DOI:
10.1039/C5RA03593G
(Paper)
RSC Adv., 2015,
5, 26977-26984
Adsorption and sustained release of haemoglobin imprinted polysiloxane using a calcium alginate film as a matrix
Received
28th February 2015
, Accepted 10th March 2015
First published on 10th March 2015
Abstract
A calcium alginate (CA) hydrogel film was prepared by cross-linking sodium alginate (SA) with a CaCl2 solution. Haemoglobin (Hb) molecularly imprinted polysiloxane (MIP) was synthesized using silane as a functional monomer, Hb as a template and a CA hydrogel film as a matrix. SEM, TEM, TG and a water contact angle test were used to characterize the CA hydrogel and MIP film. The adsorption and recognition properties of MIPs were evaluated and the results showed that the MIP exhibited a significant improvement in terms of rebinding capacity for Hb compared with non-imprinted polysiloxane (NIP). Haemoglobin imprinted polysiloxane could selectively recognize the template protein from a mixture of other competitive proteins. The surface hydrophilicity and the swelling behaviour of the MIP film can be adjusted by changing the ratio of two types of silane. Hb was released from the MIP in a sustained manner in PBS and Tris–HCl solutions. The Higuchi model fits best for the MIP film no matter whether it is in PBS or in a Tris–HCl solution. It is a diffusion-controlled release mechanism for the MIP film. But diffusion is not the key factor for release of the NIP film.
1. Introduction
The controlled release of peptides and proteins has aroused widespread interest. However, protein release from many materials shows burst release behaviour due to the weak bonds between proteins and the carriers.1–4 Molecular imprinting is a promising and evolving technology to synthesize tailor-made materials with complementary binding sites and specific recognition cavities for the template.5,6 The molecularly imprinted polymer (MIP) can inherently act as a reservoir for drug molecules and increase the residence time of the drug due to the specific recognition cavities.7–13 Carmen Alvarez-Lorenzo et al.10 introduced norfloxacin (NRF) imprinted soft contact lenses with the ability to load NRF and to control its release. After immersion in 0.025, 0.050 and 0.10 mM drug solutions, the imprinted hydrogels loaded greater amounts of NRF than the non-imprinted ones, and the imprinted hydrogels showed a greater ability to control the release process with sustained release for more than 24 h. Cai et al. synthesized MIP for tetracycline using methacrylic acid monomer and found that the tetracycline release from MIP was slower than that from NIP.12 However, several challenges still remain in protein imprinting because of the flexibility and complexity of proteins.14–18 The diffusion of proteins is difficult.
Polyacrylamide hydrogel has often been used for the imprinting of proteins. But the hydrogel lack sufficient thermo stability and mechanical strength, and the diffusion of protein in the hydrogel is very slow. Surface imprinting technique can be employed to resolve the problem of protein diffusion.19–21 Silanes and their derivatives were used to prepare surface molecularly imprinted polysiloxane on solid substrate. Silanes possess numerous features such as temperature resistance, physical inertia, and can easily create polysiloxane networks by sol–gel. Multiple hydrogen bonds can be formed between the silanol groups of polysiloxane and the surface polar residues of proteins. The molecularly imprinted polysiloxane can be prepared using silanes by an aqueous imprinting method without any organic chemicals, which was favourable in life science applications such as drug delivery systems.
Calcium alginate is biocompatible and has been successfully used in drug release,22 food, beverage and pharmaceutical.23,24 Calcium alginate imprinted bovine serum albumin (BSA) film was prepared and showed sustained release of BSA from the matrix.11 In our previous work, calcium alginate based hydrogel microspheres were used as the matrix for the imprinting of proteins.17,24,25 However, organic solvents are often used as dispersion medium to prepare the microspheres, which inevitably causes protein denaturation. Besides, particles pose the problem of post-processing which requires tedious steps to recover them.
In this paper, calcium alginate (CA) hydrogel films were prepared by cross-linking sodium alginate (SA) with CaCl2 solution. Haemoglobin molecularly imprinted polysiloxane (MIP) was synthesized using silane as the functional monomer, Hb as the template and CA hydrogel film as the matrix in an aqueous phase. The adsorption and recognition properties of the MIP were evaluated. The sustained release behaviours of Hb from the MIP were shown in phosphate buffer solution (PBS) and Tris–HCl solutions. Compared with NIP, MIP exhibited slower and sustained release of Hb.
