DOI:
10.1039/C5RA03960F
(Paper)
RSC Adv., 2015,
5, 40429-40436
Effect of zinc
:
cobalt composition in ZnCo2O4 spinels for highly selective liquefied petroleum gas sensing at low and high temperatures†
Received
5th March 2015
, Accepted 13th April 2015
First published on 14th April 2015
Abstract
Nano ZnCo2O4 mixed phase materials were synthesized at varying zinc and cobalt ratios such as 1
:
1, 1
:
1.5, 1
:
2, 1
:
2.5 and 1
:
3. With a change in composition from 1
:
1 to 1
:
2.5, the gas sensing response characteristics increased two times (from 40 to 77.5 kΩ), while at higher zinc cobalt composition (1
:
3) a saturation point is shown (about 80 kΩ). This shows that optimal cobalt loading (1
:
2.5) leads to a two times enhancement in redox ability (from 174 to 346 mmol) and number of active sites, and this upshot significantly helps the sensing response reach a much lower 10 ppm and increases the saturation point to a higher 60 ppm LPG concentration. Furthermore, nano ZnCo2O4 (with a zinc and cobalt ratio of 1
:
2.5) material exhibited an excellent response time (∼80–90 s), rapid recovery time (∼65–75 s), excellent repeatability (fourth cycle), good selectivity (for LPG), higher gas response (∼77.5 kΩ), lower as well as higher operating temperature (from 30 to 250 °C). The results clearly reveal that by tuning cobalt composition in ZnxCo2−xO4, we can achieve maximum sensing efficiency and repeatability.
Introduction
Liquefied petroleum gas (LPG) is an important chemical raw material widely used as fuel for domestic and industrial purposes to provide a clean source of energy. It is a combustible gas which mainly consists of butane, propane, propylene, butylene, ethylene, methane and carbon monoxide.1 However, LPG is dangerous because of its extreme flammability.1–4 LPG leakage may take place at any facilities for LPG production or during transportation, storage and application. Therefore, LPG leakage monitoring is extremely important in these facilities.
In previous decades, various efforts have been made to improve the chemical sensing activities of sensing materials, but only a few effective sensing materials for LPG gas have been reported.1–4 This has stimulated considerable interest for scientific research to develop simple and cost-effective chemical sensors for the detection of LPG in recent years and many efforts, in this field, are today devoted to the synthesis of novel sensing materials with enhanced performance.2,5–8 Up until now, semiconductors, especially metal oxides, have been widely investigated as gas sensing materials because of their low cost and power consumption, simplicity of fabrication and use, versatility in detecting a wide range of flammable/toxic gases, and stability in harsh environments.9,10 Recent studies reveal that nanomaterials have ultrahigh sensitivity to different gases due to their small particle size and large surfaces were prepared using different morphologies (i.e. in the form of nanorods, nanoparticles, nanowires, worm like, etc.) of single and mixed phase metal oxide materials like Pd, Fe, Cd, Sn, Cu, In, Pt, Zn, Cd, In and Co.1–4,6–28 However, major drawbacks associated with these systems are: the use of expensive noble metals, multistep syntheses, sensor activity limited to room temperature, sensor deactivation due to formation of inactive metal species and blockage of active species by chemi-adsorption of unreacted intermediates to the active species.29,30
Recently, our group has been focusing on developing efficient semiconductors and efficient catalytic materials for solar light harvesting.31,32 The Co3O4 and ZnO nanoparticles serve as the active sites of redox reactions, which result in high activity for gas sensing materials.33 Cobalt and zinc are the most attractive elements for gas sensing materials because of their strong ability to activate molecular gases (oxygen, LPG, CO2, etc.) and are also more cost effective than the noble metals. In spite of these facts, a single report is found on LPG gas sensing using mixed phase ZnCo2O4 as a sensing material.1 This study showed the results of ZnCo2O4 sensing materials only at high temperature. Whereas other aspects, such as optimization of cobalt and zinc composition in ZnCo2O4 in order to enhance surface active species, stability/reusability, interaction of LPG molecules over a semiconductor via a mechanistic pathway, and sensing response at low as well as high concentration and temperature, were not explicated well.
In order to overcome the problems associated with existing sensor materials, we have developed ZnCo2O4 nanomaterials with varying cobalt and zinc composition. Furthermore in order to achieve more surface active sites, uniform crystallite size and high surface area, samples are heat treated at different calcination temperatures.30 Hence, we prepared mixed phase zinc cobalt oxide sensing materials by varying the above preparation parameters and these samples were then evaluated for gas sensing at room temperature as well as at high temperature for gases such as LPG, H2, ethanol, CO, CO2, and NH3. The nano-structured ZnCo2O4 catalyst prepared under optimized conditions exhibited excellent gas sensing characteristics. Prepared catalysts were characterized by various techniques like TGA, XRD, XPS, HRTEM and temperature programmed reduction (TPR) measurement.
