peroxide (H2O2) is important in the regulation of eukaryotic signal transduction and the response to various stimuli including cytokines and growth factors, and is also an essential mediator in food, industrial, and environmental analysis.80,81 Shao et al.82 introduced a facile method to produce N-graphene by exposing a functionalized graphene sheet (FGS) in nitrogen plasma. The carbon atoms adjacent to nitrogen dopants possessed a higher positive charge density in doped graphene, which enhanced the adsorption of H2O2. Meanwhile, the nitrogen-induced charge delocalization changed the chemisorption of O2 from monoatomic end-on adsorption to diatomic side-on adsorption, which effectively weakened the O–O bond to facilitate the electrocatalytic reduction of H2O2. Furthermore, N elements in graphene also created some structural defects on graphene with an amount of unsaturated carbon atoms, which are very active to reactions with H2O2. Hence, N-graphene exhibited a great electrocatalytic activity toward H2O2 reduction. A well-defined reduction peak around −0.2 V in the CV of N-graphene with a linear relationship from 10−5 mM to 2.8 mM was observed, which was much wider than that of graphene (10−4 mM to 1.8 mM). As H2O2 is a general enzymatic product of glucose, it is supposed that doping graphene improves the catalytic activity to glucose, which is the diabetes target analyte. However, the catalytic site of glucose oxidase is seated deeply in a cavity and not easily accessible for direct electron transfer reaction, which limits the catalytic activity of glucose oxidase. The doped graphene produced by Wang et al.11 displayed good biocompatibility, high electrocatalytic activity and fast direct electron transfer kinetics for enzymes, realizing glucose biosensing with high sensitivity. As shown in Fig. 6A, they obtained N-doped graphene by nitrogen plasma treatment of graphene with regulation of the N percent in the host graphene from 0.11–1.35%. The nitrogen atom was inset into the graphite plane to form a quaternary N or graphitic nitrogen (G-N), while nitrogen atoms were doped in graphene to form pyridinic N and pyrrolic N. Due to the change of the density of electronic states (DOES) by doping effects, the functional graphene had a better performance in electrocatalysis. More importantly, the N-doped graphene showed a remarkable ability for electron transfer from the enzyme cavity to the electrode surface, resulting in a good response for glucose from 0.01 to 0.5 mM in the presence of interferences (Fig. 6B). As electron transfer is sensitive to the surface chemistry and DOES near the Fermi potential, the redox peak current is much higher for N-doped graphene than for graphene. Due to the porous structures that may offer a higher surface area for enzyme loading, Guo et al.83 proposed a new kind of enzyme carrier for biosensors by a three-dimensional (3D) network. They utilized chemical vapour deposition to prepare a highly conductive 3D nitrogen-doped graphene (3D-NG) structure, shown in Fig. 6C and D, which exhibited higher redox peak currents than those of 3D graphene. The sensitivities of the 3D-NG sensor for glucose were 226.24 μA mM−1 cm −2 for the lower concentration region and 108.94 μA mM −1 cm −2 for the higher concentration region. This was ascribed to the hydrogen bonds of the nitrogen-containing groups in 3D-NG, which can promote the adsorption of biomaterials. Moreover, by calculating the surface coverage concentration of the electroactive glucose oxidase (GOx), it’s concluded that the 3D porous structure provided more specific surface area and adsorption sites for enzyme loading.
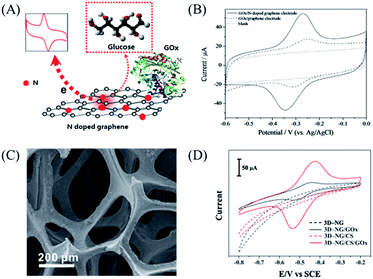 |
| Fig. 6 (A) Scheme of detection of glucose by N-doped graphene and graphene. (B) Cyclic voltammograms of GOx immobilized on an N-doped graphene electrode (solid line), a graphene electrode (dashed line) and GCE (dotted line) in an N2-saturated 0.1 M PBS solution (pH 7.0). Scan rate = 0.05 V s−1. (C) SEM image of 3D-NG. (D) Cyclic voltammograms of bare 3D-NG (black dashed curve), 3D-NG/GOx (black curve), 3D-NG/CS (red dashed curve) and 3D-NG/CS/GOx (red curve) in N2-saturated 0.1 M PBS (pH = 7.40). Scan rate = 0.1 V s−1. Reprinted with permission from ref. 11, copyright (2010) American Chemical Society and from ref. 83, copyright (2015) Royal Society of Chemistry, respectively. Abbreviation: chitosan (CS). | |
Ascorbic acid (AA), uric acid (UA) and dopamine (DA) are closely related to the physiological function of organisms and many diseases including cancer, Parkinson’s disease and cardiovascular disease.82 However, AA, DA and UA usually coexist and their oxidation potentials are severely overlapped, which make their selective detection difficult. Sheng et al.84 developed a facile approach to synthesize nitrogen-doped graphene by thermally annealing a mixture of GO and melamine. The nitrogen-doped graphene was realized to detect AA, DA and UA simultaneously with high sensitivity. The linear ranges are 5.0 × 10−6 to 1.3 × 10−3 M, 5.0 × 10−7 to 1.7 × 10−4 M and 1.0 × 10−7 to 2.0 × 10−5 M with detection limits of 2.2 × 10−6 M, 2.5 × 10−7 M and 4.5 × 10−8 M (S/N = 3) for AA, DA and UA respectively. The high electrocatalytic activity of the sensor was related to the fact that nitrogen atoms in the NG layers may interact with these molecules via hydrogen bonding, which can activate the hydroxyl and amine groups, and further accelerate the charge transfer kinetics of these molecules at the NG surface. Meanwhile, the π–π interactions between graphene layers and these molecules can also promote the charge transfer of the three molecules. The selective analysis of DA in the presence of AA and UA was also realized on the boron-doped graphene modified electrode.85 In addition, the boron-doped graphene also exhibited electrochemical activity toward the oxidization of β-nicotinamide adenine dinucleotide (NADH), which is of great interest since it is required in many dehydrogenase-based biosensors.86 Importantly, the boron-doped graphene modified electrode showed excellent performance towards NADH oxidation product fouling. The boron atoms in graphene decreased the electron density of neighbouring carbon sites, which had an impact on the chemical and electrochemical activity of the boron-doped graphene to biomolecules.
