DOI:
10.1039/C5RA03524D
(Paper)
RSC Adv., 2015,
5, 28487-28496
The role of cerium(III)/yttrium(III) nitrate hexahydrate salts on electroactive β phase nucleation and dielectric properties of poly(vinylidene fluoride) thin films
Received
26th February 2015
, Accepted 18th March 2015
First published on 18th March 2015
Abstract
Electroactive poly(vinylidene fluoride) (PVDF) thin films modified with Ce(NO3)3·6H2O and Y(NO3)3·6H2O (1–30 mass%) have been prepared via a simple solution casting method. The thermal stability and microstructures of the films were investigated using thermal gravimetric analysis techniques and field emission electron microscopy respectively. X-ray diffraction spectroscopy, Fourier transform infrared spectroscopy and differential scanning calorimetry confirmed the nucleation of the electroactive β phase in the composite films. Strong interfacial interaction i.e. ion dipole interaction via formation of hydrogen bonds between the water molecules of the salts and the polar –CF2 dipoles of the polymer chains resulted in improved electroactive β phase nucleation and a large dielectric constant in PVDF films.
1. Introduction
Recently, electroactive polymer thin films have gained tremendous attention from researchers worldwide due to their excellent piezoelectric, pyroelectric, ferroelectric, dielectric and electro-optic properties. High flexibility, simple fabrication processes and non-toxicity make these polymer films a suitable alternate for traditional ferroelectric ceramics.1–9
Poly(vinylidene fluoride) [PVDF] and its copolymers like poly(vinylidene fluoride-trifluoroethylene) [P(VDF-TrFE)], poly(vinylidene fluoride-hexafluoropropylene) [P(VDF-HFP)] etc. have been considered as prospective materials for versatile applications such as in sensors, actuators, non-volatile memories in microelectronics, rail runs, piezoelectric nanogenerator, thin film transistors, pulsed lasers, electric or hybrid vehicles, artificial muscles, charge-storage devices due to their inspiring piezoelectric, pyroelectric, dielectric and ferroelectric properties.1–10
Electroactive semicrystalline PVDF occurs in at least five polymorphs like α, β, γ, δ and ε. But α, β and γ polymorphs are most common crystalline phases of PVDF. Nonpolar α phase has TGTG′ (T-trans, G-gauche+, G′-gauche) dihedral conformation which is thermodynamically stable. Electroactive or polar β and γ phase have all trans (TTTT) and (TTTGTTTG′) conformation respectively.10,11 Among the polar β and γ phases, mainly β phase show promising dielectric, ferroelectric, piezoelectric and pyroelectric properties.10,11 So, improvement of the electroactive β-phase in PVDF is of great importance for the electronic and biomedical applications of this polymer.
Currently, several research work have reported to improve the electroactive β phase nucleation in PVDF as well as its dielectric properties by incorporating fillers like metal nanoparticles (NPs),12–14 clays,15–18 ceramics,19,20 metal oxide NPs,21 carbon black,22 carbon nanotubes,23,24 graphene,25 inorganic salts,26,27 quaternary phosphorus salt functionalized graphene,28 ferrites,29,30 carbon nanofibers,31–33 some other polymers34,35 etc. However, literatures on the effect of rare earth salts on the crystalline structures of PVDF as well as its dielectric properties are very scarce. Relatively large size and low capacity for covalent bond formation make these earth cations (R3+) ions different in chemical properties from the transition metal cations like Fe3+, Al3+, Cr3+ etc. Due to these properties, rare earth ions can form complexes via electrostatic i.e. ionic bond formation.36,37 Previous reports have showed that the rare earth salts have a noticeable impact on the structural, optical and electrical properties of some polymers.38–41 Recently, Hassen et al. prepared lanthanum(III) chloride (LaCl3) doped PVDF film by casting the mixture of PVDF in N,N-dimethylformamide and LaCl3 in absolute ethanol.42 They showed that the presence of LaCl3 in PVDF increased the dielectric constant and alternating current (ac) conductivity. El-Sayed et al., also investigated the dielectric properties ErCl3 or GdCl3 (3 mass%) doped PVDF films. However, little attention has been paid about the electroactive β phase nucleation mechanism and its direct effect on the enhancement of dielectric properties.
The present work involves fabrication of cerium(III)/yttrium(III) nitrate hexahydrate (Ce(NO3)3·6H2O and Y(NO3)3·6H2O) (1–30 mass%) doped PVDF thin films by simple solution casting method. The significant role of the rare earth salts on the improvement of electroactive β nucleation and dielectric properties of the modified PVDF thin films have been investigated and discussed in terms of interfacial phenomena.
2. Experimental
2.1. Materials
The materials used in the study are poly(vinylidene fluoride) (PVDF) pellets (Aldrich, Germany. Mw: 180
000 GPC, Mn: 71
000), cerium(III) nitrate hexahydrate (Ce(NO3)3·6H2O) (Acros Organics, India), yttrium(III) nitrate hexahydrate (Y(NO3)3·6H2O) (Acros Organics, India), dimethyl sulfoxide (DMSO) (Merck, India).