Experimental
The synthesis of nano ZnCo2O4 was done using an already reported method.30,34,35 For the synthesis of Co3O4 nanorods, the cobalt hydroxyl carbonate precursor was prepared through a low cost chemical co-precipitation/digestion method in alkaline conditions maintaining a pH ∼ 7–8 at 60 °C. The solubility diagram for the cobalt–water–carbonate system was reported by Lewis et al.,36 which revealed the precipitation of water free cobalt carbonate above 160 °C. However, cobalt hydroxyl carbonate is preferentially precipitated at room temperature in the pH range of 7.5–10.0. It has been reported that the cobalt hydroxyl carbonate precursor particles are not well crystallized at room temperature.37 Therefore, we made certain to carry out the co-precipitation/digestion reaction at 60 °C to obtain better crystallinity of the cobalt hydroxyl carbonate precursor. Following the same procedure, we have made an attempt to prepare the mixed precursor in alkaline conditions maintaining a pH ∼ 7–8 at 60 °C. When potassium carbonate, cobaltous nitrate and zinc nitrate solutions are separately dropped into stirred distilled water kept at 60 °C, the following chemical reaction occurs to precipitate a mixed precursor consisting of cobalt hydroxyl carbonate and zinc hydroxyl carbonate at pH ∼ 7–8: |
Co2+ + Zn2+ + OH1− + CO32− + H2O → [Co(OH)1−x(CO3)1−x + Zny(OH)1−x(CO3)1−x]n·H2O
| (1) |
As stoichiometric quantities such as 1
:
1, 1
:
1.5, 1
:
2, 1
:
2.5 and 1
:
3 of zinc
:
cobalt cationic solutions were used during preparation, it was anticipated that the mixed precursor (cobalt hydroxyl carbonate + zinc hydroxyl carbonate) was precipitated by reaction (1) in stoichiometric quantity with uniform mixing of the respective components. It is reported that the zinc hydroxyl carbonate with composition {Zn4(OH)6(CO3)·H2O} is precipitated at pH ∼ 7.5–8.0, provided factors such as M2+ and/or M2+/M3+ ion concentration, their ratio, pH and temperature are carefully controlled to obtain a single phase product during synthesis. As stoichiometric quantities of M2+ and/or M2+/M3+ ions were used for the synthesis of the ZnCo2O4 spinel in the present study, it is therefore reasonable to expect that the mixed precursor with composition shown by reaction (1) will be precipitated during our synthesis protocol. The importance of this process is that nano spinel (mixed oxide) material is prepared without any templates or capping and by calcining the single molecular precursors at low temperature in air.
The structure of the calcined sample ZC-1–5 was investigated using X-ray diffraction (XRD). The X-ray diffraction patterns were recorded using a Rigaku diffractometer (Miniflex Model, Rigaku, Japan) (Cu Kalpha λ = 0.1542 nm). High resolution transmission electron microscopy (HRTEM) was used to determine the particle size and the morphology of the nano-sized powder with a JEOL 1200 EX. The X-ray photoelectron spectroscopy (XPS) analysis was carried out using a V.G. Microtech Scientific spectrometer, ESCA 3000, U.K., equipped with two ultrahigh vacuum chambers. The pressure in the chambers during the experiments was about 10−9 Pa. The XPS spectra were recorded with monochromatized Mg K radiation (photon energy = 1253.6 eV) at a constant 50 eV pass energy. The core level binding energies were corrected with the C 1s binding energy of 284.6 eV. The nanostructured ZnCo2O4 powder was pressed into pellets under a pressure of 15 MPa and the ohmic contacts were made with the help of silver paste to form the sensing element. The gas sensing studies were carried out on these sensing elements in a static gas chamber to sense LPG in ambient air. The sensing element was kept directly on a heater in the gas chamber and the temperature was varied from 50 to 400 °C. The temperature of the sensing element was monitored by a chromel–alumel thermocouple placed in contact with the sensor. A known volume of the LPG was introduced into the gas chamber prefilled with air and it was maintained at atmospheric pressure. The electrical resistance of the sensing element was measured before and after exposure to LPG using a sensitive digital multimeter (METRAVI 603). The performance of the sensing element is presented in terms of gas response (S), which is defined as:
|
 | (2) |
where,
Rair and
Rgas are the electrical resistance values of the sensor element in air and in the presence of LPG gas, respectively.
