DOI:
10.1039/C5RA03564C
(Paper)
RSC Adv., 2015,
5, 38995-39002
Facile fabrication of a novel nanoporous Au/AgO composite for electrochemical double-layer capacitor†
Received
27th February 2015
, Accepted 15th April 2015
First published on 15th April 2015
Abstract
In this work, a novel nanoporous gold/silver oxide (NP Au/AgO) composite (NPAAC) was fabricated by a simple two-step partial dealloying–stripping process. Microstructural and electrochemical characterizations suggested that the NPAAC has a bicontinuous, interpenetrating nanoporous structure with ultrafine ligament–channel sizes down to ∼10 nm, and it delivered a rectangle cyclic voltammetry (CV) curve with a wide electrochemical double-layer region from −0.3 to 0.7 V (vs. Ag/AgCl in sulfuric acid) and a specific capacitance of ∼80 F g−1. Moreover, the NPAAC revealed a superior cyclic performance as compared to metal-based pseudocapacitors, with stable charge–discharge cycling at ∼77 F g−1 for more than ten thousand times with no detectable degradation. This work demonstrates that the new systems of nanoporous noble metal/metal-oxide composites could be similarly fabricated by this approach and developed as potential double-layer capacitors with comparable cyclic performance to carbon-based supercapacitors.
Introduction
Electrochemical supercapacitors have attracted tremendous attention in the field of materials research due to their high power density, fast charging–discharging rate, and long operation lifetimes.1–7 Recently, various nanomaterials such as transition metal oxides8,9 and conductive polymer nanostructures have been explored for use in supercapacitor applications.10,11 Generally supercapacitors can be categorized into two major types: electrochemical double-layer capacitors, which store energy by ion adsorption and desorption on the diffuse double layer, and pseudocapacitors, which store energy via surface redox reaction between the electrodes and the electroactive species in the electrolyte. Tremendous research efforts had been focused on developing pseudocapacitors based on various nanostructured metal/metal oxide materials,12 such as in a recent work by Lang et al. in which a pseudo-hybrid (gold/MnO2) nanostructure was fabricated and demonstrated a very high capacitance due to the enhanced conductivity from the porous nanostructure.1 For double-layer supercapacitors, existing electrode materials were mainly based on carbon nanomaterials.13,14 On the other hand, it is well known that the electrochemical double-layer also widely exists in noble metals such as Pt,15 Pd16 and Au,17 and earlier reports also suggested that the enhancement of the porosity of the noble metals could significantly enhance their electrical capacity.7,26 Recently, the so-called “dealloying” technique has been successfully developed for the rational fabrication of various porous metal nanostructures with high efficiency and low cost20–25 and provide a great opportunity for the development of nanoporous metals-based supercapacitors. Due to its enhanced conductivity as a substrate, nanoporous gold has been already used in several supercapacitors based on metal oxides and conductive polymers.1,2,18,19
Despite the great performance of metal/metal-oxide based nanocomposites in novel supercapacitor applications (such as shown by Lang et al.1), the fabrication of such porous metallic nanocomposite structures generally remains quite complicated and costly. Here, we report the facile fabrication of a nanoporous Au/AgO composite (NPAAC) for possible use in double-layered electrochemical capacitor applications. Fabrication was achieved by a simple two-step method involving partial electrochemical dealloying and subsequent stripping of Ag–Au alloy in sulfuric acid solution. The novel metal/metal oxide nanocomposite delivered a rectangular CV curve with a wide electromechanical double-layer region, as well as an outstanding cycling performance with stable charge–discharge over ten thousand cycles.
