DOI:
10.1039/C5RA04423E
(Paper)
RSC Adv., 2015,
5, 32168-32174
Selective and sensitive SERS sensor for detection of Hg2+ in environmental water base on rhodamine-bonded and amino group functionalized SiO2-coated Au–Ag core–shell nanorods†
Received
13th March 2015
, Accepted 30th March 2015
First published on 30th March 2015
Abstract
Here, we designed a new type of SERS sensor with high selectivity and sensitivity for the determination of Hg2+ at picomolar concentrations based on hydrolysis reactions. The Rhodamine 6G-derived Schiff base bonded and amino group functionalized SiO2-coated Au–Ag core–shell nanorods (Au@Ag@SiO2–NH2-R6G, NR-Rs), which show extraordinary enhancement factors (EF) and good stability, were employed as the SERS substrate of the sensor. The tunable ability of the chemical bonding of the substrate generates appropriate probe molecules bound to the surface of the nanorods (NRs) and improves the selectivity and sensitivity of the SERS sensor dramatically. The limit of detection (LOD) of Hg2+ obtained using the present SERS sensor was 0.33 pmol L−1. Furthermore, the proposed substrate has pH-responsive ability. The present sensor is suitable for the on-site determination of pH and Hg2+ by combining with a portable Raman spectrometer.
1. Introduction
The technique of surface-enhanced Raman spectroscopy (SERS) is currently undergoing a very dynamic development and many novel directions are rapidly emerging.1,2 In recent years, SERS has opened up new and exciting horizons in analytical chemistry and molecular biology, such as sensors for detecting organic pollutants and single-molecule detection of proteins.3–5 Now it is widely accepted that there are two main effects of enhancement mechanisms of SERS: the electromagnetic effect (EM) and the chemical effect (CM). The localized surface plasmon resonance (LSPR) plays a key and dominant role for the EM in the overall enhancement; whereas the CM, which is due to the interaction of the molecules adsorbed on the metal with the metal surfaces, is mostly from the first layer of the charge-transfer resonance between the adsorbate and the metal.6–8
Over the past 30 years, a large variety of highly SERS-active substrates have been developed, such as metal nanoparticles,9–11 roughened electrodes,12 metal island films13–15 and so on. Metal nanoparticles, particularly Au and Ag nanoparticles are frequently used as SERS substrates because of the simplicity of their preparations and characterizations.16–18 Previous research found that bimetallic Au and Ag nanoparticles could form favorable states of aggregation and increase the SERS activity more significantly than mono- Au or Ag nanoparticles.19–21 Metal nanoparticles with various shapes, including cubes, prisms, rods and octahedra, have been synthesized as SERS substrates.22 Experimental and theoretical results indicate that anisotropic nanoparticles have stronger enhancement effects than spherical nanoparticles because the sharp edges and angles of metal nanoparticles will give extra enhancements owing to the “lightning rod effect”.23 In order to improve the selectivity and stability of SERS-active substrates, Tian et al.24 developed a new technique called shell-isolated nanoparticle-enhanced Raman spectroscopy (SHINERS), by employing a kind of metal nanoparticles with insulating ultrathin thin SiO2 shells possessing pinholes on their surface as SERS substrates. The thin coating keeps nanoparticles from agglomerating, and protects the SERS-active nanostructure from contacting with whatever is being analyzed. Compared with routine metal nanoparticles, metal@SiO2 nanoparticles have higher sensitivity, better stability and reproducibility.25,26
Mercury ions (Hg2+) can lead to damages of brain, kidneys, the central nervous system, the immune system and the endocrine system of human body even at a very low concentration.27 Soluble Hg2+ in drinking waters are the main source of mercury absorbed into human body and attract great concern.28 U. S. Environmental Protection Agency (EPA) recommends that the levels of Hg2+ in drinking water should be reduced to 2 μg L−1 (10 nmol L−1).29 Thus, there is an urgent need of accurate and ultrasensitive detection methods for Hg2+.
Nowadays, several Hg2+ detection methods based on inductively coupled plasma-mass spectrometry (ICP-MS),30 fluorescence quenching,31 surface plasmon resonance (SPR) sensor32 and SERS33 have been developed. SERS, in particular, is a promising method for ultra-highly sensitive detection of Hg2+ because it offers practical facilities for on-site detection by using miniaturized Raman instruments.34 Unlike organic or biological molecules, Hg2+ does not exhibit any Raman signals, thus the only way to determine it using SERS probe is the indirect signal readout. Recently, several SERS probe for the determination of Hg2+, such as cysteine,35 tryptophan,36 4,4′-dipyridyl,37 and DNA38 were proposed by many research groups. In these researches, nanoparticles and probes were usually self-assembled by physical absorption.