NO2− is also an important anion which has been recognized as an alarming pollutant to the environment and human health. Excessive NO2− in the human body may cause methemoglobinemia and may become a source of carcinogenic N-nitrosamines.87,88 By the π-stacking interaction between graphene and phenanthrene, Li et al.89 added graphene to a phenanthrene/K solution complex to prepare K-doped graphene, which showed a high response toward the oxidation of NO2−. Based on the K-doped graphene sensing platform, a detection limit of 0.2 μM was obtained at a signal-to-noise ratio of 3 with the linear range from 0.5 μM to 7.8 mM. The sensor was also successfully applied to determine NO2− released from liver cancer and leukemia cells, showing a good potential in biomedical applications. Different types of K species (mainly K oxides, K ions, and K metal) in graphene might introduce a change in the Fermi level and charge transfer of the graphene. As a consequence, K modification might be beneficial to regulate the electronic properties and enhance the electrocatalytic activity of graphene in electrochemical systems. This method can be extended to the chemical modification of other alkali metals, which enables the formation of the most variations of graphene.
As metal nanoparticles have a large specific active surface area, they can be distributed on graphene as tiny conducting centres. Hence, the incorporation of metal nanoparticles into graphene can decrease the energy barrier, enhance electron transfer and improve the biosensor response.78,110 In this regard, Kannan et al.78 introduced platinum nanoparticles into nitrogen-doped graphene (N-GN-PtNP) to detect homocysteine with an experimental detection limit of 200 pM. It was shown that the potential shifted to 210 mV positively and the oxidation current improved 3-fold on the N-GN-PtNP modified electrode. It was also implied by the authors that nitrogen atoms facilitate the interaction between graphene and metals, which can improve the biosensing efficiency further.
4.2 Protein sensors
Proteins are vital for gene expression mechanisms and reflect the development of diagnostics for disease states. Due to the excellent properties of graphene, such as an ambipolar electric field effect and high carrier mobility, graphene-based FETs are considered to be the most promising replacement to silicon electronics. However, the zero-gap and ambipolar characteristics have limited the application of graphene in electronics. Doping can solve these problems by injecting or extracting a carrier to tailor the electronic structure, thus tuning the band gap. Jang’s group10 applied doped graphene in a FET device to detect biomolecules with high sensitivity. They grew nitrogen-doped few layer graphene (PPy-NDFLG) with polypyrrole as the solid source by the CVD method. After nitrogen doping, there were three kinds of N atoms in the functional graphene exhibiting stable n-type behaviour, shown in Fig. 7B. They utilized PPy-NDFLG as a signal transducer integrated with an anti-VEGF RNA aptamer into a flexible FET platform to detect vascular endothelial growth factor (VEGF), which is useful for the early diagnosis, staging and monitoring of cancers (Fig. 7C). It is shown in Fig. 7A that the anti-VEGF RNA aptamer was integrated by modifying the plane of PPy-NDFLG with glutaraldehyde conjugated with 1,5-diaminonaphthalene (DAN) through a Schiff-base reaction.90–94 As the interactions between the aptamer and VEGF affected the charge-carrier density on the surface of PPy-NDFLG, the electronic control was realized. This concentration-dependent sensor gave a rapid response time of less than 1 s with a detection limit of 100 fM, which was approximately 1–3 orders of magnitude lower than that of conventional VEGF sensors (Fig. 7D). Moreover, the FET-type aptasensor displayed outstanding mechanical flexibility making it suitable for portable fabrication and implantable sensors.