2.2. Fabrication of cerium/yttrium nitrate hexahydrate salt–PVDF thin films
Initially, 0.4 g of PVDF was dissolved in 8 ml DMSO at 60 °C to form a clear solution. Then desired amount of Ce(NO3)3·6H2O or Y(NO3)3·6H2O (1–30 mass%) was added to the PVDF solution and magnetically stirred for 12 hours at 60 °C followed by ultrasonication (30 min) under ambient condition. The mixture was then cast on a clean Petri dish to obtain the film and heated at 80 °C in a dust free oven for 12 hours for complete evaporation of the solvent. Pure PVDF film was also prepared without adding any salts under same conditions. The thickness of the films was in the range of 50–60 μm. All samples were stored in a vacuum desiccator for further characterization. Sample designations are tabulated as follows (Table 1).
Table 1 Sample designations showing respective compositions
Sample name |
Amount of PVDF (g) |
Percentage of salts (mass%) |
Amount of salts (g) |
Name of the salt added to PVDF |
PVDF |
0.4 |
0 |
0 |
|
PCe 1 |
0.4 |
1 |
0.004 |
Ce(NO3)3·6H2O |
PCe 5 |
0.4 |
5 |
0.020 |
PCe 10 |
0.4 |
10 |
0.040 |
PCe 20 |
0.4 |
20 |
0.080 |
PCe 30 |
0.4 |
30 |
0.120 |
PY 1 |
0.4 |
1 |
0.004 |
Y(NO3)3·6H2O |
PY 5 |
0.4 |
5 |
0.020 |
PY 10 |
0.4 |
10 |
0.040 |
PY 20 |
0.4 |
20 |
0.080 |
PY 30 |
0.4 |
30 |
0.120 |
2.3. Instrumentation
2.3.1. Thermal gravimetric analysis (TGA). Thermal gravimetric analysis (TGA) was performed using a TGA/SDTA851e (Mettler Toledo AG) under nitrogen atmosphere with a constant flow rate of 40 ml min−1 using ceramic crucibles having 70 μl capacity. The samples were heated from 50 °C to 600 °C at a heating rate of 10 °C min−1.
2.3.2. Field emission scanning electron microscopy (FESEM). The morphology and microstructure of the thin films were observed using field emission electron microscope (FESEM) (INSPECT F50, Netherland).
2.3.3. X-ray diffraction (XRD). The XRD patterns of the films were recorded by X-ray diffractometer (Model-D8, Bruker AXS Inc., Madison, WI) with 2θ varying from 10° to 40° and a scan speed of 0.3 s per step using nickel filtered Cu-Kα radiation operating under a voltage of 40 kV.
2.3.4. Fourier transform infrared spectroscopy (FTIR). The films were further characterized using FTIR (FTIR-8400S, Shimadzu) to determine the effect of salts addition on phase nucleation. The absorbance data of the films with thickness 50–60 μm were recorded in the wavenumber range from 400 cm−1 to 1000 cm−1 with a resolution of 4 cm−1. 50 scans were performed for each sample.The fraction of β-phase (F(β)) in samples were calculated using Lambert–Beer law which is,
|
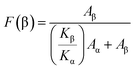 | (1) |
where,
Aα and
Aβ are the absorbance at 764 cm
−1 and 840 cm
−1, respectively and
Kβ (7.7 × 10
4 cm
2 mol
−1) and
Kα (6.1 × 10
4 cm
2 mol
−1) are the absorption coefficients at the respective wavenumber.
11,15,30
2.3.5. Differential scanning calorimetry (DSC). The crystallization and melting behaviour of the pure PVDF and composite thin films were analysed using a differential scanning calorimeter (DSC-60, Shimadzu, Singapore). The instrument was calibrated with an indium standard and all the studies were performed under N2 gas atmosphere. All the samples were heated from 80 °C to 200 °C at the rate of 10 °C min−1. The enthalpies (ΔHm) of fusion or melting enthalpies of the samples were obtained by calculating the areas under the endothermic peaks of the DSC curves. Then, the degree of crystallinity (Xc) of the samples was evaluated using the following equation:where, ΔHm is the heat of melting or enthalpy of fusion and ΔH100% is the melting enthalpy of 100% crystalline PVDF with a value 104.6 J g−1.11,15
2.3.6. Dielectric properties measurements. The dielectric properties were investigated using a digital LCR meter (Agilent, E4980A). The capacitance (C) and tangent loss (tan
δ) were recorded in the frequency range 20 Hz to 2 MHz at room temperature applying 1 V ac voltage across the two opposite surfaces of the samples. The dielectric constant (ε) and the ac conductivity (σac) of the samples were calculated using following eqn (3) and (4) respectively, |
σac = 2πfε0ε tan δ
| (4) |
where, d and A are the thickness and area of the samples respectively and f is the frequency in Hz applied across the samples and ε0 is permittivity of free space with value 8.854 × 10−12 F m−1.11,15
3. Results and discussions
3.1. Thermal gravimetric analysis (TGA)
Thermal stability of pure PVDF and salts doped PVDF thin films have been investigated by TGA thermographs. Fig. 1 shows the TGA thermographs of pure PVDF and composite thin films. Only one single weight loss is observed at 430 °C for pure PVDF film. The Ce(NO3)3·6H2O modified samples showed two stage mass loss: first one is at 195 °C and the second one in the 435–460 °C range with increasing doping concentration of the salt (Fig. 1a). Y(NO3)3·6H2O doped PVDF films exhibited similar pattern of mass losses occurring at 230 °C and 447 °C respectively for all samples (Fig. 1b).