Results
The newly developed two-step fabrication process, as illustrated in Fig. 1, involved incomplete electrochemical dealloying and subsequent stripping of Ag–Au alloy. In the partial electrochemical dealloying step, when we applied a 1.8 V potential to the Ag–Au alloy, the oxygen atoms gathered on the surface of the alloy and reacted with surface silver atoms, as shown in Fig. 1a and b. After the surface silver atoms were oxidized, the penetration of oxygen atoms in alloy and surface diffusion of silver atoms occurred. Moreover, the surface diffusion of gold atoms may also occur but in a slight degree because of the natural inertness characteristic of gold. Thus, nanoscale pores and channels were generated on the surface of the Ag–Au alloy, providing a pathway for the surface diffusion and oxidation of silver atoms. As the process continued, Au atoms were electrochemically driven to diffuse and agglomerate to form a ligament–channel structure. The produced AgO nanostructure aggregated on the surface to form the floccule through the internal channels with small parts of AgO embedded in the matrix, as shown in Fig. 1c and d. Finally, as the ligament–channel structure propagated, the nanoporous structure was formed uniformly in the matrix, resulting in the floccule-covered NPAAC. It is important to point out that at this moment the surface of NPAAC is still tightly covered by the AgO floccule. In the next step, we further applied a 0 V potential on the floccule-covered NPAAC for 1 min to strip off the covered AgO floccule, as schematically shown in Fig. 1e and f. Despite some of the AgO floccule embedding in the nanoporous structure, most of them covered the surface of NPAAC, which blocked the pores and impeded the ion transportation. Due to its poor adhesion, the fragile AgO floccule could be easily striped off at the 0 voltage under the pounding of massive hydrogen bubbles generated simultaneously. Hence, we define this process as “electrochemical stripping”. Finally, we obtained high quality NPAAC specimens for follow-up characterization.
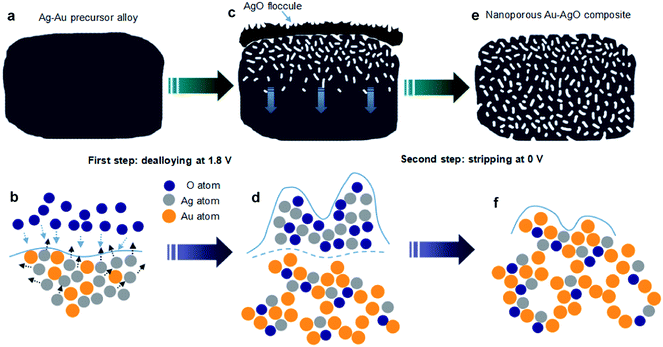 |
| Fig. 1 Schematic diagram for the fabrication process of the nanoporous Au/AgO composite (NPAAC). | |
In order to understand the mechanisms of the reactions during the two steps, microstructure characterizations of NPAAC were carried out at first. Fig. 2a shows a SEM image of Ag–Au alloy without any electrochemical treatment. It can be seen that there were few casting flaws on the flat surface. After dealloying, it can be observed that the surface was covered with dense floccule, as shown in Fig. 2b. It should be noted that the floccule was tightly attached to the matrix and was not precipitated into solution before the electrochemical reaction. As hydrogen atoms aggregated on the interface between the AgO floccule and the matrix with the application of 0 V potential on the working electrode, the AgO floccule stripped off from the surface and the matrix turned into a uniform nanoporous structure, as demonstrated in Fig. 2c. It is a typical bicontinuous interpenetrating nanoporous structure with an average ligament–channel size of ∼10 nm. Note that the size of the nanoporous structure was considerably smaller than nanoporous gold fabricated by directly chemically dealloying Ag–Au alloy in nitric acid.1,2 The SAED pattern (Fig. 2d) verified that the NPAAC was constituted of polycrystalline Au and AgO. In order to analyze the chemical composition and further study the mechanism of NPAAC, XPS was carried out to investigate the elemental composition at different steps (ESI†).
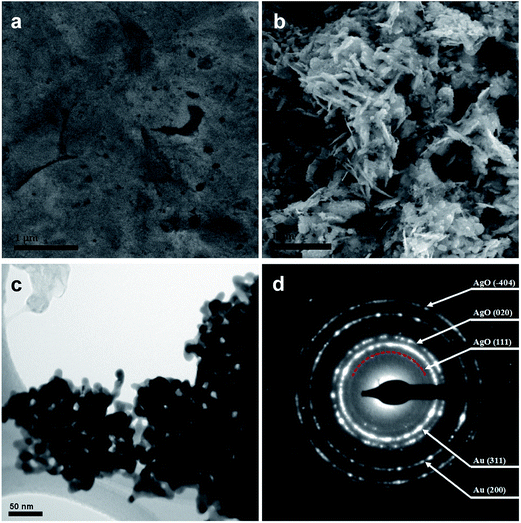 |
| Fig. 2 SEM images of (a) the original Ag–Au alloy and (b) the sample after dealloying (both scale bars show 1 μm). (c) TEM images of the nanoporous-structured NPAAC after stripping. (d) SAED pattern of the Au (200), (311) and AgO (111), (020), and (−404). | |
Before assessing the capacitance performance of the NPAAC, the electrochemical window was revealed by expanding the CV scanning potential region from (0.1 V, 0.3 V) to (−0.4, 0.8 V) with an interval of 0.1 V, as shown in Fig. 3. A strong oxygen adsorption peak emerged when the applied potential was increased to 0.8 V during the positive scan. A corresponding oxygen desorption peak evident appeared in the subsequent negative scan. A similar scenario was observed when the applied potential was reduced to −0.4 V. It is evident that a couple of hydrogen adsorption/desorption peaks appeared with the negative scan beyond −0.3 V. The strong adsorption/desorption of oxygen and hydrogen induced by applied potentials can be regarded as an over-potential redox reaction.27–29 Therefore, this suggested that the effective electrochemical region of NPAAC should be around −0.3 to 0.7 V. As AgO occurs as a reversible redox reaction,12,30 the rectangular CV curves can be attributed to the joint contribution of AgO and Au to the capacitance of NPAAC, which is a feature of a double-layer supercapacitor.