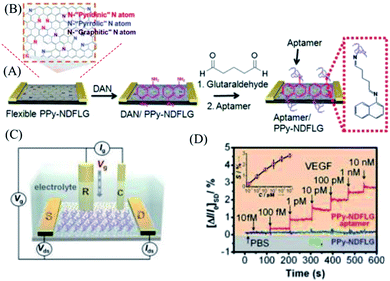 |
| Fig. 7 (A) Schematic illustration of reaction steps for the fabrication of aptasensor platforms based on PPy-NDFLG conjugated with an anti-VEGF RNA aptamer. (B) Structure diagram of PPy-NDFLG. (C) Schematic diagram of a liquid-ion gated FET using an aptamer conjugated with PPy-NDFLG. (D) Real-time responses and a calibration curve of aptasensors with various vascular endothelial growth factor concentrations. Reprinted with permission from ref. 10, copyright (2012) American Chemical Society. | |
For biological recognition events, the electrochemiluminescence (ECL) technique is a versatile method with a low background signal, desirable sensitivity and good analytical performance. In the ECL process, the key factor to affect the signal is the electron transfer reaction between formed nanocrystal species and co-reactants. To enhance the signal, the nanocrystals hold great promise for realizing a sensor’s potential applications with their remarkable electronic activities, chemical stability, and large surface area. Wang et al.95 developed metal K-doped graphene with SiO2@CdS nanocomposites to fabricate a sensitive ECL sensor successfully (Fig. 8). They found that the doped K in graphene with three species (K cations, K oxides and K metal) not only accelerated the electron transfer in the ECL reaction, but also anchored more SiO2@CdS/DNA composites due to its high surface/volume ratio. They applied this sensor to detect the ubiquitous transcriptional factor TATA-binding protein (TBP). The detection limit of TBP in this work was found at levels down to 0.02 nM, which was superior to that obtained from electrochemical assay (3 nM). To improve the ECL efficiency and stability of luminophores, Wang’s group104 introduced silver bromide nanoparticles (AgBr NPs) on nitrogen-doped graphene by the water bath method. The AgBr˙− radical released an electron, which came from the oxidizing agent’s SO4˙− radicals, to form the AgBr* for ECL emission. In this system, nitrogen-doped graphene worked as an electron reservoir to accept the electron from the CB of n-type AgBr, which can avoid Ag+ capturing the photogenerated electrons.
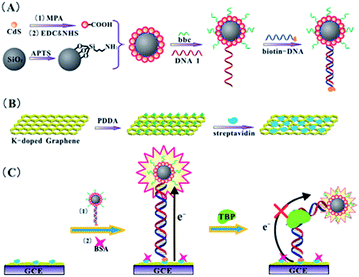 |
| Fig. 8 The analytical procedure of the amplified ECL TBP assay. Reprinted with permission from ref. 95, copyright (2012) Royal Society of Chemistry. | |
In order to acquire more chemically active sites, further functionalization on doped graphene can be carried out to achieve better electrochemical performances. Generally, there are some functional groups such as CN, NH3 or SH in the proteins, and the active groups are easy to coordinate with metals, which can not only increase the surface area of the nanocomposites but also improve the captured amounts of proteins. Matrix metalloproteinase-2 (MMP-2), a protease related to tumor growth, invasion, reproduction and tissue remodeling, is secreted by malignant tumor cells. It is of great importance to analyze MMP-2 sensitively in the early detection of disease. In this regard, Zhu’s group96 introduced Au nanoparticles on a nitrogen-doped graphene network to fabricate an ultrasensitive electrochemical immunosensor to detect MMP-2. It was demonstrated that the hydrophobicity and hence biocompatibility were improved by the incorporation of Au on doped graphene, making it more suitable for protein loading. Consequently, the immunosensor showed linear detection, ranging from 0.0005 to 50 ng mL−1 with a detection limit of 0.11 pg mL−1. The detection limit of previous reports was 0.02 ng mL−1, much higher than that of this proposed system. The doped graphene composites were also employed in the fabrication of a non-mediator electrochemical biosensor for the detection of several biomarkers, such as alpha-fetoprotein (AFP)97 and zearalenone.98 To produce a higher catalytic efficiency, Ju’s group99 assembled hemin on N-doped graphene (hemin@NG) to quench the ECL emission of QDs by electrocatalytically reducing the dissolved oxygen, the ECL co-reactant. Due to the doping of nitrogen, the N atoms acted as the electron donors to promote the reactivity of adsorbed molecules. Meanwhile, hemin is normally used as an HRP mimic, owing to its capability as an oxygen reduction catalyst. The N defective sites on graphene can coordinate with the iron active centre in hemin for better immobilization, which can facilitate the electrocatalytic reduction. This new ultrasensitive immunoassay sensor was used to detect a carcinoembryonic antigen (CEA), showing a detection limit of sub-picogram per milliliter with a linear range of over five orders of magnitude.
4.3 Cell sensors
Due to the growth, metabolism and apoptosis of cells being closely related to biological environments in organisms, it’s of great significance to visualize and monitor physiological events to understand cell biology. The physiological processes or diseases are related to special biomolecules, which serve as biomarkers. By introducing probes, these biomarkers can be traced to reveal the activities of living cells at a molecular level, especially for tumors. Thus, they play an important role in the discovery and treatment of diseases. With non-invasiveness and high sensitivity, fluorescence spectroscopic techniques offer excellent observation for intracellular signaling and intracellular analysis.