In this paper, we try to use Rhodamine 6G (R6G)-derived Schiff base bonded and amino group functionalized SiO2-coated Au–Ag core–shell nanorods (NR-Rs) as the SERS substrate. It has been reported that R6G and its derivatives can be used as fluorescence39 and SERS40,41 probes. However, SERS sensors are more selective than fluorescence sensors in general due to the abundant information of molecular structures in Raman spectra. R6G possesses functional groups of chemical affinity, and exhibits strong Raman signals as SERS probe.42 The tunable ability of chemical bonding of the substrate will make appropriate probe molecules bound to the surface of nanorods and improve selectivity and sensitivity of the SERS sensor dramatically.43 The proposed substrate was applied to the determination of pH and Hg2+ by combining with a portable Raman spectrometer.
2. Experimental details
2.1 Chemicals and reagents
Rhodamine 6G (R6G, 98.5%), hydrogen tetrachloroaurate(III) trihydrate (HAuCl4·3H2O, 49.0% Au) and silver nitrate (AgNO3, 99%) were purchased from J&K Chemical Ltd. 3-Aminopropyltriethoxysilane (APTES, 99%) and sodium silicate solution (Na2SiO3, 27% SiO2) were purchased from Sigma-Aldrich Ltd. Hydrazine monohydrate (N2H4·H2O, 85%), oxalaldehyde hydrate (40%), sodium borohydride (NaBH4, 96%), hexadecyl trimethyl ammonium bromide (CTAB, 98%), ascorbic acid (AA, 99%), sulfuric acid (H2SO4, 98%), nitric acid (HNO3, 65%), hydrochloric acid (HCl, 38%), phosphorous acid (H3PO4, 85%), sodium hydroxide (NaOH, 98%), ammonia solution (25%), Hg(NO3)2, CuSO4·5H2O, Cr(NO3)3·9H2O, Pb(CH3COO)2·3H2O, FeCl3·6H2O, MgCl2·6H2O, NiCl2·6H2O, CoCl2·6H2O, ZnCl2, CdSO4·8/3H2O and BaCl2·2H2O were purchased from Sinopharm Chemical Reagent Co., Ltd., China. All of these reagents were used without further purification. All the solvents were of analytical grade. All aqueous solutions were prepared with ultra-pure water (18.2 MΩ cm) from a Milli-Q water purification system.
2.2 Instruments
Raman spectra were obtained using a portable miniature Raman spectrometer (Changchun Jilin University Little Swan Instruments Co., Ltd., Changchun, China) with 1 cm quartz cells and a 785 nm diode laser. The laser power was chosen as 300 mW. The surface morphologies of the NRs were measured on a Hitachi H-800 transmission electron microscope (TEM, Hitachi Ltd., Japan) operating at 175 kV and a JEM-2100F high resolution transmission electron microscope (HR-TEM, JEOL Ltd., Japan) operating at 200 kV. The samples for TEM images were prepared by dropping the solutions containing NRs on the carbon-coated copper grid and drying at room temperature. Absorption spectra of the NRs colloids were recorded on a TU-1810C UV-Vis spectrometer (Beijing Purkinje General Inc., China) within the wavelength range from 300 to 1000 nm. Spectra of 1H-NMR were measured on a Mercury 300BB nuclear magnetic resonance spectrometer (Varian Inc., USA) with chemical shifts reported as ppm (in d6-DMSO, TMS as internal standard). ESI-MS spectra were measured on a LC/MS QTRAP spectrometer (AB SCIEX Inc., USA). High resolution ESI-TOF-MS (HR-ESI-TOF-MS) spectra were measured on a HPLC-ESI-TOF-MS spectrometer (AB SCIEX Inc., USA). FT-IR spectra were recorded using KBr pellets on a Nicolet Avatar 360 FT-IR spectrophotometer (Thermo Fisher Scientific Inc., USA) in the wavenumber region of 4000–400 cm−1. Fluorescent spectra were collected with a RF-5301 PC fluorospectrophotometer (SHIMADZU CO., LTD., Japan). All pH measurements were made with a PHS-3C pH-Meter (INESA Scientific Inc., China).
2.3 Synthesis of SERS substrate NR-Rs
The synthetic route of NR-Rs is shown in Fig. 1.