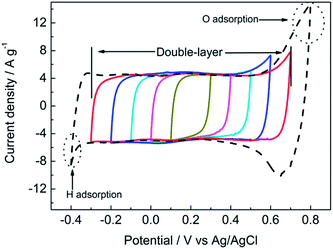 |
| Fig. 3 CV curves of the nanoporous Au/AgO composites (NPAAC) at a scan rate of 50 mV s−1 with extension of the potential region from (0.1 V, 0.3 V) to (−0.4 V, 0.8 V) and an interval of 0.1 V (electrochemical double-layer region shown in the center with the hydrogen (H) adsorption region and oxygen (O) adsorption region shown in the circled areas). | |
The alloying effect on the formation of NPAAC in comparison to pure silver and pure gold was studied. In order to identify the derivation of the capacitance and the impact of the component effect, we treated pure gold sheet and silver sheet with the same method used to produce NPAAC. Then, electrochemical performance of the formed nanoporous gold and silver was studied in the same electrochemical window (from −0.3 to 0.7 V). The formation mechanism can be explained based on the anodization–reduction model,31 in which the metal atoms will combine with oxygen to form metal oxide under a high potential, and the oxidation product will be reduced to a metallic state or strip off from the matrix subsequently. Fig. 4a and c show typical bicontinuous ligament–channel microstructures of nanoporous gold and silver fabricated by a two-step method. The ligament–channel sizes of nanoporous gold and nanoporous silver were ∼70 nm and ∼400 nm, respectively. Au atoms have a lower surface diffusion rate compared to Ag atoms, which will have a significant rate-limiting effect on the mobility of Au atoms. The finer nanoporous structure can thus be formed as compared with nanoporous silver.32 Fig. 4b illustrates the CV curve of nanoporous Au tested in sulfuric acid solution within a scan potential region of −0.3 to 0.7 V. A strong hydrogen adsorption effect appeared when the applied potential was below 0.2 V, which is commonly observed in CVs of Au nanostructures tested in sulfuric acid.33,34 As shown in Fig. 4d, the CV curve of nanoporous Ag exhibited a typical redox reaction peak.35
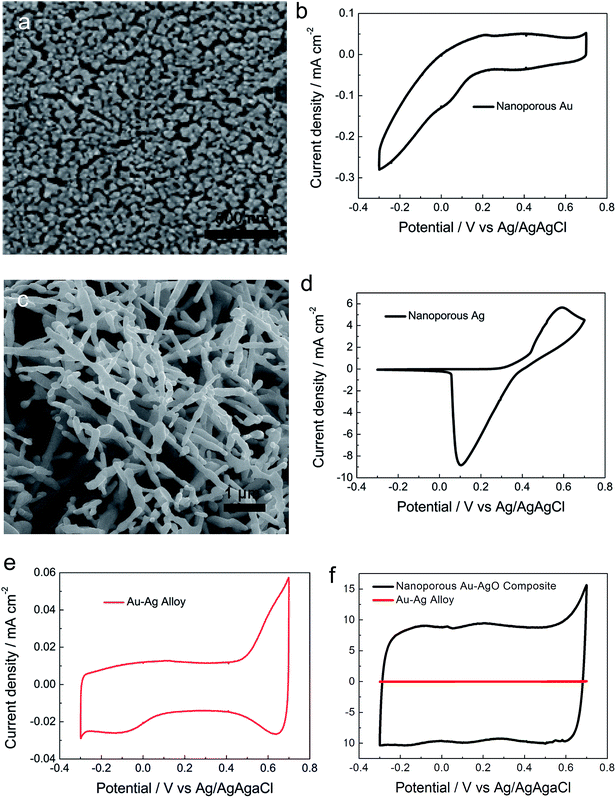 |
| Fig. 4 (a and b) SEM image of nanoporous Au obtained by treating with pure gold sheet and the corresponding CV curve. (c and d) SEM image of nanoporous Ag obtained by treating with pure silver sheet and the corresponding CV curve. (e) CV curve of the Ag–Au alloy and (f) CV curve of the NPAAC for comparison (Y-axis is also in current density/mA cm−2). All data are taken at a scan rate of 50 mV s−1. | |
As the electrochemical performances of nanoporous Au and Ag were fabricated by the same method, the formation of the unique electrochemical double-layer region cannot be attributed to any single component (Au or AgO) of NPAAC. The outstanding electrochemical performance of NPAAC is attributed to the particular composition and the fine microstructure, which provides Au/AgO interfaces for enhanced electronic transport and also open-pore channels for sufficient contact between NPAAC and the electrolyte. Although the electrochemical performance cannot be directly measured due to its poor conductivity, the porous silver will generate Ag–O− or Ag–OH species during the cyclic voltammogram test.