Two-photon fluorescence imaging (TPFI) is suitable for in vivo cell bioimaging because of a larger penetration depth, a minimized tissue autofluorescence background and minimal photodamage to biotissues. The two-photon fluorescent probes with enough brightness are essential for scattering deep tissues. With bleaching, low cytotoxicity and excellent biocompatibility, graphene quantum dots (GQDs) are one of the most ideal options for two-photon fluorescent probes. After nitrogen doping, strong electron donating groups (such as dimethylamido) can be introduced to GQDs. The lone pair of electrons from dimethylamido formed the p–π conjugation with the aromatic rings to enlarge the π-conjugated system. The primary HOMO was elevated to a higher energy orbit by the strong orbital interaction, which further decreased the band gap and red-shifted the fluorescence emission. Furthermore, the large π-conjugated system facilitated the charge transfer efficiency and enhanced the two-photon absorption, which provided strong two-photon-induced fluorescence for N-GQDs. Gong75 used N-GQDs as two-photon fluorescent probes for cellular and deep-tissue imaging. As is shown in Fig. 9A, the lone pair of electrons from the donating group dimethylamido were doped to the plane of the N-GQDs, which can enlarge the π-conjugated system. The primary HOMO (blue dashed line in Fig. 9A) was promoted to a higher energy orbit HOMO (black solid line) by the orbital interaction between dimethylamido and the π-conjugated system of the N-GQD. Then, the band gap decreased and fluorescence emission was red-shifted. Furthermore, dimethylamido and the π-conjugated system of the N-GQD can facilitate the charge transfer efficiency and the two-photon absorption. They used DMF as the source of nitrogen and GO as the carbon source to fabricate N-GQDs. The N-GQDs showed a two-photon absorption cross section as high as 48
000 GM and a large imaging penetration depth of 1800 μm (Fig. 9B and C).
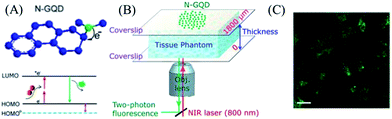 |
| Fig. 9 (A) Fluorescence mechanism of N-GQD. (B) Schematic of the setup used for TPFI of N-GQDs in tissue phantom with different thicknesses. (C) Two-photon cell imaging under 800 nm excitation. Reprinted with permission from ref. 75, copyright (2013) American Chemical Society. | |
Considering the excellent properties of carbon nanomaterials in biosensing applications, the doped carbon nanomaterials have drawn much attention in cell imaging applications due to their high quantum efficiency and biocompatibility. For example, Si-doped carbon quantum dots100 and nitrogen-doped carbonaceous nanospheres101 have been successfully applied for bioimaging in cells. However, it is still at an initial stage for carbon nanomaterials in applications as fluorescent chemosensors to monitor and image biological processes. It is anticipated that doped graphene possesses a brilliant future in monitoring biological activity of deep tissues and detecting diseases in living biosystems.
5. Conclusions and perspective
In summary, we represent an overview of the recent development in doped graphene. Various synthesis methods have been reported to obtain heteroatom-doped graphene with a tuned band gap. Endowed with a real band gap, it can be used as p-type or n-type semiconductor. Compared to pristine graphene, both the physical and chemical properties of doped graphene can be tailored. The dopants changed the electron density of neighbouring carbon atoms, which determined its physical properties such as electrical conductivity and transparency. Meanwhile, dopants can act as active sites for catalysis in electrochemical sensors. In future work, key insights into the doping mechanism such as the bonding type, the dopant location and the influencing factors of doping should be further explored.
Besides the applications of doped graphene in energy related areas, the extraordinary properties make it a promising material for bioanalysis. The increased active sites caused by heteroatoms show excellent activity towards small molecules. In addition, doped graphene endowed with a real band gap is an ideal material for FETs and ECL, which improves the detection limit for proteins or cells by several orders of magnitude. We believe there will be more doped graphene-based methods developed for biomolecule detection in the future.
Acknowledgements
This work was financially supported by the National Basic Research Program of China (no. 2011CB935704, no. 2013CB934004), the National Natural Science Foundation of China (no. 21375073, no. 21235004, 21327806, no. 11079002), the Research Fund of Ministry of Education of China (no. 20110002130007) and Tsinghua University Initiative Scientific Research Program (no. 2014z21027).
References
- K. S. Novoselov, A. K. Geim, S. V. Morozov, D. Jiang, Y. Zhang and S. V. Dubonos, Science, 2004, 306, 666 CrossRef CAS PubMed.
- X. Huang, X. Qi, F. Boey and H. Zhang, Chem. Soc. Rev., 2012, 41, 666 RSC.
- M. D. Stoller, S. Park, Y. Zhu, J. An and R. S. Ruoff, Nano Lett., 2008, 8, 3498 CrossRef CAS PubMed.
- C. Cheng, S. Li, S. Nie, W. Zhao, H. Yang, S. Sun and C. Zhao, Biomacromolecules, 2012, 13, 4236 CAS.
- C. Cheng, S. Nie, S. Li, H. Peng, H. Yang, L. Ma, S. Sun and C. Zhao, J. Mater. Chem. B, 2013, 1, 265 RSC.
- H. Liu, Y. Liu and D. Zhu, J. Mater. Chem., 2011, 21, 3335 RSC.
- A. H. Castro Neto, F. Guinea, N. M. R. Peres, K. S. Novoselov and A. K. Geim, Rev. Mod. Phys., 2009, 81, 109 CrossRef CAS.