2. Experimental
2.1 Materials
Sodium alginate (SA, chemical grade) were purchased from Northern China Medical Chemical Reagent (Tianjin, China). Oxalic acid and CaCl2 (analytical grade) were from Shanghai Shen Xiang Chemical Reagent Co. Ltd. Ethylenetri(β-methoxy)ethyoxysilane (KH-570) and γ-amidopropyltriethyoxysilane (KH-550) were supplied by Tianjin Shengbin Chemical Plant (Tianjin, China), analytical grade. Bovine serum albumin (BSA), ovalbumin (OVA), bovine hemoglobin (Hb) and bovine γ-globulin (Glo) were purchased from Shanghai Science and Technology Development Company (Shanghai, China). Phosphate buffer (PBS) and Tris–HCl buffer were offered by Tianjin no. 2 Chemical Reagent Factory (analytical grade). Distilled water was used throughout the experiments.
2.2 Preparation of calcium alginate (CA) hydrogel films
Firstly, 0.3046 g, 0.4082 g, 0.5128 g, 0.6186 g, and 0.7254 g of SA powders were added respectively into five beakers containing 20 mL water and the mixtures were stirred for 120 minutes. After ultrasonic processing for 5 minutes, the SA solutions were put aside for 12 h to eliminate bubbles. About 3–10 g of the viscous SA solution was scraped into films on a glass plate using a glass rod twined copper wire with a diameter of 0.4 mm. Then the glass plate was respectively put into CaCl2 solution with concentrations of 1.5 wt%, 2.5 wt%, 3.5 wt%, and 2.5 wt% for cross-linking. The resulted calcium alginate (CA) hydrogel films were saved in 1 wt% CaCl2 aqueous solution.
2.3 Preparation of CA film based MIP and NIP
About 0.100 g of CA films was placed in a glass vial and 10 mL of 1.34 mg mL−1 Hb solution was added. After 12 hours, 0.050 mL KH-550 or 0.050 mL KH-570 silanes was added into separate CA/Hb containing vials. After two days' incubation at 20 °C for the hydrolysis and condensation polymerization of the silanes, the supernatant was removed. The CA films coated with polysiloxane were washed with 10 mL of 0.5 mol L−1 oxalic acid solution to remove the template Hb. After washing with distilled water to remove oxalic acid, Hb molecularly imprinted polysiloxane was prepared and noted as MIP. Fig. 1 shows the schematic fabrication procedure of CA film based MIP.
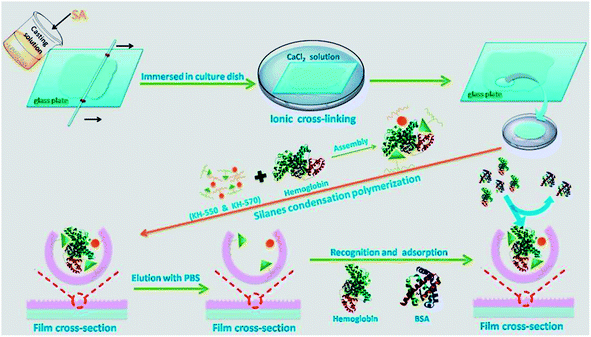 |
| Fig. 1 Schematic representation of the fabrication procedure of CA film based MIP. | |
Non-imprinted polysiloxane (NIP) was also fabricated according to the above procedures except that the Hb solution was replaced by distilled water.
2.4 Characterization
The CA films prepared with 3.0 wt% SA and 2.5 wt% CaCl2 as well as MIP based on the CA films were fully characterized. The thicknesses of wet CA and MIP films were measured with a digital display micrometer gauge from Shanghai Luchuan Co., Ltd. The morphologies of CA film and MIP were observed by scanning electron microscope (SEM; S-4800, HITACHI, Japan) and transmission electron microscope (TEM, FEI Tecnai G2 F30).
Thermal gravimetric analysis of polysiloxane, CA and MIP film was performed under air atmosphere with airflow of 25 mL min−1 (TGA; PerkinElmer, TGA-7).
Surface hydrophilicity of CA and MIP film was characterized by water contact angle measurements (OCA20, Dataphysics Instruments with GmbH, Germany) at 20 °C.