In order to clearly analyze the origination of the CV regions of NPAAC, we also tested the Ag–Au alloy and NPAAC under the same conditions, with the corresponding CV curves shown in Fig. 4e and f, respectively. It should be noted that the red curve shown in Fig. 4f is actually the same curve shown in Fig. 4e (flattened due to scale difference). The dealloying process drove the compact Ag–Au alloy into the desired ultrafine nanoporous structure (as shown in Fig. 2c and ESI Fig. S3†), whereas the enormous difference in the current density was indeed caused by the significantly enhanced surface area of NPAAC. The oxygen adsorption/desorption peaks were mainly caused by the redox reaction of metallic silver in the Ag–Au alloy. The hydrogen adsorption was relatively weak compared to nanoporous gold when the potential was below 0.2 V. It can be caused by the interaction, i.e. alloying effect36 between Ag and Au in the Ag–Au alloy. Though an oxygen adsorption peak appeared at 0.7 V in the CV curve of NPAAC, the primary reaction still cannot be attributed to the formation of Au–O− or Au–OH monolayer because the applied potential was not big enough to drive the reaction and no desorption peak was observed. Thus, the oxygen adsorption peak can still be regarded as existing in the electrochemical reaction region and was caused by the ion adsorption. In addition, it can be suggested that the ion absorption ability can be enhanced with the formation of Au/AgO hybrid porous structure. Furthermore, an explosive growth of the double-layer was observed after the two-step treatment, as shown in Fig. 4f.
Discussion
The effect of scan rate on the cyclic voltammogram response of NPAAC was investigated over the range 10–70 mV s−1, as shown in Fig. 5a. It is important to note that the scan rate leads to further augmentation of the CV curve, indicating that the charge separation at the electrode/electrolyte interfaces were faster. Although the scan rate reaches 70 mV s−1, the cyclic voltammogram retained a rectangular shape and exhibited no resistive behavior. The specific capacitances, C, of the NPAAC can be calculated from the CV curves using the following equation: |
 | (1) |
where i is the current density (A), V is the potential (V), v is the scan rate (mV s−1), and m is the mass of the NPAAC. The results are illustrated in Fig. 5c (red curve). The capacitance decreased slightly with the increased scan rates. The specific capacitance was maintained at 72 F g−1 at a scan rate of 70 mV s−1, which is comparable to the capacitance 76 F g−1 at a scan rate of 10 mV s−1. Fig. 5b shows the galvanostatic charge–discharge profiles of NPAAC at different current densities. The linear symmetric charging and discharging profiles indicated that the capacitive behavior of NPAAC was reversible with fast charge transfer kinetics. The specific capacitance can be calculated from cyclic chronopotentiometric curves using the following equation: |
 | (2) |