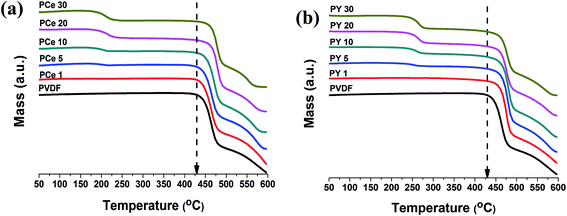 |
| Fig. 1 TGA thermographs of pure PVDF and the salts loaded PVDF thin films; (a) Ce(NO3)3·6H2O loaded PVDF thin films and (b) Y(NO3)3·6H2O loaded PVDF thin films. | |
The initial weight losses at 195 °C and 230 °C in the composite films are due to thermal evolution of water of crystallization in the Ce(NO3)3·6H2O or Y(NO3)3·6H2O salts respectively. The shifting in thermo-degradation temperature from 5 °C to 30 °C with increasing Ce(NO3)3·6H2O content in PVDF matrix and to 27 °C for Y(NO3)3·6H2O modified films may be attributed to the strong interaction between the polymer chains and the salts yielding thermal stability to the polymer chains closer to the salt interface.30,44
3.2. Field emission scanning electron microscopy (FESEM)
Fig. 2 and 3 shows the surface morphology and microstructure of pure PVDF and salts modified PVDF thin films.
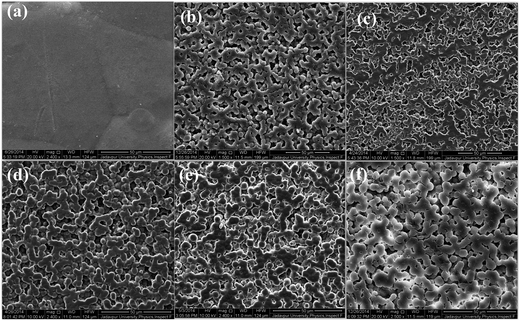 |
| Fig. 2 FESEM images of pure PVDF and the Ce(NO3)3·6H2O loaded PVDF thin films (PCe 1, PCe 5, PCe 10, PCe 20 and PCe 30). | |
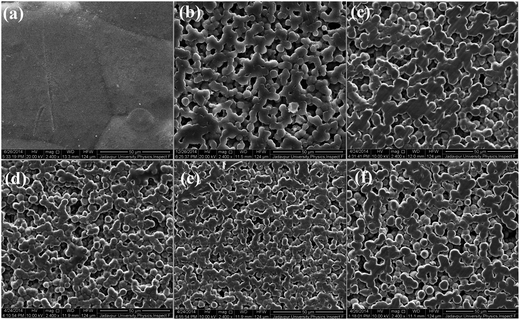 |
| Fig. 3 FESEM images of pure PVDF and the Y(NO3)3·6H2O loaded PVDF thin films (PY 1, PY 5, PY 10, PY 20 and PY 30). | |
The micrograph of pure PVDF thin film shows domains with diameter in the range 50–70 μm. Whereas in case of Ce(NO3)3·6H2O or Y(NO3)3·6H2O doped films, clear formation of small spherical domains or spherulites with improved smoothness can be observed. The diameter of the spherulites was found to vary from 3 μm to 5 μm (Fig. 2 and 3). Generally, α and γ phase dominant PVDF domains have diameter greater than 10 μm.14,45 Thus the formation of smaller spherulites (diameter ∼ 3–5 μm) in PVDF may be attributed to the formation of electroactive β phase in the salts modified PVDF thin films.14
3.3. X-ray diffraction analysis
The crystalline phases of pure PVDF and the salt doped PVDF thin films have been studied using X-ray diffraction (XRD) spectroscopy. Fig. 4a and 5a represent the XRD pattern of Ce(NO3)3·6H2O and Y(NO3)3·6H2O doped PVDF thin films respectively. The XRD spectrum of pure PVDF shows diffraction peaks around 17.6° (100), 18.3° (020), 19.9° (021) and 26.6° ((201), (310)) corresponding to non-polar α phase and a tiny peak at 38.3° (211) assigned to polar γ phase.10,11,15 However, after addition of Ce(NO3)3·6H2O or Y(NO3)3·6H2O salt in PVDF, all diffraction peaks related to α and γ phase were completely diminished and two prominent peaks around 20.7° ((110), (200)) and 36.7° ((020), (100)) appear confirming the nucleation of electroactive β phase in the composite thin films.10,11,15 Thus the electroactive β phase crystallization process is significantly accelerated in presence of both the salts. On closer observation of the intensities of the main diffraction peak of β phase (2θ = 20.7°) maximum β phase crystallization was found to occur for PCe 10 and PY 5.