Results and discussion
XRD results
XRD patterns of ZnCo2O4 spinel samples with various compositions (Zn
:
Co from 1
:
1 to 1
:
3) calcined at 500 °C are presented in Fig. 1. All the samples exhibit diffraction peaks at 2θ = 31.2° (220), 36.1° (311), 44.7° (400), 59.1° (422) and 65.1° (511), which were attributed to the spinel phase of ZnCo2O4 (*) and ZnO (#) phases. The calculated lattice parameter for all the samples was found to be 8.101 Å which matched with the reported value for the spinel ZnCo2O4 and ZnO phases (JCPDS 81-2299 and 80-0075). With change in Zn
:
Co ratio from 1
:
1 to 1
:
3, a slight decrease in peak broadening and increase in peak intensity was observed for the peaks at 2θ = 31.2° (220), 36.1° (311), 44.7° (400), 59.16° (422) and 65.12° (511), indicating an improvement in the crystallinity. The crystallite sizes of the ZC-1 to ZC-5 samples were calculated for the most intense peak at 2θ = 36.1° (311) using the Scherrer equation and were found to be ∼20, 31, 38, 46 and 61 nm respectively. Thus, the increase in crystallite size for the samples, ZC-2, ZC-3, ZC-4 and ZC-5 was mainly due to the restructuring of the surface at 500 °C.32,38
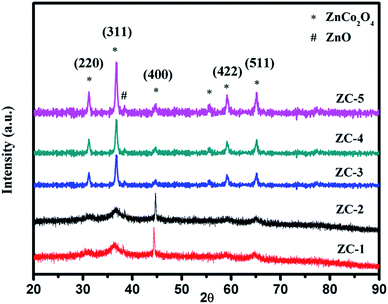 |
| Fig. 1 XRD patterns of nanostructured ZnCo2O4 prepared with different Zn : Co compositions. | |
Thermal gravimetric analysis
Fig. 2 depicts the TGA curve of the as-prepared mixed precursor, which exhibits two major weight losses in the temperature ranges of 50–210 °C and 210–420 °C. In the first step, the weight loss occurs practically from room temperature to 210 °C, with a weight loss of 13% mainly due to the loss/removal of water. The second weight loss step is observed from 210 °C and is continued up to 420 °C, with a weight loss of 22%. It is associated with the decarbonation occurring during decomposition. The formation temperature is found to be comparatively lower than that reported for the corresponding solid state reaction route and co-precipitation at higher pH.1,39
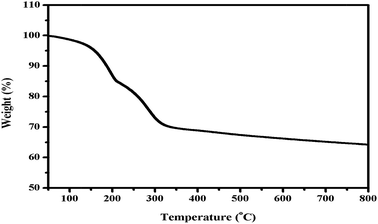 |
| Fig. 2 TGA curve of the mixed precursor. | |
Surface morphology
The HRTEM images of the calcined sample ZC-4 are shown in Fig. 3. As can be seen from Fig. 3(A), most of the ZnCo2O4 particles are in the form of rods (diameter = 6–8 nm, length = 30–50 nm) with the presence of a few spherical crystallite particles of size ∼4–7 nm in the calcined sample ZC-4, which was in good agreement with the XRD results. The parallel lattice fringes across almost all the primary particles are clearly visible (Fig. 3(A1)) which confirms the oriented aggregation of the nanoparticles of ZnCo2O4. It is clearly seen that the aggregated ZnCo2O4 rod like particles are composed of many small ZnCo2O4 nanoparticles. However, the characteristic planes, (311) and (400), obtained from the SAED image (Fig. 3(A2)) were matched with the XRD data. Thus HRTEM and XRD results indicate formation of the ZnCo2O4 nanoparticles.
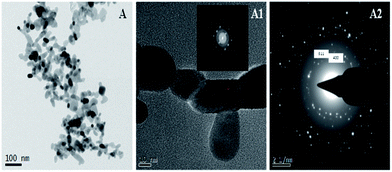 |
| Fig. 3 HRTEM image of (A) nanorod aggregates, (A1) high resolution image of primary nanoparticles and (A2) SAED image of the ZC-4 sample. | |
Surface analysis
The XPS spectra of the ZC-4 sample is shown in Fig. 4. The Co 2p spectra showed two peaks with binding energies at 780.0 and 795.3 eV, corresponding to the Co 2p3/2 and Co 2p1/2 core level peaks.30 The Co 2p3/2 and Co 2p1/2 peak separation value obtained was 15.3 eV, comparable to that observed for the Co3O4 spinel (Fig. 4(a)). A small hump observed at a binding energy of 787.7 eV was a characteristic satellite peak of Co3+.1,40 The Zn 2p spectra for all the samples showed two peaks with binding energies at 1020 and 1043 eV, which were assigned to Zn 2p3/2 and Zn 2p1/2 levels with a splitting value of 23 eV, confirming the presence of Zn2+ (Fig. 4(b)).31,41 Hence, the above XPS and XRD results confirm the formation of ZnCo2O4 spinel oxide.