The swelling ratio (SR) of CA and MIP films in PBS solution was determined by measuring the weight of the wet film in different swelling state. At different time points, the mass of wet film was accurately weighed using a microbalance after absorbing the surface water from the film with a filter paper. Then the wet film was placed back into PBS solutions. The swelling rate (SR) is calculated as follows:
|
SR = 100% × (Ws − W0)/W0
| (1) |
W0 is the initial weight of the film and
Ws is the weight of the film at different swelling times.
The mechanical properties of films were tested using a tensile testing machine (LLY-06F, Laizhou Electronic Instrument Co., Ltd., China) in wet form.
2.5 Adsorption thermodynamics of MIP and NIP
Adsorption experiments were carried out using a batch-wise adsorption method. MIP and NIP (100 mg) were separately put into individual glass bottle containing various concentrations (0–2.00 mg mL−1) of Hb to determine the adsorption isotherms and the imprinting efficiency. Hb concentrations were measured by an ultraviolet spectrophotometer at certain time intervals. Equilibrium rebinding capacity Qe (mg g−1) of protein onto the MIP and NIP was determined according to eqn (2).where C0 and Ce (mg mL−1) is the initial and final protein concentrations (mg mL−1) in solutions, V (mL) is the volume of Hb solution and W (g) is the mass of MIP or NIP in dried form.
The imprinting efficiency (IE) of MIP was defined as follows:
where
QMIP and
QNIP are the
Qe of MIP and corresponding NIP, respectively.
2.6 Recognition performance of MIP
MIP and NIP (100 mg) were put into glass bottles containing 10 mL of 1.36 mg mL−1 Hb, Glo, OVA and BSA solutions respectively to evaluate the recognition performance. Protein mixture containing 1.36 mg mL−1 OVA, Hb, BSA and Glo was also used to investigate the recognition performance. The protein mixture (10 mL) before and after adsorbed by MIP and NIP was subjected to SDS-PAGE analysis and proteins were visualized using silver staining.26
2.7 Release of Hb from MIP and NIP films
The Hb rebinded MIP and NIP films were put into 5 mL of phosphate buffer solution (PBS, pH = 7.4) or Tris–HCl solution (pH = 7.4) to study the release mode of Hb in a sterile incubator. The amounts of Hb released at different times were detected by TU-1901 ultraviolet spectrophotometer.3
Three types of kinetic models were utilized to describe the release profile, and the correlation coefficient values (R2) demonstrated how well the models described the release process. They are as follows:
Zero-order rate model:
First-order rate model:
Higuchi model:
where
C0 is the initial protein concentration and
Ct is the concentration of protein at time
t. The release rates are
k0,
k1 and
kH.
3. Results and discussions
3.1 Characterizations of CA and MIP film
SEM images of CA and MIP films are presented in Fig. 2. The CA film (Fig. 2a) was smoother than MIP film (Fig. 2b). Substantial amount of polysiloxane particles with size ranged from 50 to 400 nm appeared on the surface of MIP film. NIP film exhibited similar surface morphology to MIP via SEM observation. The surface polysiloxane of MIP decreased the transparency of CA film and increased the thickness (form 0.127 ± 0.022 mm to 0.255 ± 0.031 mm).
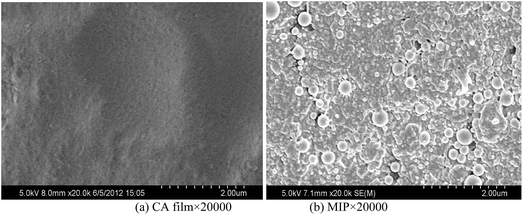 |
| Fig. 2 SEM images of CA film (a) and MIP (b). | |
Fig. 3 shows the TEM images of MIP film. Hb imprinted polysiloxane nanoparticles with the diameter of 50–300 nm were found on the surface of the MIP. The size of the polysiloxane nanospheres was consistent with the results observed by SEM.
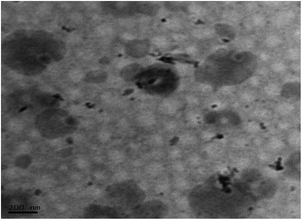 |
| Fig. 3 TEM images of MIP. | |
Fig. 4 shows the TG curves of polysiloxane particles, CA, and MIP film. The polysiloxane particles showed the minimum weight loss which was caused by bound water evaporation (200–300 °C) and the degradation of Si–OH groups (400–600 °C). The CA film had two pyrolysis stages, the first thermal degradation process occurred in the temperature range of 225–300 °C which resulted from the degradation of the carboxyl group. The second stage occurred when the temperature was higher than 650 °C, which was due to the depolymerisation of alginate polymer and formation of a carbonaceous residue. The MIP showed less weight loss than CA film, indicating that the thermo stability was improved by incorporating the polysiloxane.