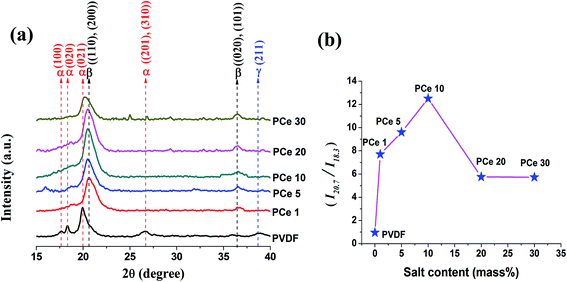 |
| Fig. 4 (a) XRD patterns of pure PVDF and Ce(NO3)3·6H2O doped PVDF thin films and (b) ratio of I20.7 and I18.3 of the thin films. | |
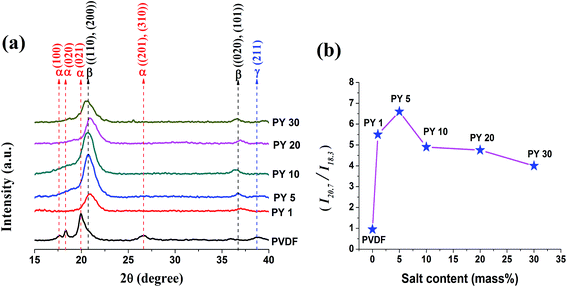 |
| Fig. 5 (a) XRD patterns of pure PVDF and Y(NO3)3·6H2O doped PVDF thin films and (b) ratio of I20.7 and I18.3 of the thin films. | |
Fig. 4b and 5b represent the ratio of the intensity of the peak at 20.7° and 18.3° (I20.7/I18.3) which is a measure of α and β phase content in the polymer.45 For pure PVDF, the ratio is 0.95 which was found to gradually increase and then decrease with doping concentration of both the salts in PVDF. The ratio reaches maximum value 12.5 for 10 mass% loading of Ce(NO3)3·6H2O (Fig. 4b) and 6.6 for 5 mass% doping of Y(NO3)3·6H2O (Fig. 5b). Hence, the nucleation of the electroactive β phase is comparatively more facilitated at low concentration of Y(NO3)3·6H2O than Ce(NO3)3·6H2O. This disparity in nucleating action may be due to the smaller size and more positive charge density of Y3+ ion than Ce3+ ion which is also confirmed by TGA thermographs where degradation temperature corresponding to the decomposition of structural water molecules of the salts is about 35 °C higher for the Y(NO3)3·6H2O modified films implying more strong bonding of water molecules with Y3+ ion as well as with polymer chains.
3.4. Fourier transform infrared spectroscopy
The nucleation of electroactive β phase in the films was further investigated by Fourier transform infrared (FTIR) spectra. Fig. 6a and 7a represent the FTIR spectra of pure PVDF and Ce(NO3)3·6H2O or Y(NO3)3·6H2O doped polymer. The absorbance spectrum of pure PVDF displays bands around 488 cm−1 (CF2 waging) 532 cm−1 (CF2 bending), 614, 764 cm−1 (CF2 bending and skeletal bending) 795 and 975 cm−1 (CH2 rocking) assigned to α-phase and small band at 840 cm−1 (CH2 rocking, CF2 stretching and skeletal C–C stretching) is due to β phase.10,11,15 Upon the addition of salt in PVDF, absorbance bands due to non-polar α phase almost vanished and characteristic absorbance bands corresponding to polar or electroactive β phase around 445 cm−1 (CF2 rocking and CH2 rocking), 479 cm−1 (CF2 deformation) 510 cm−1 (CF2 stretching), 600 cm−1 (CF2 wag) and 840 cm−1 (CH2 rocking, CF2 stretching and skeletal C–C stretching) started to emerge prominently.10,11,15 The intensity of electroactive β-PVDF bands notably increased up to 10 mass% doping of Ce(NO3)3·6H2O and 5 mass% loading of Y(NO3)3·6H2O in PVDF matrix respectively and then got reduced at higher salt concentrations.
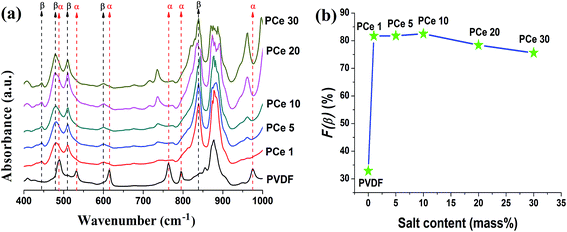 |
| Fig. 6 (a) FTIR spectra of pure PVDF and Ce(NO3)3·6H2O doped PVDF thin films and (b) evaluation of β-phase content with increasing Ce(NO3)3·6H2O content form IR spectra. | |
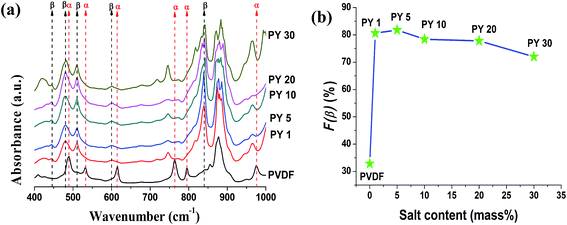 |
| Fig. 7 (a) FTIR spectra of pure PVDF and Y(NO3)3·6H2O doped PVDF thin films and (b) evaluation of β-phase content with increasing Y(NO3)3·6H2O content form IR spectra. | |
The relative fraction of β phase content (F(β)) in the salt loaded PVDF thin films was evaluated using eqn (1) and the variation of F(β) (%) with salt content (mass%) has been graphically presented in Fig. 6b and 7b. The electroactive β phase content increased for all the salt concentrations. But the interesting fact is that 10 mass% Ce(NO3)3·6H2O is needed to reach F(β) value ∼82.3% (Fig. 2b) whereas only 5 mass% Y(NO3)3·6H2O was sufficient to achieve ∼81.8% of electroactive β phase content in the composite films. Thus, FTIR spectroscopic data also conforms to the higher catalytic or nucleating effect of yttrium salt on the development of electroactive β phase in the PVDF composite films.