where i is the discharge current, Δt is the discharge time, m is the mass of the NPAAC, and ΔV is the potential window. The results of specific capacitance values are illustrated in Fig. 5c (the blue curve). It can be seen that the specific capacitance decreased evidently with the increase of current density. The lowest capacitance was 72 F g−1 and the highest was about 83 F g−1, which is a general value for the supercapacitor.1,2,30 The big gap between the atomic mass of Au (or Ag) and other atoms (like C) is supposed to indicate that the specific capacitance value of NPAAC is smaller than that of other electrochemical capacitors such as carbon materials which ranges from 100 to 300 F g−1.26,37–40 In addition, the specific capacitance measured at 1 A g−1 was slightly larger than that measured at 10 mV s−1, mainly because of the slight influence of oxidation reactions which is hard to be avoided at high potential. The energy density (de) and power density (dp) can also be calculated according to the following eqn (3) and (4): |
 | (3) |
|
 | (4) |
where C is the specific capacitance, ΔV is the voltage change after a full charge or discharge, and Δt is the time for a full charge or discharge. As shown in the inset, an energy density of 11 W h kg−1 and a power density of 2500 W kg−1 was obtained at a charge–discharge rate of 5 A g−1. It is necessary to point out that the power density is comparable to typical electrochemical double-layer capacitors.30
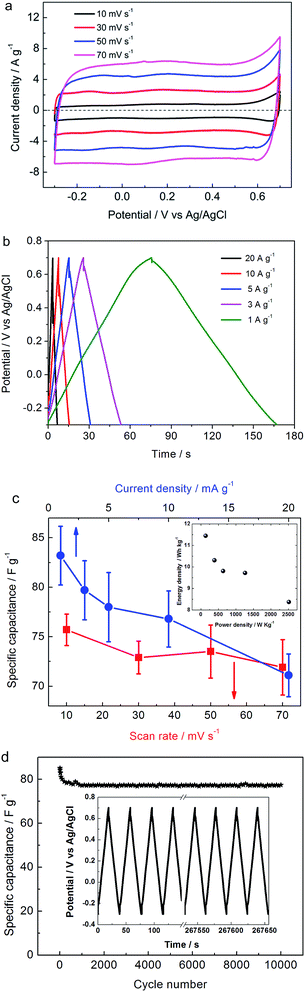 |
| Fig. 5 (a) CV curves of nanoporous Au/AgO composites (NPAAC) at four different scan rates between 10 and 70 mV s−1. (b) Charge–discharge curves of the NPAAC at five different current densities between 1 and 20 A g−1. (c) Corresponding specific capacitance of NPAAC versus scan rate and current density. Inset: Ragone plot of the NPAAC. (d) Cycling performance of NPAAC at a current density of 5 A g−1. Inset: charge–discharge profile of the NPAAC at a current density of 5 A g−1. | |
Lastly, cycling performance is another important parameter for any potential supercapacitor applications. For carbon-based double-layer supercapacitors, the specific capacitance can be maintained for thousands charge–discharge cycles, which is far better than most existing metal oxides-based pseudocapacitors. In this work, the cycling stability of the NPAAC was also examined over ten thousands of charge–discharge cycles at a current density of 5 A g−1, as shown in Fig. 5d. At the beginning of the cycles, the specific capacitance dropped slightly from about 82 F g−1 to 77 F g−1. This activation process was derived from the complete adsorption and desorption of electrochemical species after the initial cycles. After that, the specific capacitance values remained stable until the end of the cycling. In comparison with most metal oxides-based pseudocapacitors, in which the cycling performance is maintained only for hundreds or thousands cycles, the NPAAC revealed a considerably more outstanding cycling performance with stable charge–discharge characterization over ten thousand cycles. This fact can be also confirmed by the charge–discharge profile of the NPAAC shown in the inset of Fig. 5d and can be mainly attributed to the nature of electrochemical double-layer mechanism.
Conclusions
In summary, we successfully fabricated a novel nanoporous Au/AgO composite (NPAAC) with ligament–channel sizes of ∼10 nm by a newly developed electrochemical partial dealloying–stripping method. The NPAAC demonstrated an electrochemical window with a wide double-layer from −0.3 to 0.7 V vs. Ag/AgCl, and delivered a comparable specific capacitance of about 77 F g−1 at a current density of 5 A g−1. More importantly, the ultrafine NPAAC displayed a superior stable charge–discharge cyclic performance, which could make nanoporous noble metal/metal-oxide composites another promising candidate for supercapacitor applications.