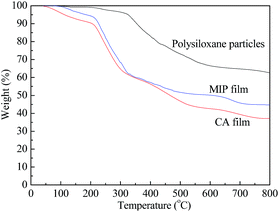 |
| Fig. 4 TG curves of polysiloxane particles, CA and MIP film. | |
The water contact angles of CA and MIP films prepared with different kinds of silanes were shown in Fig. 5. The structure of KH550 and KH570 silane was inserted in Fig. 5 (c) and (d). It is found that the water contact angle of CA and MIP film prepared with KH550 + KH570 was 38.5° and 67.5°, respectively. The hydrophobic alkyl group in the polysiloxane increased the water contact angle of MIP. Water contact angle of MIP prepared using only KH570 reached 124.6°. By contrast, water contact angle of MIP prepared using only KH550 was 10.3°. These results demonstrate that the surface hydrophilicity of the MIP film can be adjusted by changing the ratio of two types of silane.
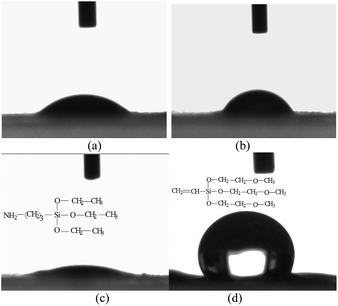 |
| Fig. 5 Water contact angle of CA (a) and MIP films prepared with (b) KH550 + KH570, (c) KH550 and (d) KH570. | |
Swelling behaviours of CA and MIP films in PBS solution are shown in Fig. 6. The CA film fell apart after swelling for 20 minutes in PBS solution because the cations in PBS solution could exchange calcium ions in calcium alginate hydrogel, which would destroy the cross-linking structure of the hydrogel. The MIP survived in PBS solution and the swelling ratio was kept stable for about 60 min. The polysiloxane on the surface of the MIP can effectively restrict the swelling of CA hydrogel, especially for MIP film prepared using only KH570. The swelling behaviour of MIP can be controlled by changing the experimental conditions, such as the ratio of two kinds of silane, the thickness of the CA film, and the temperature.
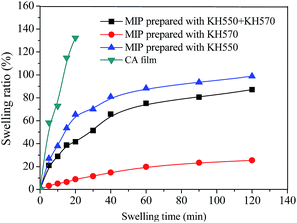 |
| Fig. 6 Swelling of CA and MIP films in PBS solution. | |
We then investigated the mechanical properties of the CA and MIP film as shown in Fig. 7. The strength of MIP film was higher than that of CA film because of the interactions between the polysiloxane and alginate. Kurayama et al.27 prepared microcapsules with 3-aminopropyltriethoxysilane/alginate hybrid shell based on sol–gel processes. According to the literature, KH-550 molecules can diffuse to alginate and adsorb on alginate shells via electrostatic interaction between protonated amino groups of KH-550 and carboxyl groups of alginate. Then self-condensation of KH-550 and KH-570 occurred to form polysiloxane particles on the surface of alginate, which further increased the crosslinking degree of alginate to lead to higher mechanical properties, especially modulus.
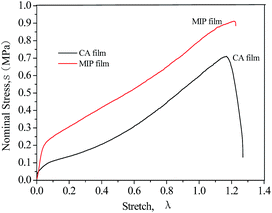 |
| Fig. 7 Stress–strain curves of CA film and MIP film. CA and MIP film were prepared with 3.0 wt% SA solution. | |
3.2 Adsorption of Hb on MIP prepared with different SA concentrations
Fig. 8 shows the adsorption capacity and IE of MIP prepared with different SA concentrations. The adsorption capacities of MIP and NIP increased when increasing SA concentration from 1.5 to 3.5 wt%. More polysiloxane was formed on the surface of CA film prepared with higher SA concentration. The MIP adsorbed more protein than NIP did. The concentration of calcium chloride had no effect on the adsorption capacity of MIP and NIP films. Considering the mechanical performance of CA film and the viscosity of SA solution, 3.0 wt% SA was used for the preparation of CA and MIP films in subsequent experiments.