3.5. Differential scanning calorimetry
Differential scanning calorimetry (DSC), a complementary technique of XRD and FTIR was utilized for the verification of the different crystalline phases in PVDF. Fig. 8 represents the DSC thermographs of pure PVDF and salt loaded PVDF thin films. The thermograph of pure PVDF shows a prominent melting peak at 163.8 °C corresponding to nonpolar α phase crystallization. However for all the salt modified PVDF films the melting temperature (Tm) shifted to higher temperatures (by ∼3.2 to 3.8 °C) suggesting more electroactive β phase nucleation in the composite films which is consistent with XRD and FTIR data.10,11,15,18,24
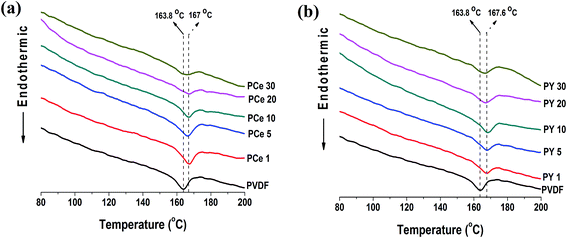 |
| Fig. 8 DSC thermographs of pure PVDF and the salts loaded PVDF thin films; (a) Ce(NO3)3·6H2O loaded PVDF thin films and (b) Y(NO3)3·6H2O loaded PVDF thin films. | |
Information about the melting enthalpy (Hm (J g−1)) and degree of crystallinity (Xc) were also obtained from the DSC thermographs. The melting enthalpies were determined from DSC thermographs and the degree of crystallinity has been computed using eqn (2). The variation of Hm and Xc with salt concentration have been graphically shown in Fig. 9.
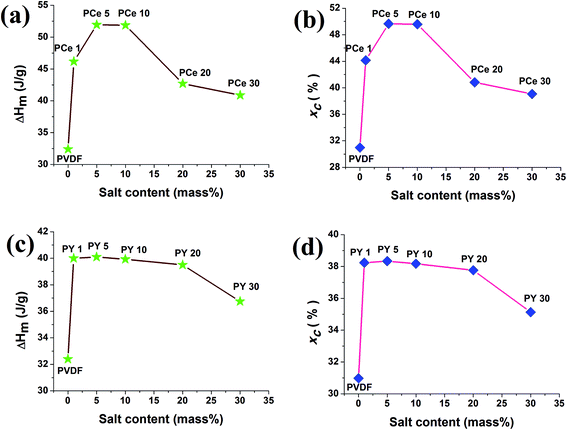 |
| Fig. 9 Evaluation of melting enthalpy (a, c) and degree of crystallinity (b, d) of pure PVDF and Ce(NO3)3·6H2O/Y(NO3)3·6H2O modified PVDF thin films with increasing salts content from DSC thermographs. | |
The melting enthalpy and the degree of crystallinity of the composite films were found to increase with doping concentration of both the salts. Highest crystallinity of about 49.5% was achieved in case of PCe 5 and PCe 10 whereas 39.3% was observed for PY 5. The increase in crystallinity for lower loading of the salts (up to 10 mass% loading for Ce(NO3)3·6H2O and 5 mass% doping of Y(NO3)3·6H2O) may be attributed to catalytic or nucleating action of the salts.11,15 And further loading of the salts leads to confinement of movement of the polymer chains resulting decrease in the crystallinity values.11,15
3.6. Mechanism of electroactive β phase nucleation
Our previous study on clay mineral–PVDF and Fe2O3/Co3O4 doped PVDF thin films suggest that strong interfacial interaction between the positive CH2 dipoles of the polymer chains and the negatively charged surfaces of the clay minerals or Fe2O3/Co3O4 NPs leads to nucleation of stabilized longer TTTT conformation i.e. β phase crystallization on the fillers surfaces.11,15
In the present work also, it is also necessary to investigate the probable interaction mechanism between the salts and the polymer chains which improves the electroactive β phase nucleation in the composite samples. The nucleation of β phase in composite samples may be attributed to the hydrogen bonds formation between the water molecules of the salts and the polar –CF2 dipoles of the polymer.27,46,47 After addition of salts in PVDF solution, the water molecules of the salts interact with the polar –CF2 dipoles of PVDF. When the thin films are formed at 80 °C, the salts act as the substrate for β-phase nucleation. Thus, strong ion–dipole interaction between the polar CF2 dipoles of PVDF and the water molecules of the salts promotes longer alignment of stabilized all trans conformation i.e. electroactive β phase nucleation in Ce(NO3)3·6H2O or Y(NO3)3·6H2O modified PVDF thin films. The schematic for possible β phase nucleation mechanism or interaction between the salt and the polymer chains has been presented in Fig. 10.