- I. Gierz, C. Riedl, U. Starke, C. R. Ast and K. Kern, Nano Lett., 2008, 8, 4603 CrossRef CAS PubMed.
- D. Wei and Y. Liu, Adv. Mater., 2010, 22, 3225 CrossRef CAS PubMed.
- O. S. Kwon, S. J. Park, J. Y. Hong, A. R. Han, J. S. Lee, J. S. Lee, J. H. Oh and J. Jang, ACS Nano, 2012, 6, 1486 CrossRef CAS PubMed.
- Y. Wang, Y. Shao, D. W. Matson, J. Li and Y. Lin, ACS Nano, 2010, 4, 1790 CrossRef CAS PubMed.
- A. J. Samuels and J. D. Carey, ACS Nano, 2013, 7, 2790 CrossRef CAS PubMed.
- T. O. Wehling, K. S. Novoselov, S. V. Morozov, E. E. Vdovin, M. I. Katsnelson and A. K. Geim, Nano Lett., 2008, 8, 173 CrossRef CAS PubMed.
- P. Wei, N. Liu, H. R. Lee, E. Adijanto, L. Ci and B. D. Naab, Nano Lett., 2013, 13, 1890 CrossRef CAS PubMed.
- F. Yavari, C. Kritzinger, C. Gaire, L. Song, H. Gulapalli and T. Borca-Tasciuc, Small, 2010, 6, 2535 CrossRef CAS PubMed.
- F. Schedin, A. K. Geim, S. V. Morozov, E. W. Hill, P. Blake and M. I. Katsnelson, Nat. Mater., 2007, 6, 652 CrossRef CAS PubMed.
- N. Jung, N. Kim, S. Jockusch, N. J. Turro, P. Kim and L. Brus, Nano Lett., 2009, 9, 4133 CrossRef CAS PubMed.
- S. Some, J. Kim, K. Lee, A. Kulkarni, Y. Yoon and S. Lee, Adv. Mater., 2012, 24, 5481 CrossRef CAS PubMed.
- W. Chen, S. Chen, D. Qi, X. Gao and A. T. Wee, J. Am. Chem. Soc., 2007, 129, 10418 CrossRef CAS PubMed.
- Y. H. Lu, W. Chen and Y. P. Feng, J. Phys. Chem. B, 2009, 113, 2 CrossRef CAS PubMed.
- X. Dong, D. Fu, W. Fang, Y. Shi, P. Chen and L. J. Li, Small, 2009, 5, 1422 CrossRef CAS PubMed.
- D. B. Farmer, R. Golizadeh-Mojarad, V. Perebeinos, Y. M. Lin, G. S. Tulevski, J. C. Tsang and P. Avouris, Nano Lett., 2009, 9, 388 CrossRef CAS PubMed.
- P. Wei, N. Liu, H. R. Lee, E. Adijanto, L. Ci and B. D. Naab, Nano Lett., 2013, 13, 1890 CrossRef CAS PubMed.
- G. Giovannetti, P. Khomyakov, G. Brocks, V. Karpan, J. Brink and P. Kelly, Phys. Rev. Lett., 2008, 101, 026803 CrossRef CAS.
- H. Wang, T. Maiyalagan and X. Wang, ACS Catal., 2012, 2, 781 CrossRef CAS.
- Y. Zhang, L. Zhang and C. Zhou, Acc. Chem. Res., 2013, 46, 2329 CrossRef CAS PubMed.
- T. Kato and R. Hatakeyama, ACS Nano, 2012, 6, 8508 CrossRef CAS PubMed.
- Y. Xue, B. Wu, L. Jiang, Y. Guo, L. Huang and J. Chen, J. Am. Chem. Soc., 2012, 134, 11060 CrossRef CAS PubMed.
- K. Yan, L. Fu, H. Peng and Z. Liu, Acc. Chem. Res., 2013, 46, 2263 CrossRef CAS PubMed.
- X. Li, W. Cai, L. Colombo and R. S. Ruoff, Nano Lett., 2009, 9, 4268 CrossRef CAS PubMed.
- A. Reina, S. Thiele, X. Jia, S. Bhaviripudi, M. S. Dresselhaus and J. A. Schaefer, Nano Res., 2009, 2, 509 CrossRef CAS.
- B. Dai, L. Fu, Z. Zou, M. Wang, H. Xu, S. Wang and Z. Liu, Nat. Commun., 2011, 2, 522 CrossRef PubMed.
- N. Liu, L. Fu, B. Dai, K. Yan, X. Liu, R. Zhao, Y. Zhang and Z. Liu, Nano Lett., 2011, 11, 297 CrossRef CAS PubMed.
- X. Liu, L. Fu, N. Liu, T. Gao, Y. Zhang, L. Liao and Z. Liu, J. Phys. Chem. C, 2011, 115, 11976 CAS.
- Y. F. Lu, S. T. Lo, J. C. Lin, W. Zhang, J.-Y. Lu, F. H. Liu, C. M. Tseng, Y. H. Lee, C. T. Liang and L. J. Li, ACS Nano, 2013, 7, 6522 CrossRef CAS PubMed.
- H. Wang, Y. Zhou, D. Wu, L. Liao, S. Zhao, H. Peng and Z. Liu, Small, 2013, 9, 1316 CrossRef CAS PubMed.