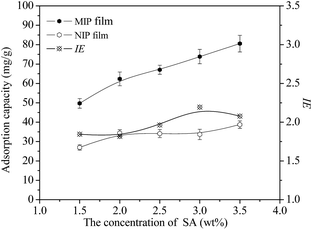 |
| Fig. 8 Adsorption capacity and IE of MIP using CA prepared with different SA concentrations. | |
3.3 Adsorption of Hb on MIP prepared with different silane amount
Fig. 9 shows the adsorption capacity and IE of MIP prepared with different silane amount. The Qe and IE of MIP increased initially and then decreased with the increase of silanes amount. When the amount of silane was 30 μL, the Qe reached the maximum value of 70.43 mg g−1. However, when the amount of silane was 20 μL, the IE of MIP reached the maximum value (2.36). For low amount of silane, protein imprinted polysiloxane on the surface of CA film was few and the Qe and IE of MIP were also low. Increasing the amount of silanes (>30 μL), the template protein were anchored more rigidly on the matrix, which made it difficult for the absolute removal of the templates and resulted in the decrease of Qe and IE. Our results are consistent with that reported in literature. Shiomi et al.21 synthesized Hb imprinted polysiloxane on silica using 3-aminopropyl-trimethoxysilane and trimethoxypropylsilane as functional monomers. They found that not only the silane amount but also the relative proportions played an important role in protein imprinting. The quantities of both removed template Hb and readsorbed Hb decreased proportionally when the amount of polymerized organosilane was increased. Template Hb thus appeared to be more rigidly anchored on base silica when more organosilanes were polymerized, inducing complementary protein–polymer interactions.
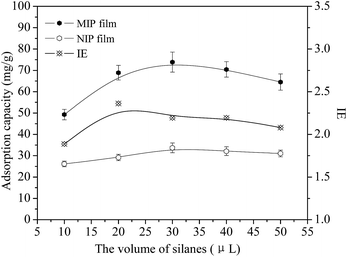 |
| Fig. 9 Adsorption capacity and IE of MIP using CA prepared with different silane concentrations. | |
3.4 Adsorption kinetics of Hb on MIP and NIP
Fig. 10 shows the dynamic adsorption curves of MIP and NIP. It is found that the adsorption rate was fast and the adsorption levels reached 80–85% of the equilibrium adsorption capacities for both MIP and NIP within 3.3 h. The adsorption rate of molecularly imprinted polysiloxane was much faster than that of the molecularly imprinted hydrogel.19,21,22 The stable adsorption capacity of MIP was 2.33 times higher than that of NIP after 5.5 h, with a value of 74.21 mg g−1. The higher adsorption capacity was attributed to the complex and complementary cavities between Hb molecules and the MIP. Because the imprinting cavities and the sites were on the surface of the MIP and macromolecular protein could spread more easily, the MIP had a fast adsorption rate.
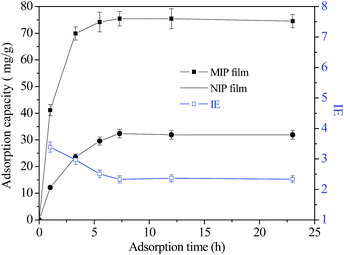 |
| Fig. 10 Adsorption dynamic of Hb on MIP and NIP. | |
3.5 Adsorption thermodynamics of Hb on MIPs and NIPs
The adsorption thermodynamics of Hb on MIP and NIP are displayed in Fig. 11. It is found that the adsorption capacity increased rapidly at first and gradually tended to equilibrium with the rise of initial Hb concentration. The equilibrium adsorption capacity of Hb on MIP was over 2.3 times as much as that of NIP. At low Hb concentrations, the amount of Hb was not enough to fill up the specific binding pits and cavities, so the equilibrium adsorption capacity increased with the increase of Hb concentration. When almost all specific imprinted sites were gradually occupied, the adsorption capacity of MIP became steady. The saturation value was achieved at a Hb concentration of 1.34 mg mL−1, which was the concentration for the preparation of MIP.