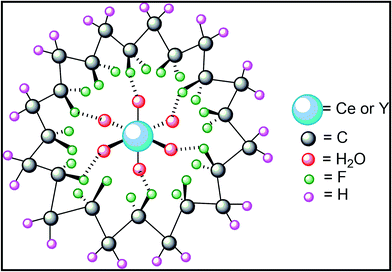 |
| Fig. 10 Proposed schematic of electroactive β phase transformation mechanism. | |
3.7. Dielectric properties
3.7.1. Salt content dependence. Fig. 11 represents the variation of dielectric constant and tangent loss (tan
δ) with increasing salt concentration at two different frequencies: 100 Hz and 1 MHz. Improvement in dielectric constant with salt concentration have been observed at 100 Hz. However, at 1 MHz, the dielectric constant increased up to 10 mass% doping for both the salts and then almost stabilises at higher concentrations. This enhancement of dielectric constant at lower frequency i.e. at 100 Hz may be due to the appearance of large Maxwell–Wagner–Sillars (MWS) interfacial polarization11,15,48–51 i.e. large accumulation of space charges between the interfaces of the salts and the polymer chains at lower frequency (100 Hz) shown schematically shown in Fig. 10. Upon the addition of salts in polymer, the salt molecules are well distributed and separated from each other in PVDF matrix. The strong interaction via formation of hydrogen bond between the water of salt molecules and the polar –CF2 dipoles of polymer chains not only improve the high dielectric β phase crystallization but also enhance the effective interfacial interfaces in the salts modified polymer samples. With increasing concentration of the salt content, the number of salt molecules and the interfacial area per unit volume associated with the interface of salt molecules and polymer chain increases while the inter-particle distance reduces. This enhances the average localized polarization accompanied with the salt molecules and the coupling between neighbouring polymer grains, which improves the dielectric constant of the salts modified polymer films.11,49
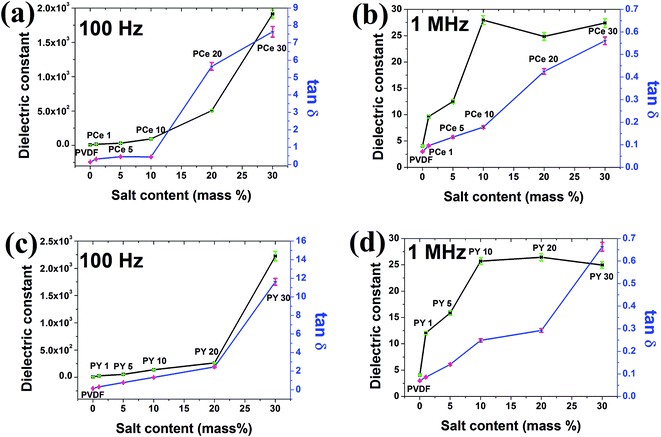 |
| Fig. 11 Salts content dependence of dielectric constants and tangent losses at 100 Hz and 1 MHz; (a, b) the Ce(NO3)3·6H2O loaded PVDF thin films (PCe 1, PCe 5, PCe 10, PCe 20 and PCe 30) and (c, d) the Y(NO3)3·6H2O loaded PVDF thin films (PY 1, PY 5, PY 10, PY 20 and PY 30). Error bars represent the standard deviation of the experiment conducted in triplicate and were assessed by one way ANOVA using graph pad Instat version 5.0 software. | |
At higher frequency dielectric constant is mainly dominated by dipolar polarization.11,48–51 As the variation of the electroactive β phase content (F(β)) in composite samples is small at higher concentration of the salt in polymer matrix (Fig. 6b and 7b), so the number of dipoles do not vary much for those samples. Hence, the dielectric constant exhibits almost stable values for higher doping of the salt (10–30 mass%) at 1 MHz. Highest dielectric constant was found to be ∼2.2 × 103 and 1.9 × 103 at 100 Hz for 30 mass% loading of Ce(NO3)3·6H2O and Y(NO3)3·6H2O in PVDF respectively. The results are quite promising compared to previously reported dielectric constant data by Hassen et al.42 and El-Sayed et al.43 Hassen et al. achieved a dielectric constant of 100 by adding 10 mass% LaCl3 in PVDF at 100 Hz whereas, El-Sayed et al. reported ε ∼ 15 and 20 by adding 3 mass% GdCl3 and ErCl3 respectively. The tangent loss (tan
δ) of the samples was found to increase with salt concentration in a non-linear fashion.
3.7.2. Frequency dependence. Fig. 12 shows the frequency dependence of dielectric constant and tangent loss (tan
δ) of pure PVDF and Ce(NO3)3·6H2O/Y(NO3)3·6H2O loaded PVDF thin films under ambient condition. The dielectric constant of all the films decreased with frequency due to reduction of effective number of dipoles. At low frequency the dipoles may follow the direction of the ac electrical field but with increasing frequency the dipoles starts to lag behind the frequency of the electric field resulting decrease in dielectric value. After reaching the characteristic value of frequency 1/t (t is the relaxation time), the dielectric constant shows a relaxation. This is because at very higher frequency the dipoles are not able to align with the field.42,43,49,50
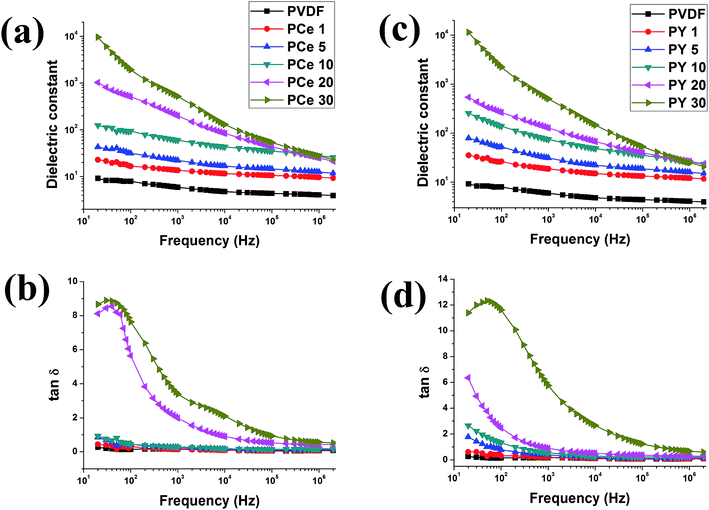 |
| Fig. 12 Frequency dependence of dielectric properties of pure PVDF and Ce(NO3)3·6H2O/Y(NO3)3·6H2O doped PVDF thin films; (a, c) dielectric constants, (b, d) tangent losses. | |
The reduction of dielectric constant with increasing frequency and enhancement of dielectric constant with increasing salt content can be satisfactorily explained by MWS interfacial effect11,15,48–51 (Fig. 12a and c). On the application of electric field on the samples, the charge carriers from the impurities (i.e. salts) or the electrode move freely and accumulate at the interface between the salts and polymer due to the difference in conductivity between the polymer and the salts. This movement and accumulation process continues smoothly at low frequency as the charge carriers or dipoles get enough time for their movement. Consequently, the movement and accumulation of charge carriers at the interface results in large polarization and dielectric constant. With increasing frequency the movement of the charge carriers or dipoles are limited due to frequency mismatch.15,42,43,48 Hence a decrease in dielectric constant is observed with increasing frequency for all samples. The dielectric constants are achieved ∼9.5 × 103 for PCe 30 and 11.5 × 103 for PY 30 at 20 Hz.