- A. L. M. Reddy, A. Srivastava, S. R. Gowda, H. Gullapalli, M. Dubey and P. M. Ajayan, ACS Nano, 2010, 4, 6337 CrossRef CAS PubMed.
- Z. Jin, J. Yao, C. Kittrell and J. M. Tour, ACS Nano, 2011, 5, 4112 CrossRef CAS PubMed.
- P. C. Chang, Z. Y. Fan, D. W. Wang, W. Y. Tseng, W. A. Chiou, J. Hong and J. G. Lu, Chem. Mater., 2004, 16, 5133 CrossRef CAS.
- H. M. Jeong, J. W. Lee, W. H. Shin, Y. J. Choi, H. J. Shin, J. K. Kang and J. W. Choi, Nano Lett., 2011, 11, 2472 CrossRef CAS PubMed.
- X. Li, L. Fan, Z. Li, K. Wang, M. Zhong, J. Wei, D. Wu and H. Zhu, Adv. Energy Mater., 2012, 2, 425 CrossRef CAS PubMed.
- H. Liping, W. Bin, C. Jianyi, X. Yunzhou, G. Dechao, G. Yunlong, Y. Gui and L. Yunqi, Small, 2013, 9, 1330 CrossRef PubMed.
- J. Han, L. L. Zhang, S. Lee, J. Oh, K.-S. Lee, J. R. Potts, J. Ji, X. Zhao, R. S. Ruoff and S. Park, ACS Nano, 2013, 7, 19 CrossRef CAS PubMed.
- D. Deng, X. Pan, L. Yu, Y. Cui, Y. Jiang, J. Qi, W.-X. Li, Q. Fu, X. Ma, Q. Xue, G. Sun and X. Bao, Chem. Mater., 2011, 23, 1188 CrossRef CAS.
- Z. S. Wu, A. Winter, L. Chen, Y. Sun, A. Turchanin, X. Feng and K. Muellen, Adv. Mater., 2012, 24, 5130 CrossRef CAS PubMed.
- O. Volotskova, I. Levchenko, A. Shashurin, Y. Raitses, K. Ostrikov and M. Keidar, Nanoscale, 2010, 2, 2281 RSC.
- B. Shen, J. Chen, X. Yan and Q. Xue, RSC Adv., 2012, 2, 6761 RSC.
- L. S. Panchokarla, K. S. Subrahmanyam, S. K. Saha, A. Govindaraj, H. R. Krishnamurthy, U. V. Waghmare and C. N. R. Rao, Adv. Mater., 2009, 21, 4726 Search PubMed.
- L. Huang, B. Wu, J. Chen, Y. Xue, D. Geng, Y. Guo, G. Yu and Y. Liu, Small, 2013, 9, 1330 CrossRef CAS PubMed.
- S. Wang, L. Zhang, Z. Xia, A. Roy, D. W. Chang, J. B. Baek and L. Dai, Angew. Chem., Int. Ed., 2012, 51, 4209 CrossRef CAS PubMed.
- W. Ding, Z. Wei, S. Chen, X. Qi, T. Yang, J. Hu, D. Wang, L. J. Wan, S. F. Alvi and L. Li, Angew. Chem., Int. Ed., 2013, 52, 11755 CrossRef CAS PubMed.
- J. Liang, Y. Jiao, M. Jaroniec and S. Z. Qiao, Angew. Chem., Int. Ed., 2012, 51, 11496 CrossRef CAS PubMed.
- S. Yang, L. Zhi, K. Tang, X. Feng, J. Maier and K. Muellen, Adv. Funct. Mater., 2012, 22, 3634 CrossRef CAS PubMed.
- Q. D. Chen, L. M. Dai, M. Gao, S. M. Huang and A. Mau, J. Phys. Chem. B, 2001, 105, 618 CrossRef CAS.
- H. M. Jeong, J. W. Lee, W. H. Shin, Y. J. Choi, H. J. Shin, J. K. Kang and J. W. Choi, Nano Lett., 2011, 11, 2472 CrossRef CAS PubMed.
- T. Kato, L. Jiao, X. Wang, H. Wang, X. Li, L. Zhang, R. Hatakeyama and H. Dai, Small, 2011, 7, 574 CrossRef CAS PubMed.
- Z. Fang, Y. Wang, Z. Liu, A. Schlather, P. M. Ajayan, F. H. L. Koppens, P. Nordlander and N. J. Halas, ACS Nano, 2012, 6, 10222 CrossRef CAS PubMed.
- J. Nicolle, D. Machon, P. Poncharal, O. Pierre-Louis and A. San-Miguel, Nano Lett., 2011, 11, 3564 CrossRef CAS PubMed.
- M. Kim, N. S. Safron, C. Huang, M. S. Arnold and P. Gopalan, Nano Lett., 2012, 12, 182 CrossRef CAS PubMed.
- C. K. Chang, S. Kataria, C. C. Kuo, A. Ganguly, B. Y. Wang, J. Y. Hwang, K. J. Huang, W. H. Yang, S. B. Wang, C. H. Chuang, M. Chen, C. I. Huang, W. F. Pong, K. J. Song, S. J. Chang, J. H. Guo, Y. Tai, M. Tsujimoto, S. Isoda, C. W. Chen, L. C. Chen and K. H. Chen, ACS Nano, 2013, 7, 1333 CrossRef CAS PubMed.