Methods
The fabrication of NPAAC, nanoporous gold and nanoporous silver
Free-standing Ag–Au alloy (Ag66Au34), Au and Ag sheets were ultrasonically cleaned in acetone, ethanol and deionized water (DI) in sequence. The area of the working electrode (WE) was about 1 cm−2. All electrochemical treatments and measurements were performed in a standard three-electrode electrochemical workstation (CHI 760E) with a plate-like Pt as the counter electrode (CE) and a Ag/AgCl electrode as the reference electrode (RE) in 0.5 M H2SO4. A potential of 1.8 V vs. Ag/AgCl for 20 min was applied to all specimens in the dealloying stage, and then a potential 0 V vs. Ag/AgCl was applied for 1 min in the stripping stage.
Microstructure characterization
The microstructure analysis of specimens was conducted using a field-emission scanning electron microscope (Philips XL-30, 15 keV) and a field-emission transmission electron microscope (CM 20 FEG, 200 kV), assisted with image processing and analyzing software. The chemical states and components of gold, silver and oxygen in NPAAC were revealed by a X-ray photoelectron spectrometer (XPS, VG ESCALAB 220i-XL) equipped with a monochromatic Al Kα (1486.6 eV) X-ray source.
Electrochemical characterization
The applied potentials (1.8 and 0 V) were determined by positive-going linear sweep voltammetry (LSV) curves at a scan rate of 50 mV s−1. CV and galvanostatic charge–discharge tests were used to characterize the electrochemical behavior of the NPAAC. The CV curves were measured in a potential range between −0.3 and 0.7 V vs. Ag/AgCl at different scan rates, and the charge–discharge cycling performance were evaluated between the potential of −0.3 and 0.7 V at different current densities. The cycling stabilities were assessed under a constant current density of 5 A g−1 for over 10
000 cycles.
Acknowledgements
Y. Lu gratefully acknowledges the funding support from the National Natural Science Foundation of China (Grant no. 51301147), the Research Grants Council of the Hong Kong Special Administrative Region of China (GRF no. 11209914) and City University of Hong Kong (Project nos 7200339 and 9680108). W. Zhang acknowledges the funding support from the National Natural Science Foundation of China (Grant nos 51372213, and 61176007).
References
- X. Lang, A. Hirata, T. Fujita and M. Chen, Nanoporous Metal/Oxide Hybrid Electrodes for Electrochemical Supercapacitors, Nat. Nanotechnol., 2011, 6, 232–236 CrossRef CAS PubMed.
- F. Meng and Y. Ding, Sub-Micrometer-Thick All-Solid-State Supercapacitors with High Power and Energy Densities, Adv. Mater., 2011, 23, 4098–4102 CrossRef CAS PubMed.
- X. Xia, J. Tu, Y. Zhang, J. Chen, X. Wang, C. Gu, C. Guan, J. Luo and H. J. Fan, Porous Hydroxide Nanosheets on Preformed Nanowires by Electrodeposition: Branched Nanoarrays for Electrochemical Energy Storage, Chem. Mater., 2012, 24, 3793–3799 CrossRef CAS.
- L. Bao, J. Zang and X. Li, Flexible Zn2SnO4/MnO2 Core/Shell Nanocable-Carbon Microfiber Hybrid Composites for High-Performance Supercapacitor Electrodes, Nano Lett., 2011, 11, 1215–1220 CrossRef CAS PubMed.
- R. Liu, J. Duay and S. B. Lee, Redox Exchange Induced MnO2 Nanoparticle Enrichment in Poly(3,4-ethylenedioxythiophene) Nanowires for Electrochemical Energy Storage, ACS Nano, 2010, 4, 4299–4307 CrossRef CAS PubMed.
- S. Biswas and T. L. Drzal, Multilayered Nanoarchitecture of Graphene Nanosheets and Polypyrrole Nanowires for High Performance Supercapacitor Electrodes, Chem. Mater., 2010, 22, 5667–5671 CrossRef CAS.
- J. Lee, S. Yoon, T. Hyeon, S. M. Oh and K. B. Kim, Synthesis of a New Mesoporous Carbon and its Application to Electrochemical Double-Layer Capacitors, Chem. Commun., 1999, 2177–2178 RSC.
- Z. J. Fan, J. Yan, T. Wei, L. J. Zhi, G. Q. Ning, T. Y. Li and F. Wei, Asymmetric Supercapacitors Based on Graphene/MnO2 and Activated Carbon Nanofiber Electrodes with High Power and Energy Density, Adv. Funct. Mater., 2011, 21, 2366–2375 CrossRef CAS PubMed.