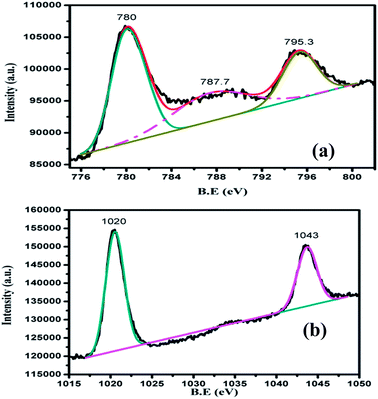 |
| Fig. 4 XPS spectra (a) Co 2p and (b) Zn 2p of the nano spinel ZnCo2O4. | |
Temperature programmed reduction
The number of active sites available for gas sensing is determined by the reducibility of the samples. The reducibility of the ZC-1, ZC-2, ZC-3, ZC-4 and ZC-5 catalysts was studied by the H2-TPR technique (Fig. 5). The peak observed in the range of 180–300 °C in all the samples was attributed to the reduction of trivalent cobalt oxide (ZnCo2O4) to divalent cobalt oxide (CoO). Another broad peak observed in the higher temperature range of 320–580 °C in all the samples could be attributed to the reduction of both divalent cobalt oxide (CoO) and zinc oxide (ZnO) to metallic cobalt (Co0) and zinc (Zn0).30,31,42 With the change in composition from ZC-1 (1
:
1) to ZC-5 (1
:
3) a slight increase in peak intensity was observed, due to an increase in composition of cobalt in the Co3O4 spinel.43 While the change in zinc and cobalt composition from 1
:
1 to 1
:
2.5 resulted in an increase in H2 uptake from 174 to 346 mmol, a further change in composition to 1
:
3 did not remarkably increase H2 uptake (357 mmol). The activities of these catalysts were found to be ∼1.3, 1.7 and 2 times higher than that for the nano ZC-1 spinel catalyst (Table 1). The highest reducibility of the ZC-4 catalyst might be due to uniform distribution of zinc and cobalt species as observed in XPS analysis. This indicates that an increase in cobalt composition improves the reducibility of the ZnCo2O4 spinel and it also confirms that the redox nature was more dependent on the population of Co3+/Co2+ and less on the Zn2+ species in the ZnCo2O4 spinel.30
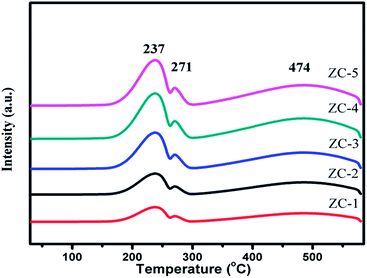 |
| Fig. 5 TPR spectra of the spinel ZnCo2O4 with different compositions. | |
Table 1 TPR-H2 uptake profiles of the ZnCo2O4 nanocatalysts.
Catalyst |
Composition (Zn : Co) |
H2 uptake (mmol) |
ZC-1 |
1 : 1.0 |
174 |
ZC-2 |
1 : 1.5 |
233 |
ZC-3 |
1 : 2.0 |
293 |
ZC-4 |
1 : 2.5 |
346 |
ZC-5 |
1 : 3.0 |
357 |
Formation mechanism of ZnCo2O4 nanorods
When zinc nitrate, cobalt nitrate and potassium carbonate solutions are dropped from separate burettes into distilled water at 60 °C, the following chemical reaction may occur to precipitate zinc cobalt hydroxyl carbonate precursor at pH ∼ 7.0–8.0. The XRD study confirmed the formation of polycrystalline cobalt hydroxyl carbonate with a composition shown by equation:
Co2+ + Zn2+ + OH1− + CO32− + H2O → [Co(OH)1−x(CO3)1−x + Zny(OH)1−x(CO3)1−x]n·H2O |
The probable mechanism of formation of rod shaped morphology in the zinc cobalt hydroxyl carbonate precursor and resulting spinel oxide of ZnCo2O4 is described as given below: initially, the zinc cobalt hydroxy carbonate particles are formed which grow with the increase in digestion time when in contact with a solution having pH ∼ 7.0–8.0. Finally, in calcination treatment decarbonation takes place. Initially, the small particles are formed (ZnCo2O4) and self-align to form rods by an oriental attachment mechanism.