Fig. 12b and c show the frequency dependence tan
δ of pure PVDF and the salt modified PVDF thin films. The tan
δ has been decreased with increasing frequency due to MWS effect. Sample PCe 20, PCe 30 and PY 30 show a tangent loss peak at ∼40–50 Hz which may be due to reaching the characteristic value of frequency (1/t) for those samples.42
Fig. 13 represent the dependency of ac conductivity of the samples on the frequency. As expected ac conductivity increased with loading concentration of the salts and with increasing frequency due to MWS effect between the interface of salt and the polymer matrix.
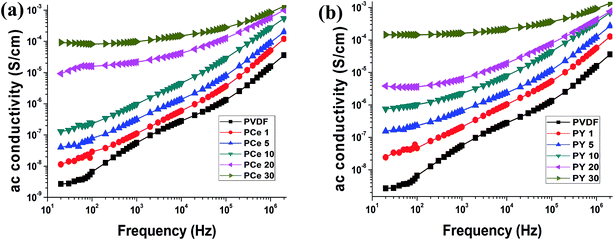 |
| Fig. 13 Frequency dependence of ac conductivities; (a) pure PVDF and the Ce(NO3)3·6H2O doped PVDF thin films (PCe 1, PCe 5, PCe 10, PCe 20 and PCe 30) and (b) pure PVDF and the Y(NO3)3·6H2O doped PVDF thin films (PY 1, PY 5, PY 10, PY 20 and PY 30). | |
The conductivity of the samples may be divided into two regions. First one is the lower frequency region which is mainly dominated by dc conductivity and the conductivity of the higher frequency region which is mainly depended on frequency. The nature of the curve correlates directly with MWS effect and dielectric relaxation at higher frequency.15,51 Thus the variation of ac conductivity with frequency and salt concentration are mainly due to MWS interfacial effects and dipolar relaxations.
4. Conclusions
Electroactive β phase nucleation has been observed in Ce(NO3)3·6H2O and Y(NO3)3·6H2O modified PVDF thin films. About 82.3% of electroactive β phase nucleation is obtained for 10 mass% Ce(NO3)3·6H2O and 81.8% for 5 mass% of Y(NO3)3·6H2O in the polymer matrix respectively. Strong interactions via hydrogen bonding between the hydrogen atoms of the salts and the –CF2 dipoles of the polymer chains lead to the formation of longer TTTT conformation i.e. electroactive β phase nucleation in the composite films. Large dielectric constant of about 9.5 × 103 for PCe 30 and 11.5 × 103 for PY 30 has been achieved at 20 Hz. Large interfacial polarizations at the interface between the salts and the polymer matrix results in high dielectric values observed in PCe 30 and PY 30 films.
Thus, given the significantly improved electroactive and dielectric thin films may be a promising candidate for development of energy storage devices (like super capacitors, solid electrolyte batteries, self-charging power cells, lithium ion batteries etc.), nanogenerator, sensors and actuators.
Acknowledgements
We are thankful to University Grants Commission (UGC), Government of India (F. 17-76/2008 (SA-1)) for the financial assistance and Mr Nayim Sepay, Department of Chemistry, Jadavpur University for his fruitful suggestion in drawing the chemical structure of mechanism section.
References
- T. T. Wang, J. M. Herbert and A. M. Glass, The Applications of Ferroelectric Polymers, Chapman and Hall, New York, 1988 Search PubMed.
- H. S. Nalwa, Ferroelectric Polymers: Chemistry, Physics and Applications, Marcel Dekker, New York, 1995 Search PubMed.
- A. J. Lovinger, Science, 1983, 220, 1115–1121 CAS.
- X. Zhou, B. Chu, B. Neese, M. Lin and Q. M. Zhang, IEEE Trans. Dielectr. Electr. Insul., 2007, 14(5), 1133–1138 CrossRef.
- Y. Lu, J. Claude, B. Neese, Q. M. Zhang and Q. Wang, J. Am. Chem. Soc., 2006, 128(25), 8120–8121 CrossRef CAS PubMed.
- N. Karawasa and W. A. Goddard III, Macromolecules, 1992, 25(26), 7268–7281 CrossRef.
- A. B. Silva, M. Arjmand, U. Sundararaj and R. E. S. Bretas, Polymer, 2014, 55, 226–234 CrossRef PubMed.
- V. Tomer, E. Manias and C. A. Randall, J. Appl. Phys., 2011, 110, 044107–044110 CrossRef PubMed.