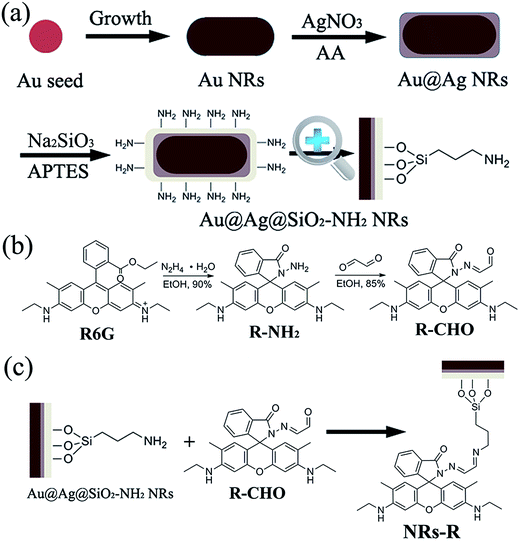 |
| Fig. 1 (a) Schematic illustration of the formation process of Au@Ag@SiO2–NH2 NRs. (b) Synthetic route of compound R–CHO. (c) Synthetic route of SERS substrate NR-Rs. | |
2.3.1 Synthesis of Au@Ag@SiO2–NH2 NRs. Au NRs were synthesized by using a seed mediated growth procedure.44 CTAB-capped Au seeds were synthesized by the chemical reduction of HAuCl4 with NaBH4. 7.5 mL of 0.1 mol L−1 CTAB was mixed with 100 μL of 24 mmol L−1 HAuCl4. The mixture was diluted with ultrapure water to 9.4 mL, and was stirred with a magnetic stirrer in ice-bath. Then, 600 μL of 10 mmol L−1 NaBH4 was added. The Au seeds were obtained. The growth solution for the formation of the Au NRs consisted of 100 mL of 0.1 mol L−1 CTAB, 2.04 mL of 24 mmol L−1 HAuCl4, 2.0 mL of 0.5 mol L−1 H2SO4, 1.0 mL of 10 mmol L−1 AgNO3 and 800 μL of 0.1 mol L−1 AA. 240 μL of Au seed solution was added to the growth solution to initiate the growth of the Au NRs. After 12 h, the growth was stopped. The obtained Au NRs were purified by centrifuging the result solution at 13
000 rpm for 10 min, twice. The nanorods were collected and redispersed in ultrapure water with the volume of the original solution before centrifuging.20 mL of the above Au NRs solution and 3.0 mL of 0.1 mol L−1 AA were mixed in a 100 mL round flask under magnetic stirring. Then 7.0 mL of 1 mmol L−1 AgNO3 was added into the mixture in drop wise manner at a rate of 50 μL per 30 s. AgNO3 was reduced by AA and the resultant Ag continuously grew on the surface of Au NRs. After its color changed from purplish red to brown, the solution was continuously stirred for 30 min. The obtained Au@Ag NRs were purified by centrifuging the result solution at 13
000 rpm for 10 min, twice. The result nanorods were also collected and redispersed in ultrapure water with the volume of the original solution before centrifuging.
Amino group functionalized SiO2-coated Au@Ag NRs (Au@Ag@SiO2–NH2 NRs) were prepared according to the reported method, with some modification.45 A freshly prepared aqueous solution of 0.4 mL of 1 mmol L−1 APTES was added to 30 mL Au@Ag NRs solution under vigorous stirring in 15 min for the complexation of amine groups with the silver surfaces of Au@Ag NRs. Then 3.2 mL of 0.54% (w/w) Na2SiO3 was added to the solution under vigorous stirring. The mixture was stirred for 20 min in a water bath at 90 °C. The obtained Au@Ag@SiO2 NRs were purified by centrifuging the result solution at 13
000 rpm for 10 min and dispersed in 30 mL ethanol containing trace amounts of water. Then 1 mL of 0.1 mmol L−1 APTES was added to the dispersion and the mixture was heated to 50 °C for 8 h to modify the silica surfaces of NRs. The Au@Ag@SiO2–NH2 NRs were isolated from the mixture by centrifuging at 13
000 rpm for 10 min and washed with ethanol and water for 3 times. Finally, the Au@Ag@SiO2–NH2 NRs were dispersed in 30 mL ethanol and stored at 4 °C.
2.3.2 Synthesis of compound R–CHO. R–NH2 was synthesized from R6G according to the literature method.46 0.958 g R6G was dissolved in 30 mL absolute ethanol. 3.0 mL (excess) N2H4·H2O was added, and the mixture was heated to reflux in an oil bath for 2 h with stirring. The precipitate produced was filtered and washed with 15 mL EtOH/H2O (1
:
1) for 3 times, and dried over P2O5 under vacuum. The reaction yielded 0.77 g R–NH2 as light pink solid (yield 90%). ESI-MS: m/z 429.4 for [M + H]+. 1H-NMR (d6-DMSO, 300 MHz), δ (ppm): 7.80–7.71 (m, 1H), 7.51–7.41 (m, 2H), 6.98–6.88 (m, 1H), 6.27 (s, 2H), 6.10 (d, J = 0.7 Hz, 2H), 5.00 (t, J = 5.4 Hz, 2H), 4.22 (s, 2H), 3.24–3.03 (m, 4H), 1.87 (s, 6H), 1.21 (t, J = 7.1 Hz, 6H).R–CHO was synthesized according to the reported method, with some modification.47 0.30 g R–NH2 was dissolved in 15 mL of boiled absolute ethanol. Then 3.0 mL (excess) oxalaldehyde hydrate was added, and the mixture was heated to reflux in an oil bath under N2 atmosphere for 4 h with stirring. The precipitate produced was filtered and washed with 15 mL cold ethanol for 3 times, and dried over P2O5 under vacuum. The reaction yielded 0.28 g R–CHO as yellow solid (yield 85%). ESI-MS: m/z 469.3 for [M + H]+. 1H-NMR (d6-DMSO, 300 MHz), δ (ppm): 9.25 (d, J = 7.5 Hz, 1H), 8.00 (d, J = 7.0 Hz, 1H), 7.63 (dtd, J = 22.0, 7.4, 1.1 Hz, 2H), 7.26 (d, J = 7.5 Hz, 1H), 7.04 (d, J = 7.4 Hz, 1H), 6.31 (s, 2H), 6.18 (d, J = 0.5 Hz, 2H), 5.18 (t, J = 5.4 Hz, 2H), 3.14 (dd, J = 6.9, 5.6 Hz, 4H), 1.85 (s, 6H), 1.20 (t, J = 7.1 Hz, 6H).