Catalyst screening
Comparison of the activities of the prepared nano ZnCo2O4 materials for LPG gas sensing are presented in Fig. 6. At low temperatures, the gas response is relatively low (∼9.9 at 30 °C), but it increases slowly with an increase in the operating temperature in the case of all samples. While a change in sample from ZC-1 to ZC-4 resulted in a gradual increase in gas sensing response from ∼40 to 77.5 kΩ (at 250 °C), after this a marginal (∼80 kΩ) change is observed for the ZC-5 sample. This increase in gas sensing might be due to increased surface area (from 75 to 90 m2 g−1) and redox ability (174 to 346 mmol) leading to an enhancement in surface active sites (ESI, Table S1†). The gas response attains a maximum at ∼250 °C (∼77.5 kΩ) and thereafter it starts to decline with a further increase in the operating temperature (250–400 °C). The increase in gas sensing ability with increasing temperature (from 30 to 250 °C) might be due to an increase in collision frequency and internal pressure collectively helping to increase the adsorption time of the LPG molecules over ZC-4, hence resulting in the increased response. However, above 250 °C instead of giving a steady response a decrease in gas sensing behaviour is shown, probably due to two possibilities such as oxidation and stability of LPG, these phenomena are explained in brief at the end of this article. Hence, an optimum operating temperature range for the ZnCo2O4 spinel to detect LPG is from 30 to 250 °C, which is modest from the viewpoint of semiconducting oxide gas sensors. This indicates that the nature of the active sites (both cobalt and zinc) in terms of reducibility plays a dominant role in gas sensing. The activity is about two times lower for ZC-1 (reducibility 174 mmol) than ZC-4 (reducibility 346 mmol) and could be attributed to the two times lower number of active sites observed in the TPR analysis. The ZC-5 sample showed marginally higher activity than the ZC-4 sample although its cobalt composition was more than 0.5 times higher than that of ZC-4, indicating that beyond a certain value, increase in cobalt composition does not improve the gas sensing activity of the sensing material. This clearly indicates that the presence of both Co3+ and Zn2+, as well as the total reducibility, is crucial for determining the gas sensing ability for LPG. Since the nano ZC-4 material at an operating temperature of 250 °C was found to be the best catalyst because of its mixed oxidation states and highest number of active sites available for LPG sensing, the nano ZC-4 material was selected for further study to investigate additional LPG sensing properties such as response and recovery times, reproducibility and selectivity.
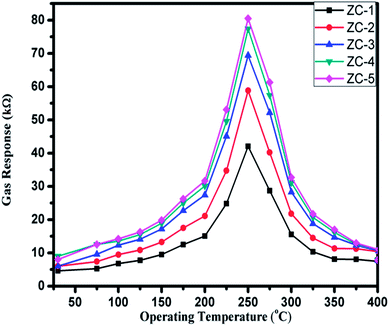 |
| Fig. 6 Effect of temperature (from room to high temperature) on the gas response of the nano ZnCo2O4 materials in the presence of 40 ppm LPG gas. | |
Effect of calcination temperature
The effect of calcination temperature on the sensing performance of the ZnCo2O4 (ZC-4) sample was studied by varying the calcination temperature in a range from 300 to 600 °C, the results of which are shown in ESI, Table S2.† A significant increase in LPG sensing response from 42 to 77 kΩ was observed with a temperature increase from 300 to 500 °C, while further increase in calcination temperature (600 °C) did not increase sensing performance appreciably (79 kΩ). However, such a dramatic increase in sensing activity might be due to the following reasons: calcination at higher temperature (500 °C) might cause the material surface to be free from impurities and probably increases the number of active sites which is in accordance with the TPR, XPS and TGA results. Thus, the above observations established that the optimum calcination temperature was 500 °C for our catalyst in order to achieve the highest gas sensing activity for LPG.
The response and recovery characteristics of the ZC-4 nanomaterial to 40 ppm LPG are shown in Fig. 7. The response and recovery times are important parameters for evaluating the performance of gas sensors. In general, these parameters report the time required for the sensor resistance to change by nineteen percent of the final resistance. It is observed that the resistance of the sensing element increases when exposed to the LPG, which suggests that the ZC-4 nanomaterial behaves as a p-type semiconductor. As can be seen from Fig. 7, the sensor responds rapidly after introduction of LPG and recovers immediately when it is exposed to air. The ZC-4 sensing material requires a response time of ∼80–90 s and a recovery time of ∼65–75 s.
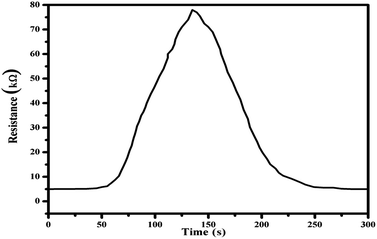 |
| Fig. 7 Response of the ZC-4 nanomaterial to 40 ppm LPG at the optimum operating temperature of 250 °C. | |
The repeatability and constancy of the ZC-4 nanomaterial were investigated by repeating the test four times as shown in Fig. 8 and ESI Fig. S1.† The ZC-4 nanomaterial shows good reproducibility and reversibility upon repeated exposure and removal of LPG under the same conditions. Additionally, the repeated tests revealed that the gas response values were maintained and the recovery abilities were not diminished after four sensing cycles. The ZC-4 nanomaterial showed LPG responses of ∼9.9 and 77.5 kΩ at 30 and 250 °C with the response and recovery times almost the same (∼80–90 and ∼65–75 s), respectively for the exposure of 90–100 s. Hence, the ZC-4 nanomaterial exhibits stable and repeatable characteristics. This indicates that it can be used as a reusable sensing material for the detection of LPG not only at lower temperature but also effectively at higher temperature.