- J. H. Kim, K. Zhu, Y. F. Yan, C. L. Perkins and A. J. Frank, Microstructure and Pseudocapacitive Properties of Electrodes Constructed of Oriented NiO–TiO2 Nanotube Arrays, Nano Lett., 2010, 10, 4099–4104 CrossRef CAS PubMed.
- L. Nyholm, G. Nystrom, A. Mihranyan and M. Stromme, Toward Flexible Polymer and Paper-Based Energy Storage Devices, Adv. Mater., 2011, 23, 3751–3769 CAS.
- G. Nystrom, A. Razaq, M. Stromme, L. Nyholm and A. Mihranyan, Ultrafast All-Polymer Paper-Based Batteries, Nano Lett., 2009, 9, 3635–3639 CrossRef PubMed.
- Q. Lu, G. J. Chen and Q. J. Xiao, Nanostructured Electrodes for High-Performance Pseudocapacitors, Angew. Chem., Int. Ed., 2013, 52, 1882–1889 CrossRef CAS PubMed.
- D. Pech, M. Brunet, H. Durou, P. Huang, V. Mochalin, G. Yury, P.-L. Taberna and P. Simon, Ultrahigh-Power Micrometre-Sized Supercapacitors Based on Onion-Like Carbon, Nat. Nanotechnol., 2010, 5, 651–654 CrossRef CAS PubMed.
- J. Yan, Q. Wang, T. Wei and Z. Fan, Recent Advances in Design and Fabrication of Electrochemical Supercapacitors with High Energy Densities, Adv. Energy Mater., 2014, 4, 1300816 Search PubMed.
- J. Weissmüller, R. N. Viswanath, D. Kramer, P. Zimmer, R. Würschum and H. Gleiter, Charge-Induced Reversible Strain in a Metal, Science, 2003, 300, 312–315 CrossRef PubMed.
- X. Wang, W. Wang, Z. Qi, C. Zhao, J. Hong and Z. Zhang, High Catalytic Activity of Ultrafine Nanoporous Palladium for Electro-Oxidation of Methanol, Ethanol, and Formic Acid, Electrochem. Commun., 2009, 11, 1896–1899 CrossRef CAS PubMed.
- J. T. Zhang, P. P. Liu, H. Y. Ma and Y. Ding, Nanostructured Porous Gold for Methanol Electro-Oxidation, J. Phys. Chem. C, 2007, 111, 10382–10388 CAS.
- X. Y. Lang, L. Zhang, T. Fujita, Y. Ding and M. W. Chen, Three-Dimensional Bicontinuous Nanoporous Au/Polyaniline Hybrid Films for High-Performance Electrochemical Supercapacitors, J. Power Sources, 2012, 197, 325–329 CrossRef CAS PubMed.
- L. Y. Chen, Y. Hou, J. L. Kang, A. Hirata, T. Fujita and M. W. Chen, Toward the Theoretical Capacitance of RuO2 Reinforced by Highly Conductive Nanoporous Gold, Adv. Energy Mater., 2013, 3, 851–856 CrossRef CAS PubMed.
- Z. Zhang, Y. Wang, Z. Qi, W. Zhang, J. Qin and J. Frenzel, Generalized Fabrication of Nanoporous Metals (Au, Pd, Pt, Ag, and Cu) through Chemical Dealloying, J. Phys. Chem. C, 2009, 113, 12629–12636 CAS.
- A. J. Forty, Corrosion Micromorphology of Noble Metal Alloys and Depletion Gilding, Nature, 1979, 282, 597 CrossRef CAS.
- M. Raney, US Pat. no. 1563587, 1925.
- J. Xu, C. Zhang, X. Wang, H. Ji, C. Zhao, Y. Wang and Z. Zhang, Fabrication of Bi-Modal Nanoporous Bimetallic Pt–Au Alloy with Excellent Electrocatalytic Performance towards Formic Acid Oxidation, Green Chem., 2011, 13, 1914–1922 RSC.
- X. Yan, F. Meng, Y. Xie, J. Liu and Y. Ding, Direct N2H4/H2O2 Fuel Cells Powered by Nanoporous Gold Leaves, Sci. Rep., 2012, 2, 941 Search PubMed.
- H.-J. Jin, X.-L. Wang, S. Parida, K. Wang, M. Seo and J. Weissmüller, Nanoporous Au–Pt Alloys As Large Strain Electrochemical Actuators, Nano Lett., 2010, 10, 187–194 CrossRef CAS PubMed.