- K. S. Novoselov, A. K. Geim, S. V. Morozov, D. Jiang, M. I. Katsnelson, I. V. Grigorieva, S. V. Dubonos and A. A. Firsov, Nature, 2005, 438, 197 CrossRef CAS PubMed.
- R. Cheng, J. Bai, L. Liao, H. Zhou, Y. Chen, L. Liu, Y.-C. Lin, S. Jiang, Y. Huang and X. Duan, Proc. Natl. Acad. Sci. U. S. A., 2012, 109, 11588 CrossRef CAS PubMed.
- Y. Wu, K. A. Jenkins, A. Valdes-Garcia, D. B. Farmer, Y. Zhu, A. A. Bol, C. Dimitrakopoulos, W. Zhu, F. Xia, P. Avouris and Y. M. Lin, Nano Lett., 2012, 12, 3062 CrossRef CAS PubMed.
- F. Bonaccorso, Z. Sun, T. Hasan and A. C. Ferrari, Nat. Photonics, 2010, 4, 611 CrossRef CAS PubMed.
- D. W. Chang, E. K. Lee, E. Y. Park, H. Yu, H. J. Choi, I. Y. Jeon, G. J. Sohn, D. Shin, N. Park, J. H. Oh, L. Dai and J. B. Baek, J. Am. Chem. Soc., 2013, 135, 8981 CrossRef CAS PubMed.
- S. Some, J. Kim, K. Lee, A. Kulkarni, Y. Yoon, S. Lee, T. Kim and H. Lee, Adv. Mater., 2012, 24, 5481 CrossRef CAS PubMed.
- G. Giovannetti, P. A. Khomyakov, G. Brocks, V. M. Karpan, J. van den Brink and P. J. Kelly, Phys. Rev. Lett., 2008, 101, 026803 CrossRef CAS.
- P. A. Khomyakov, A. A. Starikov, G. Brocks and P. J. Kelly, Phys. Rev. B: Condens. Matter Mater. Phys., 2010, 82, 115437 CrossRef.
- J. B. Bult, R. Crisp, C. L. Perkins and J. L. Blackburn, ACS Nano, 2013, 7, 7251 CrossRef CAS PubMed.
- Y. Zhang, J. Ge, L. Wang, D. Wang, F. Ding, X. Tao and W. Chen, Sci. Rep., 2013, 3, 2771 Search PubMed.
- L. S. Panchakarla, K. S. Subrahmanyam, S. K. Saha, A. Govindaraj, H. R. Krishnamurthy, U. V. Waghmare and C. N. R. Rao, Adv. Mater., 2009, 21, 4726 CAS.
- K. C. Kwon, K. S. Choi and S. Y. Kim, Adv. Funct. Mater., 2012, 22, 4724 CrossRef CAS PubMed.
- M. Li, Z. Wu, W. Ren, H. Cheng, N. Tang, W. Wu, W. Zhong and Y. Du, Carbon, 2012, 50, 5286 CrossRef CAS PubMed.
- Y. Li, Y. Zhao, H. Cheng, Y. Hu, G. Shi, L. Dai and L. Qu, J. Am. Chem. Soc., 2012, 134, 15 CrossRef CAS PubMed.
- Q. Liu, B. Guo, Z. Rao, B. Zhang and J. R. Gong, Nano Lett., 2013, 13, 2436 CrossRef CAS PubMed.
- R. Lv, Q. Li, A. R. Botello-Mendez, T. Hayashi, B. Wang, A. Berkdemir, Q. Hao, A. L. Elias, R. Cruz-Silva, H. R. Gutierrez, Y. A. Kim, H. Muramatsu, J. Zhu, M. Endo, H. Terrones, J. C. Charlier, M. Pan and M. Terrones, Sci. Rep., 2012, 2, 586 Search PubMed.
- D. Geng, S. Yang, Y. Zhang, J. Yang, J. Liu, R. Li, T. K. Sham, X. Sun, S. Ye and S. Knights, Appl. Surf. Sci., 2011, 257, 9193 CrossRef CAS PubMed.
- P. Kannan, T. Maiyalagan, N. G. Sahoo and M. Opallo, J. Mater. Chem. B, 2013, 1, 4655 RSC.
- D. Usachov, O. Vilkov, A. Grueneis, D. Haberer, A. Fedorov, V. K. Adamchuk, A. B. Preobrajenski, P. Dudin, A. Barinov, M. Oehzelt, C. Laubschat and D. V. Vyalikh, Nano Lett., 2011, 11, 5401 CrossRef CAS PubMed.
- J. Wang, Electroanalysis, 2005, 17, 7 CrossRef CAS PubMed.
- M. Zhou, Y. M. Zhai and S. J. Dong, Anal. Chem., 2009, 81, 5603 CrossRef CAS PubMed.
- Y. Shao, S. Zhang, M. H. Engelhard, G. Li, G. Shao, Y. Wang, J. Liu, I. A. Aksay and Y. Lin, J. Mater. Chem., 2010, 7491 RSC.