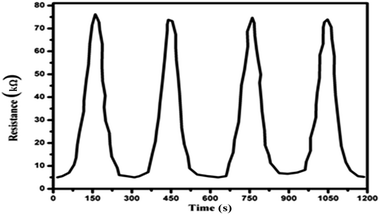 |
| Fig. 8 Repetitive response of the ZC-4 nanomaterial to 40 ppm LPG at the optimum operating temperature of 250 °C. | |
The LPG sensing response of the ZC-4 nanomaterial with LPG concentrations varying from 10 to 80 ppm is shown in Fig. 9. It is observed that the gas response increased with an increase in the LPG concentration up to 40 ppm. Above 40 ppm, the base line remains almost stable and no significant variation in the gas response is observed. The ZC-4 nanomaterial is able to detect as low as 10 ppm of LPG with a good response at the optimum operating temperature of 250 °C.
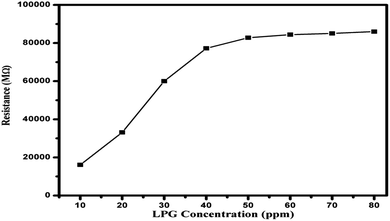 |
| Fig. 9 Response of the ZC-4 nanomaterial upon sequential exposure to LPG with concentrations varying from 10 to 80 ppm. | |
Selectivity is an important parameter of gas sensors and it is the ability of a sensor to respond to a certain gas in the presence of other gases. The gas sensing ability of the ZC-4 nanomaterial towards LPG, ethanol, CO, CO2 and NH3 with concentrations of 40 ppm each were also measured and the results are shown in Fig. 10 (ESI Fig. S2†). Among these gases, ZC-4 is most sensitive to LPG gas (∼77.5), whereas it shows a considerably lower response (<18) to H2, ethanol, CO, CO2 and NH3. This might be due to the fact that LPG gas adsorbs more efficiently than the others leading to a higher sensing response.
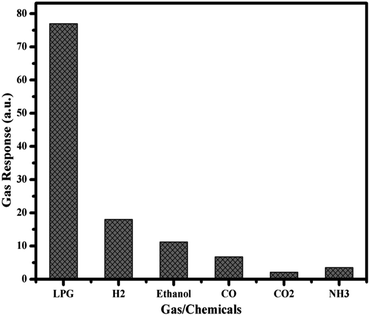 |
| Fig. 10 Bar chart showing the gas response of the ZC-4 nanomaterial for different gases. | |
It is well known that in the spinel ZnCo2O4, two oxidation states viz. Zn2+ and Co3+ coexist and a dynamic equilibrium is set up between them and the lattice oxygen (O2−) species under liquid phase oxidation conditions.29,30,43 As shown in Scheme 1, sensing of gas over the surface is initiated by oxidative dehydrogenation, proceeding via collision of the gas (LPG) with the octahedral cobalt species containing a lattice oxide ion.29,44–46 A hydrogen of the adsorbed LPG gas first forms an inter-molecular bond with the surface oxide ion as shown in Scheme 1. Subsequently, Co3+OH species were formed due to abstraction of the hydrogen by the oxide ion.47 This change in chemical states might be the source of the increase in resistance, and hence why the nanomaterial shows an instantaneous response for chemical changes at the surface. Furthermore, the activated Co3+OH species were capable of forming another intermolecular hydrogen bond and abstracting a second electron to form the major products such as oxygenated LPG (aldehyde, ketones, etc.), Co2+ and water molecules. This self sweep out phenomenon resulted in a decrease in resistance, and thus instantaneous recovery of the surface chemical states. However, the reduced Co2+ species are reoxidized using molecular oxygen to form again active Co3+O− species. The lower sensing ability observed in the case of ethanol, CO, CO2 and NH3 gas/chemicals (Fig. 10) might be due to the lesser ability of the gas/chemicals to form an intermolecular bond between hydrogen and the cobalt oxide ion in the initial step (adsorption) or after the activation of the reactant as shown in Scheme 1. Thus, our results reveal that the interactions of the gas with the active sites were less in the case of ethanol, CO, CO2 and NH3 gas/chemicals resulting in a lower sensing activity.