2.3.3 Synthesis of NR-Rs. NR-Rs were synthesized by a direct chemical bonding reaction, which is also a Schiff base condensation reaction between R–CHO and Au@Ag@SiO2–NH2 NRs. 20 mL of Au@Ag@SiO2–NH2 NRs ethanol solution was mixed with 20 mL of 1.0 × 10−5 mol L−1 R–CHO ethanol solution, and the mixture was stirred thoroughly and protected from light for 24 h at room temperature. The obtained NR-Rs were purified to remove unreacted R–CHO by centrifuging at 13
000 rpm for 10 min and washed with copious ethanol and water sequentially for 3 times. Finally, the NR-Rs were dispersed in 20 mL ultrapure water and stored at 4 °C.
2.4 SERS measurements
In a typical process, 100 μL of NR-Rs solution, 10 μL of analyte solution and 50 μL of phosphate buffer were added into a quartz cell for SERS analysis. The typical exposure time for each measurement was 10 s with 3 accumulations. Five measurements of three samples were used to calculate the relative standard deviations (RSDs). Three environmental water samples, including lake water, reservoir water and river water, were collected from local sources of drinking water. These samples and their spiked controls were directly analyzed by SERS without any preparation procedures.
3. Results and discussion
3.1 Characterization of Au@Ag@SiO2–NH2 NRs
Fig. 1a shows the preparation process of Au@Ag@SiO2–NH2 NRs. The Au NRs prepared by using a seed mediated growth procedure44 exhibit two main absorption bands: one is the transverse surface plasmon resonance (SPR) band around 520 nm, similar to the absorption band of spherical Au nanoparticles; the other is the longitudinal localized surface plasmon resonance (longitudinal LSPR) band, which is tunable from the visible through near-infrared region with the changing of nanorod aspect ratio.48 The as-prepared Au NRs with typical lengths of 45 nm and aspect ratios about 3.0 show a strong longitudinal LSPR band around 674 nm, and a weak transverse SPR band around 516 nm (Fig. 2a and e). The Au@Ag NRs were prepared by chemical reduction method. AgNO3 was reduced by ascorbic acid (AA) and the resultant Ag continuously grew on the surfaces of Au NRs. The average aspect ratios, length and thickness of silver shells of the Au@Ag NRs are 2.5, 55 nm and 5 nm, respectively (Fig. 2b and e). Compared with Au NRs, Au@Ag NRs produce a new LSPR band of Ag shells at 390 nm, and a LSPR band shifted to 596 nm. In order to obtain selective and stable SERS substrates, amino group functionalized silica shells with a thickness of 4 nm were coated on the surface of Au@Ag NRs (Fig. 2c and d). The silica shells, which have little effects on the assignments of LSPR bands of Au@Ag NRs (Fig. 2e), can reduce the agglomeration of nanoparticles. The FT-IR absorption spectrum of the Au@Ag@SiO2–NH2 NRs is shown in Fig. S1 (see ESI†). The characteristic absorption bands in the range from 3500 cm−1 to 3200 cm−1 were attributed to asymmetrical stretching vibrations of amino groups. The band at 1056 cm−1 was attributed to stretching vibrations of Si–O–Si structures.49 These absorption bands indicated that silica shells functionalized with amino groups were successfully coated on the surface of Au@Ag NRs. The Au@Ag@SiO2–NH2 NRs could remain stable for at least 20 day at room temperature. In contrast, obvious agglomeration and adherent phenomenon were observed for Au NRs and Au@Ag NRs in 5 days.