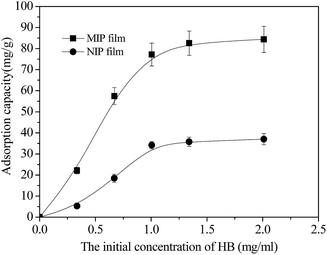 |
| Fig. 11 Curve of adsorption thermodynamics of MIP and NIP. | |
3.6 Specific adsorption of Hb on MIP and NIP
Table 1 shows the adsorption capacities of MIP and NIP for Hb and competitive proteins BSA, OVA, and Glo. It is found that the Hb imprinted MIP exhibited good adsorption selectivity for the template Hb. The adsorption capacity of Hb on MIP was above 4 times as much as those of BSA, OVA and Glo. A mixed solution of four proteins (Hb, BSA, OVA and Glo) was used for the competitive protein rebinding study. Fig. 12 shows the selectivity of the MIP film analyzed by SDS-PAGE. The lanes for Hb dispersed a little more than the competitive proteins, indicating that the MIP had high selectivity toward the template protein. Hb was adsorbed by MIP film irrespective of BSA, Ova, and Glo. The lanes for the protein mixtures did not disperse after adsorbed by NIP film, which confirmed that the NIP film showed no specific binding capability toward any protein.
Table 1 Adsorption capacity of MIP and NIP for Hb, Ova, BSA and Glo
Protein properties |
Qe(MIP) |
Qe(NIP) |
|
Mw (kDa) |
pI |
MIP |
NIP |
Ova |
43 |
4.7 |
18.65 ± 0.93 |
17.54 ± 0.88 |
Hb |
64 |
6.9 |
74.21 ± 3.71 |
31.65 ± 1.51 |
BSA |
67 |
4.9 |
16.48 ± 0.82 |
17.02 ± 0.84 |
Glo |
160 |
7.1 |
8.27 ± 0.35 |
7.84 ± 0.35 |
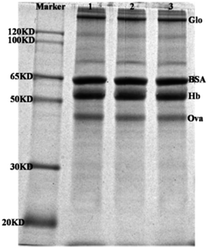 |
| Fig. 12 Gel electrophoresis analysis of protein. Lane 1, protein mixture containing 1 mg mL−1 Ova, Hb, BSA and Glo. Lane 2 protein mixture after adsorbed by NIP. Lane 3 protein mixture after adsorbed by MIP. | |
Higher Hb adsorption capacity of MIP is attributed to the generation of high-affinity Hb adsorption sites and the complementary cavities in the sol–gel reaction.21 The recognition sites of Hb were not complementary to other competitive proteins, such as BSA, OVA, and Glo. Thus, the competitive proteins had less chance to be adsorbed on the MIP. By comparison, NIP adsorbed much less Hb than MIP because there was no specific recognition site in NIP prepared in the absence of template protein.
3.7 Release of Hb from MIP and NIP films
After absorption of Hb, the MIP and NIP films were put into phosphate buffer solution (PBS) and Tris–HCl solution to study the release modes of Hb in a sterile incubator. Fig. 13 shows the Hb release profiles from MIP and NIP films in PBS solution. It was observed that 76.53% of Hb was released from the NIP film within 12 h, while the release of Hb from the MIP film was only about 26.53% after 12 h. The Hb release rate from the MIP film was significantly slower than that from the NIP film due to stronger interaction between Hb molecules and MIP. The cumulative release amount of Hb from NIP and MIP after 120 h was approximately 87.33% and 59.79% respectively. Fig. 14 shows Hb release profiles from MIP and NIP films in Tris–HCl solution. It was found that 57.05% of the Hb was released from the NIP film within 12 h, while the release of Hb from the MIP film was only 17.00% after 12 h. The Hb release rate from the MIP film was also slower than that from the NIP film. The cumulative release amount of Hb from NIP and MIP after 120 h in Tris–HCl solution was approximately 70.47% and 46.72% respectively. The release rate and amount of Hb in PBS were greater than those in Tris–HCl solution. This is because the ions in PBS can destroy the electrostatic force and the ionic interaction between protein and the film. In addition, the phosphate ions in PBS can react with the calcium ions and reduce the cross linking degree of calcium alginate hydrogel, which resulted in an increased swelling of the hydrogel film. The swelling behaviour can accelerate the release rate greatly for both MIP and NIP film.