- M. Lazzari, F. Soavi and M. Mastragostino, Mesoporous Carbon Design for Ionic Liquid-Based, Double-Layer Supercapacitors, Fuel Cells, 2010, 10, 840–847 CrossRef CAS PubMed.
- S. Y. Qian, B. E. Conway and G. Jerkiewicz, Comparative Effects of Adsorbed S-species on H Sorption into Pd from UPD and OPD H: a Kinetic Analysis, Int. J. Hydrogen Energy, 2000, 25, 539–550 CrossRef CAS.
- V. S. Bagotzky and M. R. Tarasevich, General Expression of the Linear Potential Sweep Voltammogram in the Case of Diffusionless Electrochemical Systems, J. Electroanal. Chem., 1979, 101, 1–17 CrossRef.
- M. Grdeń, M. Łukaszewski, G. Jerkiewicz and A. Czerwiński, Electrochemical behaviour of palladium electrode: oxidation, electrodissolution and ionic adsorption, Electrochim. Acta, 2008, 53, 7583–7598 CrossRef PubMed.
- P. Simon and Y. GoGotSi, Materials for Electrochemical Capacitors, Nat. Mater., 2008, 7, 845–854 CrossRef CAS PubMed.
- J. Sun, C. Zhang, T. Kou, J. Xu and Z. Zhang, Anodization of Pd in H2SO4 Solutions: Influence of Potential, Polarization Time, and Electrolyte Concentration, ACS Appl. Mater. Interfaces, 2012, 4, 6038–6045 CAS.
- J. Erlebacher, An Atomistic Description of Dealloying: Porosity Evolution, the Critical Potential, and Rate-Limiting Behavior, J. Electrochem. Soc., 2004, 151, C614–C626 CrossRef CAS PubMed.
- B. Plowman, S. J. Ippolito, V. Bansal, Y. M. Sabri, A. P. O'Mullane and S. K. Bhargava, Gold Nanospikes Formed through a Simple Electrochemical Route with High Electrocatalytic and Surface Enhanced Raman Scattering Activity, Chem. Commun., 2009, 5039–5041 RSC.
- D. A. J. Rand and R. Woods, Cyclic Voltammetric Studies on Iridium Electrodes in Sulphuric Acid Solutions: Nature of Oxygen Layer and Metal Dissolution, J. Electroanal. Chem. Interfacial Electrochem., 1974, 55, 375–381 CrossRef CAS.
- W. Zhang, C. Q. Tu, Y. F. Chen, W. Y. Li and G. Houlachi, Cyclic Voltammetric Studies of the Behavior of Lead–Silver Anodes in Zinc Electrolytes, J. Mater. Eng. Perform., 2013, 22, 1672–1679 CrossRef CAS.
- A. Dursun, D. V. Pugh and S. G. Corcoran, Dealloying of Ag–Au Alloys in Halide-Containing Electrolytes Affect on Critical Potential and Pore Size, J. Electrochem. Soc., 2003, 150, B355–B360 CrossRef CAS PubMed.
- J. H. Chen, W. Z. Li, D. Z. Wang, S. X. Yang, J. G. Wen and Z. F. Ren, Electrochemical Characterization of Carbon Nanotubes as Electrode in Electrochemical Double-Layer Capacitors, Carbon, 2002, 40, 1193–1197 CrossRef CAS.
- W. Lu and R. Hartman, Nanocomposite Electrodes for High-Performance Supercapacitors, J. Phys. Chem. Lett., 2011, 2, 655–660 CrossRef CAS.
- E. G. Bushueva, P. S. Galkin, A. V. Okotrub, L. G. Bulusheva, N. N. Gavrilov, V. L. Kuznetsov and S. I. Moiseekov, Double layer supercapacitor properties of onion-like carbon materials, Phys. Status Solidi B, 2008, 245, 2296–2299 CrossRef CAS PubMed.
- C. Portet, P. L. Taberna, P. Simon, E. Flahaut and C. Laberty-Robert, High power density electrodes for carbon supercapacitor applications, Electrochim. Acta, 2005, 50, 4174–4181 CrossRef CAS PubMed.
Footnotes |
† Electronic supplementary information (ESI) available. See DOI: 10.1039/c5ra03564c |
‡ Present address: Department of Electronic Engineering, The Chinese University of Hong Kong, Shatin, NT, Hong Kong, China. |
§ These authors contributed equally to this work. |
|
This journal is © The Royal Society of Chemistry 2015 |