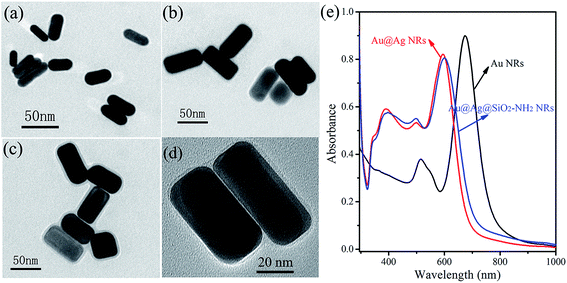 |
| Fig. 2 TEM images of Au NRs (a), Au@Ag NRs (b), and Au@Ag@SiO2–NH2 NRs (c); HR-TEM image of Au@Ag@SiO2–NH2 NRs (d); UV-Vis absorption spectra of Au NRs, Au@Ag NRs, Au@Ag@SiO2–NH2 NRs (e). | |
3.2 Calculation of SERS enhancement factor
The SERS enhancement factor (EF) is an important parameter for the performance demonstration of SERS substrates. The strong Raman peaks at 1512 cm−1 of 1.0 mmol L−1 and 1.0 nmol L−1 R6G solution were chosen as the representation peaks for EF calculation. Herein, the SERS EF is calculated from the standard equation defined as: |
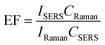 | (1) |
where ISERS and IRaman correspond to the intensities of diagnostic bands in SERS and unenhanced Raman spectra of R6G, respectively; CSERS and CRaman are, respectively, the concentrations of R6G solutions for SERS and unenhanced Raman analyses. Other experimental conditions, such as the exposure time and the power of laser, are identical in all cases. The results are shown in Fig. 3. Using Au@Ag@SiO2–NH2 NRs as SERS substrate, the Raman peak area at 1512 cm−1 of 1 nmol L−1 R6G obtained increase 2.0 and 12.9 times of the peak areas obtained using SERS substrate of Au NRs and Au@Ag NRs, respectively. The SERS activity of Au@Ag@SiO2–NH2 NRs was improved because the pinhole of silica shells make SERS-active nanostructure contact sensitively with target analytes.50
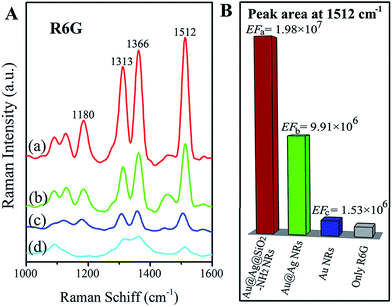 |
| Fig. 3 (A) SERS spectra of 1 nmol L−1 R6G using Au@Ag@SiO2–NH2 NRs (a), Au@Ag NRs (b), Au NRs (c) as SERS substrates, and unenchanced Raman spectrum of 1 mmol L−1 R6G (d); (B) Raman peak areas and EFs at 1512 cm−1. | |
3.3 Effect of H+ on SERS intensity and calculation of acidity constants
Fig. 4 shows the responses of the present SERS sensor at different pH values in phosphate buffer containing a few chlorine ions (50 mmol L−1). With the increase of pH values, the intensity of Raman band at 1512 cm−1 was observed to decrease distinctly, especially in the pH range from 2.0 to 7.0. It is well known that R6G-derivative exists isomers with different chemical structures and spectroscopic properties, such as spirocyclic and open-cycle forms.51,52 In an alkaline environment, most of the –R groups of NR-Rs exist in spirocyclic form, which make less –R groups fall to the “hot spot” of the NRs and the substrates exhibit weak SERS enhancement. On the basis of the surface selection rule, when molecules are perpendicular on the substrate, the SERS signal is stronger.53 Therefore, more H+ will make more spirocycles open, and will enable protonated –R groups to move closer to the surfaces of NRs, which will enhance the SERS signals. When the concentration of H+ makes the protonation tend saturated, the maximum SERS intensity of the present sensor is obtained typically.
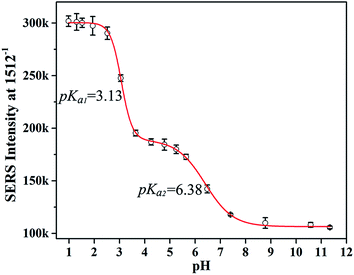 |
| Fig. 4 SERS intensity at 1512 cm−1 at different pH values. | |
The acidity constants Ka of NR-Rs were determined by SERS titration (Fig. 4). Sigmoidal curve fitting of Henderson–Hasselbach-type mass action eqn (2) to the SERS intensity I recorded as a function of pH yielded the values of pKa,
|
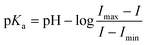 | (2) |
where
I is the observed SERS intensity at a fixed Raman shift (1512 cm
−1),
Imax and
Imin are the corresponding maximum and minimum respectively. A p
Ka1 of 3.13 and a p
Ka2 of 6.38 were yielded, which indicated that the present SERS sensor is valuable for pH sensing in acidic and near neutral media.