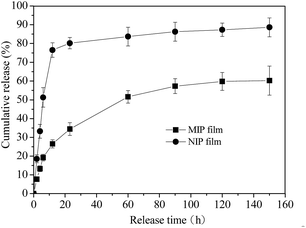 |
| Fig. 13 Hb release profiles from MIP and NIP films in PBS (pH 6.8, 20 °C) (n = 3). | |
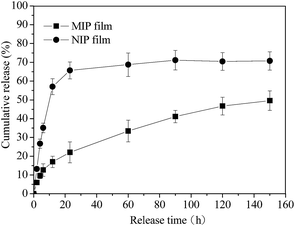 |
| Fig. 14 Hb release profiles from MIP and NIP films in Tris–HCl (pH 7.4, 20 °C) (n = 3). | |
The protein release kinetics showed a two-step release pattern with an initial burst release within 4 h and a relatively slow sustained release after 6 h. There was some nonspecific adsorption of Hb on the surface of MIP, and these proteins were easily released, causing the initial burst release. The specific adsorption of Hb had a relatively controlled and sustained release.
Kinetic models are applied to describe the mechanism of the release process of Hb. The correlation coefficients (R2) and release rates in different buffer solutions are shown in Table 2. The release rates of MIP film are much smaller than those of NIP film, showing better sustained release efficiency. According to the correlation coefficient, Higuchi model fits best for the MIP film no matter in PBS or in Tris–HCl solution. It is a diffusion-controlled release mode for MIP film. But for NIP film, in PBS solution, first-order model is the most convenient model, indicating that the release of Hb depends on its concentration. When it comes to Tris–HCl solution, Higuchi model fits better. The kinetic models could not appropriately describe the release processes for NIP film, because the correlation coefficient values are all under 0.8. One possibility is that diffusion is not the key factor for release of NIP film.
Table 2 Interpretation of the release experiments using different models
|
Film |
Zero-order model |
First-order model |
Higuchi model |
|
|
R2 |
Kd |
R2 |
Kd |
R2 |
Kd |
|
|
|
MIP |
0.8190 |
0.3759 |
0.9333 |
4 × 10−5 |
0.9561 |
4.753 |
|
|
PBS |
NIP |
0.5015 |
0.4185 |
0.7838 |
9 × 10−5 |
0.7069 |
26.02 |
|
|
Tris- |
MIP |
0.9166 |
0.3075 |
0.9701 |
12 × 10−5 |
0.9955 |
1.529 |
|
|
HCl |
NIP |
0.5325 |
0.3553 |
0.6812 |
4 × 10−5 |
0.7405 |
18.74 |
|
|
4. Conclusions
Haemoglobin (Hb) molecularly imprinted polysiloxane (MIP) was synthesized by sol–gel technology, using silanes as the functional monomers, Hb as the template and calcium alginate (CA) hydrogel film as the matrix in an aqueous phase. SEM results showed that Hb imprinted polysiloxane nanoparticles with the diameter of 50–400 nm were coated on the MIP. The mechanical strength of MIP film was higher than that of CA film because there were stronger interactions between the polysiloxane and alginate. Surface hydrophilicity and swelling behaviour of the MIP film can be adjusted by changing the ratio of two kinds of silanes.
The stable adsorption capacity of MIP was 2.33 times as much as that of NIP after 5.5 h, with a value of 74.21 mg g−1. Hb imprinted polysiloxane could recognize the template protein. The release rate from MIP film was significantly slower than that from NIP film due to the stronger interaction between Hb and the MIP. The cumulative release amount of Hb for NIP and MIP in PBS solution after 120 h was approximately 87.33% and 59.79% respectively. The release rate and amount of Hb in PBS were greater than those in Tris–HCl solution. Higuchi model fits best for the MIP film no matter in PBS or in Tris–HCl solution. It is a diffusion-controlled release mechanism for MIP film. And it is possible that diffusion is not the key factor for release of NIP film.
Acknowledgements
The research is supported by National Natural Science Foundation of China (51103102, 51103180, 81100100), fund of education ministry doctoral new teacher (20111201120004).