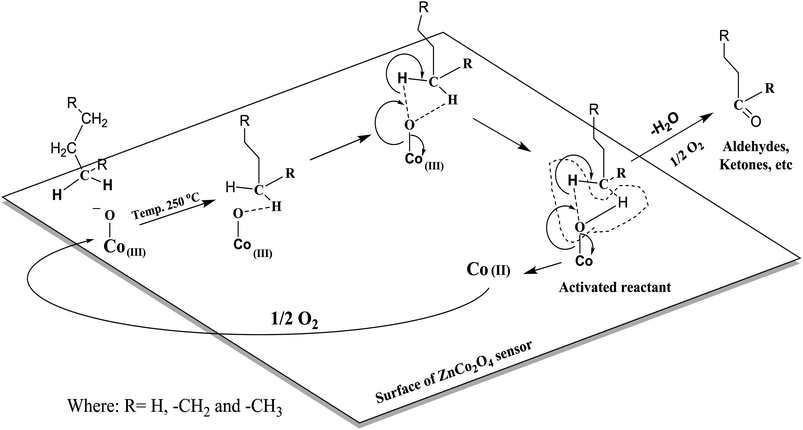 |
| Scheme 1 Plausible sensing pathway for ZnCo2O4 nanomaterial. | |
Conclusions
In summary, a novel method to enhance the sensitivity of nanorods of the ZC-4 sensor was proposed and demonstrated in this paper. The sensitivity of the sensor can be remarkably increased by an increase in cobalt composition (ZnCo composite) from 1
:
1 to 1
:
2.5, which is beneficial for the uniform distribution of Co3+ and Zn2+ active sites as a result of the increase in reducibility, ultimately leading to a two times enhancement in gas sensing. ZC-4 nanorods have good LPG sensing properties such as fast response (∼80–90 s), fast recovery time (∼65–75 s), excellent repeatability (fourth cycle), good selectivity (for LPG), higher gas response (∼77.5 kΩ) to detect a low 10 ppm LPG concentration and wide operating temperature (30–250 °C). These results along with a zinc cobalt composition of 1
:
2.5 demonstrate that surface active Zn2+, Co2+ and Co3+ species influence sensing ability and are promising for large scale fabrication of simple, cost-effective and high performance LPG gas sensors operating not only at low but also at high LPG concentration and temperature.
References
- S. Vijayanand, P. A. Joy, H. S. Potdar, D. Patil and P. Patil, Sens. Actuators, B, 2011, 152, 121–129 CrossRef CAS PubMed.
- N. Wu, M. Zhao, J. G. Zheng, C. Jiang, B. Myers, S. Li, M. Chyu and S. X. Mao, Nanotechnology, 2005, 16, 2878–2881 CrossRef CAS.
- A. Srivastava and R. K. Jain, Mater. Chem. Phys., 2007, 105, 385–390 CrossRef CAS PubMed.
- W. J. Moon, J. H. Yu and G. M. Choi, Sens. Actuators, B, 2002, 87, 464–470 CrossRef CAS.
- T. Zhang, L. Liu, Q. Qi, S. Li and G. Lu, Sens. Actuators, B, 2009, 139, 287–291 CrossRef CAS PubMed.
- K. I. Choi, H. R. Kim and J. H. Lee, Sens. Actuators, B, 2009, 138, 497–503 CrossRef CAS PubMed.
- B. Bahrami, A. Khodadadi, M. Kazemeini and Y. Mortazavi, Sens. Actuators, B, 2008, 133, 352–356 CrossRef CAS PubMed.
- L. Zhang, J. Hu, P. Song, H. Qin, K. An, X. Wang and M. Jiang, Sens. Actuators, B, 2006, 119, 315–318 CrossRef CAS PubMed.
- C. C. Wang, S. A. Akbar and M. J. Madou, J. Electroceram., 1998, 2, 273–282 CrossRef CAS.
- N. Barsan and U. Weimar, J. Electroceram., 2001, 7, 143–167 CrossRef CAS.
- Y. Sun, L. Zhang, J. Zhang, P. Chen, S. Xin, Z. Li and J. Liu, Ceram. Int., 2014, 40, 1599–1603 CrossRef CAS PubMed.
- J. X. Wang, X. W. Sun, H. Huang, Y. C. Lee, O. K. Tan, M. B. Yu, G. Q. Lo and D. L. Kwong, Appl. Phys. A, 2007, 88, 611–615 CrossRef CAS PubMed.
- Y. Jia, L. He, Z. Guo, X. Chen, F. Meng, T. Luo, M. Li and J. Liu, J. Phys. Chem. C, 2009, 113, 9581–9587 CAS.
- X. Liu, C. Zhou, C. Li, D. Zhang, B. Lei and S. Han, J. Phys. Chem. B, 2003, 107, 12451–12455 CrossRef.
- X. Gou, G. Wang, J. Yang, J. Park and D. Wexler, J. Mater. Chem., 2008, 18, 965–969 RSC.
- Q. Wan, T. H. Wang, C. C. Li, Z. F. Du, L. M. Li and H. C. Yu, Appl. Phys. Lett., 2007, 91, 032101 CrossRef PubMed.
- R. B. Waghulade, R. Pasricha and P. P. Patil, Talanta, 2007, 72, 594–599 CrossRef CAS PubMed.
- N. B. Sonawane, R. R. Ahire, K. V. Gurav, J. H. Kim and B. R. Sankapal, J. Alloys Compd., 2014, 592, 1–5 CrossRef CAS PubMed.
- M. S. Dhingra, N. K. Singh, S. Shrivastava, P. S. Kumar and S. Annapoorni, Sens. Actuators, A, 2013, 190, 168–175 CrossRef CAS PubMed.