3.4 Effect of metal ions on SERS intensity
Thirteen metal ions including Na+, K+, Mg2+, Cu2+, Co2+, Ni2+, Cr3+, Cd2+, Fe3+, Pb2+, Ba2+, Zn2+ and Hg2+ at the concentration of 1 μmol L−1 were employed to evaluate the coordinative properties of the NR-Rs by competition experiments in phosphate buffer (pH = 1.94). As seen in Fig. 5, no significant spectral changes occurred in the presence of all the metal ions except Hg2+. The presence of Hg2+ resulted in a remarkable decrease of SERS intensity. To explore the mechanism of the decrease, the mixing solution of NR-Rs and Hg2+ was centrifuged and the supernatant was analyzed by fluorescent spectrometry and HR-ESI-TOF-MS spectrometry. An obvious fluorescence emission at 552 nm (excitation was at 520 nm) and a peak at m/z 415.2007 corresponding to [R6G acid + H]+ were observed (see ESI Fig. S2 and S3†), which indicated that in the presence of Hg2+, the complex of NR-R hydrolyzed to the R6G acid, a strong fluorescent substance that could be dissolved in the supernatant (Fig. 6). Certainly, the SERS activity of the NR-Rs would be decreased due to the detachments of –R groups occurred by Hg2+, which could facilitate the determination of Hg2+.
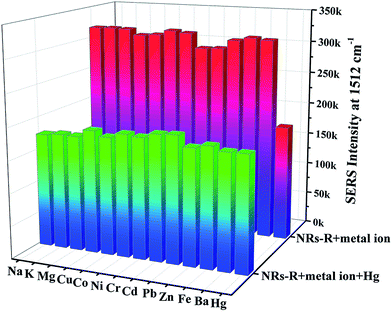 |
| Fig. 5 SRES intensity with different metal ions at 1512 cm−1 (pH = 1.94). | |
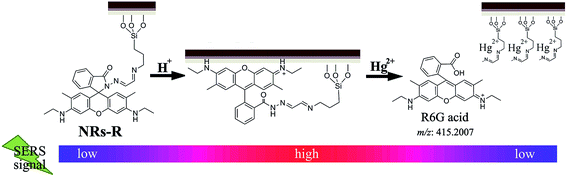 |
| Fig. 6 Schematic illustration for determining Hg2+ using NR-Rs. | |
3.5 Determination of Hg2+
100 μL of NR-Rs, 10 μL of Hg2+ solution and 50 μL of phosphate buffer (pH = 1.94) were selected as the experimental conditions for quantitative assay of Hg2+. Linear relationship between concentrations of Hg2+ and SERS intensities was attained in the concentration range of Hg2+ from 1 pmol−1 to 0.1 μmol L−1. The regression equation of calibration curve is I/I0 = −0.051
lg
C + 0.67 (C: μmol L−1), where I and I0 are the SERS peak areas at 1512 cm−1 of NR-Rs in the presence and absence of Hg2+, respectively. The correlation coefficient and the LOD were 0.9739 and 0.33 pmol L−1, respectively. These results showed that NR-Rs could be used as an ultrasensitive and selective SERS substrate for the Hg2+ sensor. The present sensor was applied to the determination of Hg2+ in environmental water samples. The obtained results were consistent with those obtained using atomic absorption spectrometry (AAS) (Table 1). Compared with AAS, the present SERS sensor for Hg2+ detection in aqueous samples has the advantages of easy and simple manipulation, as well as the ability of rapid on-site analysis with portable Raman spectrometers.
Table 1 Detection and recovery test of Hg2+ in water samples (n = 3)
Sample |
AAS method (pmol L−1) |
SERS method (pmol L−1) |
Spiked Hg2+ (pmol L−1) |
Measured Hg2+ (pmol L−1) |
Recovery (%) |
Lake water |
59.80 |
60.17 |
10.00 |
71.35 |
101.68 |
Reservoir water |
47.38 |
50.05 |
10.00 |
62.45 |
104.00 |
River water |
65.15 |
68.34 |
10.00 |
79.58 |
101.58 |
4. Conclusions
In summary, we prepared a new type of SERS sensor based on the substrates of Rhodamine-bonded and amino group functionalized SiO2-coated Au–Ag core–shell nanorods (NR-Rs), which exhibit ultra-sensitivity for Hg2+ detection and pH-responsive ability. The characteristic hydrolysis process of the complex of NR-R was identified and employed for the determination of Hg2+ in environmental water at picomolar concentrations. The consistent results obtained by the present SERS sensor and AAS, and the stability of the SERS substrates of the present sensor, show the potentials of the practical application of the present sensor in the rapid and on-site analysis.
Acknowledgements
This work was supported by National “Twelfth Five-Year” Plan for Science and Technology Support (no. 2012BAF14B08), National Natural Science Foundation of China (nos 21405057, 21207047, 21075049 and 21105037), Science and Technology Developing Foundation of Jilin Province (nos 20121808 and 20125006), and Scientific Forefront and Interdisciplinary Innovation Project of Jilin University (no. 450060481018).