References
- C. M. Mary and N. A. Peppas, Adv. Drug Delivery Rev., 2009, 61, 1391 CrossRef PubMed
. - C. He, S. W. Kim and D. S. Lee, J. Controlled Release, 2008, 127, 189 CrossRef CAS PubMed
. - S. D. Li, B. H. Kan, K. Y. Zhao, T. Ren, B. B. Lin, J. F. Wei and T. Chen, Polym. Compos., 2014 DOI:10.1002/pc.23098
. - J. Wen, S. M. Anderson, J. Du, M. Yan, J. Wang, M. Shen, Y. Lu and T. Segura, Adv. Mater., 2011, 23, 4549 CrossRef CAS PubMed
. - Z. Zhang, L. X. Chen, F. F. Yang and J. H. Li, RSC Adv., 2014, 4, 31507 RSC
. - A. Mirmohseni, M. Shojaei and R. Pourata, RSC Adv., 2014, 4, 20177 RSC
. - M. E. Byrne, K. Park and N. A. Peppas, Adv. Drug Delivery Rev., 2002, 54, 149–161 CrossRef CAS
. - C. F. Nostrum, Drug Discovery Today, 2005, 119–124 CrossRef PubMed
. - D. Cunliffe, A. Kirby and C. Alexander, Adv. Drug Delivery Rev., 2005, 57, 1836 CAS
. - A. L. Carmen, Y. Fernando, B. I. Rafael and A. Concheiro, J. Controlled Release, 2006, 113, 236 CrossRef PubMed
. - C. L. Bayer, E. P. Herrero and A. P. Nicholas, J. Biomater. Sci., 2011, 22, 1523 CrossRef CAS PubMed
. - W. S. Cai and B. G. Ram, Sep. Purif. Technol., 2004, 35, 215 CrossRef CAS
. - C. Y. Wang, A. Javadi, M. Ghaffari and S. Q. Gong, Biomaterials, 2010, 31, 4944 CrossRef CAS PubMed
. - K. Y. Zhao, L. Z. Feng, H. Q. Lin, Y. F. Fu, B. B. Lin, W. K. Cui, S. D. Li and J. F. Wei, Catal. Today, 2014, 8, 127 CrossRef PubMed
. - L. Z. Feng, B. H. Kan, K. Y. Zhao, J. F. Wei, D. W. Zhu and L. H. Zhang, J. Sol-Gel Sci. Technol., 2014, 71, 428 CrossRef CAS PubMed
. - K. Y. Zhao, B. B. Lin, W. K. Cui, L. Z. Feng, T. Chen and J. F. Wei, Talanta, 2014, 121, 256 CrossRef CAS PubMed
. - K. Y. Zhao, G. X. Cheng, J. J. Huang and X. G. Ying, React. Funct. Polym., 2008, 68(3), 732 CrossRef CAS PubMed
. - B. H. Kan, B. B. Lin, K. Y. Zhao, X. X. Zhang, L. Z. Feng, J. F. Wei and Y. C. Fan, RSC Adv., 2014, 4(99), 55846 RSC
. - M. Glad, W. O. Norrlo, B. Sellergren, N. Siegbahn and K. Mosbach, J. Chromatogr., 1985, 347, 11 CrossRef CAS
. - K. Fukazawa, Q. Li, S. Seeger and K. Ishihara, Biosens. Bioelectron., 2013, 40, 96 CrossRef CAS PubMed
. - T. Shiomi, M. Matsui, F. Mizukami and K. Sakaguchi, Biomaterials, 2005, 26, 5564 CrossRef CAS PubMed
. - H. H. Tonnesen and J. Karlsen, Drug Dev. Ind. Pharm., 2002, 28, 621 CrossRef CAS PubMed
. - A. Shilpa, S. S. Agrawal and A. R. Ray, J. Macromol. Sci., Part C: Polym. Rev., 2003, 43, 187 CrossRef PubMed
. - K. Y. Zhao, J. J. Huang, X. G. Ying and G. X. Cheng, J. Appl. Polym. Sci., 2008, 109, 2687 CrossRef CAS
. - K. Y. Zhao, J. F. Wei, G. X. Cheng, C. X. Yang and L. Chen, J. Appl. Polym. Sci., 2009, 113, 1133 CrossRef CAS
. - G. Q. Pan, Q. P. Guo, C. B. Cao, H. L. Yang and B. Li, Soft Matter, 2013, 9, 3840 RSC
. - F. Kurayama, S. Suzuki, T. Oyamada, T. Furusawa, M. Sato and N. Suzuki, J. Colloid Interface Sci., 2010, 349, 70 CrossRef CAS PubMed
.
|
This journal is © The Royal Society of Chemistry 2015 |