- J. Guo, T. Zhang, C. Hu and L. Fu, Nanoscale, 2015, 7, 1290 RSC.
- Z. H. Sheng, X. Q. Zheng, J. Y. Xu, W. J. Bao, F. B. Wang and X. H. Xia, Biosens. Bioelectron., 2012, 34, 125 CrossRef CAS PubMed.
- S. M. Tan, H. L. Poh, Z. Sofer and M. Pumera, Analyst, 2013, 138, 4885 RSC.
- L. Tang, Y. Wang, Y. Li, H. Feng, J. Lu and J. Li, Adv. Funct. Mater., 2009, 19, 2782 CrossRef CAS PubMed.
- P. Wang, Z. Mai, Z. Dai, Y. Li and X. Zou, Biosens. Bioelectron., 2009, 24, 3242 CrossRef CAS PubMed.
- S. S. Mirvish, Cancer Lett., 1995, 93, 17 CrossRef CAS.
- X. R. Li, F. Y. Kong, J. Liu, T. M. Liang, J. J. Xu and H. Y. Chen, Adv. Funct. Mater., 2012, 22, 1981 CrossRef CAS PubMed.
- X. Dong, Y. Shi, W. Huang, P. Chen and L. J. Li, Adv. Mater., 2010, 22, 1649 CrossRef CAS PubMed.
- B. Gulbakan, E. Yasun, M. I. Shukoor, Z. Zhu, M. You, X. Tan, H. Sanchez, D. H. Powell, H. Dai and W. Tan, J. Am. Chem. Soc., 2010, 132, 17408 CrossRef CAS PubMed.
- F. Chen, Q. Qing, J. Xia, J. Li and N. Tao, J. Am. Chem. Soc., 2009, 131, 9908 CrossRef CAS PubMed.
- I. Willner and M. Zayats, Angew. Chem., Int. Ed., 2007, 46, 6408 CrossRef CAS PubMed.
- R. Stine, J. T. Robinson, P. E. Sheehan and C. R. Tamanaha, Adv. Mater., 2010, 22, 5297 CrossRef CAS PubMed.
- J. Wang, W. W. Zhao, X.-R. Li, J. J. Xu and H. Y. Chen, Chem. Commun., 2012, 48, 6429 RSC.
- G. Yang, L. Li, R. K. Rana and J. J. Zhu, Carbon, 2013, 61, 357 CrossRef CAS PubMed.
- L. Zhao, S. Li, J. He, G. Tian, Q. Wei and H. Li, Biosens. Bioelectron., 2013, 49, 222 CrossRef CAS PubMed.
- R. Feng, Y. Zhang, H. Ma, D. Wu, H. Fan, H. Wang, H. Li, B. Du and Q. Wei, Electrochim. Acta, 2013, 97, 105 CrossRef CAS PubMed.
- S. Deng, J. Lei, Y. Huang, Y. Cheng and H. Ju, Anal. Chem., 2013, 85, 5390 CrossRef CAS PubMed.
- Z. Qian, X. Shan, L. Chai, J. Ma, J. Chen and H. Feng, ACS Appl. Mater. Interfaces, 2014, 6, 6797 CAS.
- W. Li, Z. Zhang, B. Kong, S. Feng, J. Wang, L. Wang, J. Yang, F. Zhang, P. Wu and D. Zhao, Angew. Chem., Int. Ed., 2013, 52, 8151 CrossRef CAS PubMed.
- Y. Zheng, Y. Jiao, L. H. Li, T. Xing, Y. Chen, M. Jaroniec and S. Z. Qiao, ACS Nano, 2014, 8, 5290 CrossRef CAS PubMed.
- Y. Ito, W. Cong, T. Fujita, Z. Tang and M. Chen, Angew. Chem., Int. Ed., 2015, 54, 2131 CrossRef CAS PubMed.
- D. Jiang, X. Du, Q. Liu, N. Hao, J. Qian, L. Dai, H. Mao and K. Wang, Chem. Commun., 2015, 51, 4451 RSC.
- M. Rein, N. Richter, K. Parvez, X. Feng, H. Sachdev, M. Kläui and K. Müllen, ACS Nano, 2015, 9, 1360 CrossRef CAS PubMed.
- S. Li, D. Wu, C. Cheng, J. Wang, F. Zhang, Y. Su and X. Feng, Angew. Chem., Int. Ed., 2013, 125, 12327 CrossRef PubMed.
- W. He, C. Jiang, J. Wang and L. Lu, Angew. Chem., Int. Ed., 2014, 53, 9503 CrossRef CAS PubMed.
- S. Li, D. Wu, H. Liang, J. Wang, X. Zhuang, Y. Mai, Y. Su and X. Feng, ChemSusChem, 2014, 7, 3002 CrossRef CAS PubMed.
- E. Haque, M. M. Islam, E. Pourazadi, M. Hassan, S. N. Faisal, A. K. Roy, K. Konstantinov, A. T. Harris, A. I. Minettc and V. G. Gomes, RSC Adv., 2015, 5, 30679 RSC.
- Y. Tian, Y. Liu, J. Zhao and Y. Ding, RSC Adv., 2015, 5, 34070 RSC.
|
This journal is © The Royal Society of Chemistry 2015 |