References
- K. Kneipp, H. Kneipp, I. Itzkan, R. R. Dasari and M. S. Feld, Chem. Rev., 1999, 99, 2957–2976 CrossRef CAS PubMed.
- X. Niu, H. Chen, Y. Wang, W. Wang, X. Sun and L. Chen, ACS Appl. Mater. Interfaces, 2014, 6, 5152–5160 CAS.
- D. W. Li, W. L. Zhai, Y. T. Li and Y. T. Long, Microchim. Acta, 2014, 181, 23–43 CrossRef CAS.
- S. L. Kleinman, E. Ringe, N. Valley, K. L. Wustholz, E. Phillips, K. A. Scheidt, G. C. Schatz and R. P. Van Duyne, J. Am. Chem. Soc., 2011, 133, 4115–4122 CrossRef CAS PubMed.
- Y. Wang, B. Yan and L. Chen, Chem. Rev., 2013, 113, 1391–1428 CrossRef CAS PubMed.
- H. Ueba, Surf. Sci., 1983, 131, 347–366 CrossRef CAS.
- A. Campion and P. Kambhampati, Chem. Soc. Rev., 1998, 27, 241–250 RSC.
- A. Otto, J. Billmann, J. Eickmans, U. Ertürk and C. Pettenkofer, Surf. Sci., 1984, 138, 319–338 CrossRef CAS.
- S. E. J. Bell and N. M. S. Sirimuthu, Analyst, 2004, 129, 1032–1036 RSC.
- Z. Zhang and Y. Wen, Appl. Phys. Lett., 2012, 101, 173109 CrossRef PubMed.
- Y. Wen, W. Wang, Z. Zhang, L. Xu, H. Du, X. Zhang and Y. Song, Nanoscale, 2013, 5, 523–526 RSC.
- Z. Liu, Z. L. Yang, L. Cui, B. Ren and Z. Q. Tian, J. Phys. Chem. C, 2007, 111, 1770–1775 CAS.
- X. Zhou, F. Zhou, H. Liu, L. Yang and J. Liu, Analyst, 2013, 138, 5832–5838 RSC.
- A. Wolosiuk, N. G. Tognalli, E. D. Martínez, M. Granada, M. C. Fuertes, H. Troiani, S. A. Bilmes, A. Fainstein and G. J. A. A. Soler-Illia, ACS Appl. Mater. Interfaces, 2014, 6, 5263–5272 CAS.
- S. Wang, L. P. Xu, Y. Wen, H. Du, S. Wang and X. Zhang, Nanoscale, 2013, 5, 4284–4290 RSC.
- P. Ma, F. Liang, Y. Sun, Y. Jin, Y. Chen, X. Wang, H. Zhang, D. Gao and D. Song, Microchim. Acta, 2013, 180, 1173–1180 CrossRef CAS.
- Y. Jin, P. Ma, F. Liang, D. Gao and X. Wang, Anal. Methods, 2013, 5, 5609–5614 RSC.
- H. Yang, M. Deng, S. Ga, S. Chen, L. Kang, J. Wang, W. Xin, T. Zhang, Z. You, Y. An, J. Wang and D. Cui, Nanoscale Res. Lett., 2014, 9, 138 CrossRef PubMed.
- S. Pande, S. K. Ghosh, S. Praharaj, S. Panigrahi, S. Basu, S. Jana, A. Pal, T. Tsukuda and T. Pal, J. Phys. Chem. C, 2007, 111, 10806–10813 CAS.
- I. H. Lee, J. M. Lee and Y. Jung, ACS Appl. Mater. Interfaces, 2014, 6, 7659–7664 CAS.
- K. Kim, K. L. Kim and S. J. Lee, Chem. Phys. Lett., 2005, 403, 77–82 CrossRef CAS PubMed.
- M. J. Banholzer, J. E. Millstone, L. Qin and C. A. Mirkin, Chem. Soc. Rev., 2008, 37, 885–897 RSC.
- J. M. McLellan, A. Siekkinen, J. Chen and Y. Xia, Chem. Phys. Lett., 2006, 427, 122–126 CrossRef CAS PubMed.
- J. F. Li, Y. F. Huang, Y. Ding, Z. L. Yang, S. B. Li, X. S. Zhou, F. R. Fan, W. Zhang, Z. Y. Zhou, Y. WuDe, B. Ren, Z. L. Wang and Z. Q. Tian, Nature, 2010, 464, 392–395 CrossRef CAS PubMed.
- Z. Zhang, C. Zhao, Y. Ma and G. Li, Analyst, 2014, 139, 3614–3621 RSC.
- K. Zhang, L. Liang, M. Huang, Y. Hu and G. Li, Microchim. Acta, 2014, 181, 1301–1308 CrossRef CAS.
- K. Leopold, M. Foulkes and P. Worsfold, Anal. Chim. Acta, 2010, 663, 127–138 CrossRef CAS PubMed.