- S. Nath, S. S. Nath and R. K. Kumar, J. Anal. Sci. Technol., 2012, 3(1), 85–94 Search PubMed.
- R. B. Ravikiran, G. Arindam, G. Anil, S. Fouran and R. Sharma, Sens. Actuators, B, 2011, 160(1), 1050–1055 CrossRef PubMed.
- R. J. Deokate, D. S. Dhawale and C. D. Lokhande, Sens. Actuators, B, 2011, 156(2), 954–960 CrossRef CAS PubMed.
- R. Aad, V. Simic, L. Le Cunff, L. Rocha, V. Sallet, C. Sartel, A. Lusson, C. Couteau and G. Lerondel, Nanoscale, 2013, 5, 9176–9180 RSC.
- D. C. Pugh, E. J. Newton, A. J. T. Naik, S. M. V. Hailes and I. P. Parkin, J. Mater. Chem. A, 2014, 2, 4758–4764 CAS.
- P. Wang, Y. Fu, B. Yu, Y. Zhao, L. Xing and X. Xue, J. Mater. Chem. A, 2015, 3, 3529–3535 CAS.
- Y. Liu, J. Dong, P. J. Hesketh and M. Liu, J. Mater. Chem., 2005, 15, 2316–2320 RSC.
- M. V. Reddy, G. V. Subba Rao and B. V. R. Chowdari, Chem. Rev., 2013, 113, 5364–5457 CrossRef CAS PubMed.
- M. V. Reddy, Z. Beichen, K. P. Loh and B. V. R. Chowdari, CrystEngComm, 2013, 15, 3568–3574 RSC.
- A. Bielanski and J. Haber, Catal. Rev.: Sci. Eng., 1979, 19, 1–41 CAS.
- V. R. Mate, A. Jha, U. D. Joshi, K. R. Patil, M. Shirai and C. V. Rode, Appl. Catal., A, 2014, 487, 130–138 CrossRef CAS PubMed.
- S. R. Kadam, V. R. Mate, R. P. Panmand, L. K. Nikam, M. V. Kulkarni, R. S. Sonawane and B. B. Kale, RSC Adv., 2014, 4, 60626–60635 RSC.
- V. R. Mate, M. Shirai and C. V. Rode, Catal. Commun., 2013, 33, 66–69 CrossRef CAS PubMed.
- D. Patil, P. Patil, S. Vijayanand, P. A. Joy and H. S. Potdar, Talanta, 2010, 81, 37–43 CrossRef CAS PubMed.
- G. Pantaleo, G. Deganello, L. F. Liotta and G. D. Carlo, Catal. Commun., 2006, 5, 329–336 Search PubMed.
- C. G. Gao, D. S. Liu, Y. Z. Wang and Y. X. Zhao, Catal. Lett., 2007, 116, 136–142 CrossRef.
- S. Lacour, R. P. Van Hille, K. Peterson and A. E. Lewis, AIChE J., 2005, 51, 2358–2368 CrossRef CAS PubMed.
- M. Oku and Y. Sato, Appl. Surf. Sci., 1992, 55, 37–41 CrossRef CAS.
- J. Yang, H. Liu, W. N. Martens and R. L. Frost, J. Phys. Chem. C, 2009, 114, 111 Search PubMed.
- K. Omata, T. Takada and S. Kasahara, Appl. Catal., A, 1996, 146, 255–267 CrossRef CAS.
- T. J. Chuang, C. R. Brundle and D. W. Rice, Surf. Sci., 1976, 59, 413–429 CrossRef CAS.
- I. Grohmann, B. Peplinski and W. Unger, Surf. Interface Anal., 1992, 19, 591–594 CrossRef CAS PubMed.
- D. G. Klissurski and E. L. Uzunova, J. Mater. Sci. Lett., 1990, 9, 576–579 CrossRef.
- J. J. Rehr, S. D. Conradson, A. L. Ankudinov and B. Ravel, Phys. Rev. B: Condens. Matter Mater. Phys., 1998, 58, 7565–7576 CrossRef.
- P. A. Rock, Inorg. Chem., 1968, 7, 837–840 CrossRef CAS.
- R. A. Sheldon and R. A. van Santen, Catalytic Oxidation: Principles and Applications, World Scientific, Singapore, 1995 Search PubMed.
- G. Centi, F. Cavani, F. Trifiro and M. V. Twigg, Fundamental and Applied Catalysis Selective Oxidation by Heterogeneous Catalysis, KluwerAcademic/Plenum Publishers, New York, 2001 Search PubMed.
- B. Gillot, M. Laarj, P. Tailhades and A. Rousset, Mater. Chem. Phys., 1988, 19, 485–495 CrossRef CAS.
Footnote |
† Electronic supplementary information (ESI) available. See DOI: 10.1039/c5ra03960f |
|
This journal is © The Royal Society of Chemistry 2015 |