- E. M. Nolan and S. J. Lippard, Chem. Rev., 2008, 108, 3443–3480 CrossRef CAS PubMed.
- E. Álvarez-Ayuso, A. García-Sánchez and X. Querol, Water Res., 2003, 37, 4855–4862 CrossRef PubMed.
- I. Sanchez Trujillo, A. Garcia de Torres, E. I. Vereda Alonso and J. M. Cano Pavon, J. Anal. At. Spectrom., 2013, 28, 1772–1780 RSC.
- T. Lasanta, J. M. Lopez-de-Luzuriaga, M. Monge, M. E. Olmos and D. Pascual, Chem.–Eur. J., 2013, 19, 4754–4766 CrossRef CAS PubMed.
- H. Zhang, L. Yang, B. Zhou, W. Liu, J. Ge, J. Wu, Y. Wang and P. Wang, Biosens. Bioelectron., 2013, 47, 391–395 CrossRef CAS PubMed.
- S. Chen, D. Liu, Z. Wang, X. Sun, D. Cui and X. Chen, Nanoscale, 2013, 5, 6731–6735 RSC.
- B. R. Wood, P. Heraud, S. Stojkovic, D. Morrison, J. Beardall and D. McNaughton, Anal. Chem., 2005, 77, 4955–4961 CrossRef CAS PubMed.
- F. Li, J. Wang, Y. Lai, C. Wu, S. Sun, Y. He and H. Ma, Biosens. Bioelectron., 2013, 39, 82–87 CrossRef CAS PubMed.
- T. Senapati, D. Senapati, A. K. Singh, Z. Fan, R. Kanchanapally and P. C. Ray, Chem. Commun., 2011, 47, 10326–10328 RSC.
- Y. Du, R. Liu, B. Liu, S. Wang, M.-Y. Han and Z. Zhang, Anal. Chem., 2013, 85, 3160–3165 CrossRef CAS PubMed.
- W. Ma, M. Sun, L. Xu, L. Wang, H. Kuang and C. Xu, Chem. Commun., 2013, 49, 4989–4991 RSC.
- M. Beija, C. A. M. Afonso and J. M. G. Martinho, Chem. Soc. Rev., 2009, 38, 2410–2433 RSC.
- G. Lu, H. Li, C. Liusman, Z. Yin, S. Wu and H. Zhang, Chem. Sci., 2011, 2, 1817 RSC.
- T. T. B. Quyen, C. C. Chang, W. N. Su, Y. H. Uen, C. J. Pan, J. Y. Liu, J. Rick, K. Y. Lin and B. J. Hwang, J. Mater. Chem. B, 2014, 2, 629–636 RSC.
- E. V. Formo, S. M. Mahurin and S. Dai, ACS Appl. Mater. Interfaces, 2010, 2, 1987–1991 CAS.
- W. Kiefer and S. Schlücker, Surface enhanced Raman spectroscopy: analytical, biophysical and life science applications, John Wiley & Sons, 2013 Search PubMed.
- L. Feng, X. Wu, L. Ren, Y. Xiang, W. He, K. Zhang, W. Zhou and S. Xie, Chem. Eur. J., 2008, 14, 9764–9771 CrossRef CAS PubMed.
- J. F. Li, X. D. Tian, S. B. Li, J. R. Anema, Z. L. Yang, Y. Ding, Y. F. Wu, Y. M. Zeng, Q. Z. Chen, B. Ren, Z. L. Wang and Z. Q. Tian, Nat. Protoc., 2013, 8, 52–65 CrossRef CAS PubMed.
- D. Wu, W. Huang, C. Duan, Z. Lin and Q. Meng, Inorg. Chem., 2007, 46, 1538–1540 CrossRef CAS PubMed.
- M. Tian, X. Peng, J. Fan, J. Wang and S. Sun, Dyes Pigm., 2012, 95, 112–115 CrossRef CAS PubMed.
- C. J. Orendorff and C. J. Murphy, J. Phys. Chem. B, 2006, 110, 3990–3994 CrossRef CAS PubMed.
- L. Wang, Y. Sun, J. Wang, J. Wang, A. Yu, H. Zhang and D. Song, Colloids Surf., B, 2011, 84, 484–490 CrossRef CAS PubMed.
- K. Zhang, Y. Hu and G. Li, Talanta, 2013, 116, 712–718 CrossRef CAS PubMed.
- J. Fan, M. Hu, P. Zhan and X. Peng, Chem. Soc. Rev., 2013, 42, 29–43 RSC.
- H. Li, J. Fan and X. Peng, Chem. Soc. Rev., 2013, 42, 7943–7962 RSC.
- M. Moskovits, Rev. Mod. Phys., 1985, 57, 783 CrossRef CAS.
Footnote |
† Electronic supplementary information (ESI) available. See DOI: 10.1039/c5ra04423e |
|
This journal is © The Royal Society of Chemistry 2015 |