- D. Kim, S. Hong, J. Hong, Y. Y. Choi, J. Kim, M. Park, T. Sung and K. No, J. Appl. Polym. Sci., 2013, 130(6), 3842–3848 CAS.
- P. Martins, A. C. Lopes and S. Lanceros-Mendez, Prog. Polym. Sci., 2013, 39(4), 683–706 CrossRef PubMed.
- P. Thakur, A. Kool, B. Bagchi, S. Das and P. Nandy, Phys. Chem. Chem. Phys., 2015, 17, 1368–1378 RSC.
- H. P. Xu and Z. M. Dang, Chem. Phys. Lett., 2007, 438(4–6), 196–202 CrossRef CAS PubMed.
- K. S. Deepa, M. S. Gopika and J. James, Compos. Sci. Technol., 2013, 78, 18–23 CrossRef CAS PubMed.
- D. Mandal, K. Henkel and D. Schmeißer, Mater. Lett., 2012, 73, 123–126 CrossRef CAS PubMed.
- P. Thakur, A. Kool, B. Bagchi, S. Das and P. Nandy, Appl. Clay Sci., 2014, 99, 149–159 CrossRef CAS PubMed.
- D. Shah, P. Maiti, E. Gunn, D. Schmidt, D. D. Jiang and C. A. Batt, Adv. Mater., 2004, 16(14), 1173–1177 CrossRef CAS.
- T. U. Patro, M. V. Mhalgi, D. V. Khakhar and A. Misra, Polymer, 2008, 49(16), 3486–3499 CrossRef CAS PubMed.
- L. Priya and J. P. Jog, J. Appl. Polym. Sci., 2003, 89(8), 2036–2040 CrossRef CAS.
- P. Mishra and P. Kumar, Compos. Sci. Technol., 2013, 88, 26–32 CrossRef CAS PubMed.
- T. Zhou, J. W. Zha, R. Y. Cui, B. H. Fan, J. K. Yuan and Z. M. Dang, ACS Appl. Mater. Interfaces, 2011, 3(7), 2184–2188 CAS.
- W. Wu, X. Huang, S. Li, P. Jiang and T. Toshikatsu, J. Phys. Chem. C, 2012, 116(47), 24887–24895 CAS.
- Y. Konishi and M. Cakmak, Polymer, 2006, 47(15), 5371–5391 CrossRef CAS PubMed.
- S. L. Jiang, U. Yu, J. J. Xie, L. P. Wang, Y. K. Zeng and M. Fu, J. Appl. Polym. Sci., 2010, 116(2), 838–842 CAS.
- A. Lund, C. Gustafsson, H. Bertilsson and W. Rychwalski, Compos. Sci. Technol., 2011, 71, 222–229 CrossRef CAS PubMed.
- Y. J. Li, M. Xu, J. Q. Feng and Z. M. Dang, Appl. Phys. Lett., 2006, 89(7), 072902–072905 CrossRef PubMed.
- R. Song, G. Xia, X. Xing, L. He, Q. Zhao and Z. Mad, J. Colloid Interface Sci., 2013, 401, 50–57 CrossRef CAS PubMed.
- Y. K. A. Low, L. Y. Tan, L. P. Tan, F. Y. C. Boey and K. W. Ng, J. Appl. Polym. Sci., 2013, 128(5), 2902–2910 CrossRef CAS.
- J. Wang, J. Wu, W. Xu, Q. Zhang and Q. Fu, Compos. Sci. Technol., 2014, 91, 1–7 CrossRef CAS PubMed.
- V. Sencadas, P. Martins, A. Pităes, M. Benelmekki, J. L. G. Ribelles and S. Lanceros-Mendez, Langmuir, 2011, 27(11), 7241–7249 CrossRef CAS PubMed.
- P. Martins, C. M. Costa, M. Benelmekki, G. Botelho and S. L. Mendez, CrystEngComm, 2012, 14(8), 2807–2811 RSC.
- L. L. Sun, B. Li, Z. G. Zhang and W. H. Zhong, Eur. Polym. J., 2010, 46(11), 2112–2119 CrossRef CAS PubMed.
- L. L. Sun, B. Li, Y. Zhao, G. Mitchell and W. H. Zhong, Nanotechnology, 2010, 21(30), 305702–305710 CrossRef CAS PubMed.
- P. Costa, J. Silva, V. Sencadas, C. M. Costa, F. W. J. van Hattum and J. G. Rocha, Carbon, 2009, 47(11), 2590–2599 CrossRef CAS PubMed.
- T. U. Patro, M. V. Mhalgi, D. V. Khakhar and A. Misra, Polymer, 2008, 49, 3486–3490 CrossRef CAS PubMed.
- Y. J. Li, Y. Iwakura, L. Zhao and H. Shimizu, Macromolecules, 2008, 41, 3120–3125 CrossRef CAS.
- M. Takahashi, Y. Hara, K. Aoshima, H. Kurihara, T. Oshikawa and M. Yamashita, Tetrahedron Lett., 2000, 41, 8485–8488 CrossRef CAS.
- M. C. Silva, A. S. S. de Camargo, L. A. O. Nunes, R. A. Silva and A. Marletta, J. Non-Cryst. Solids, 2008, 354, 5496–5503 CrossRef CAS PubMed.
- F. Xie and Z. Zheng, Phys. B, 2004, 349, 415–419 CrossRef CAS PubMed.
- H. Suzuki, Y. Hattori, T. Lizuka, K. Yuzawa and N. Matsumoto, Thin Solid Films, 2003, 438, 288 CrossRef.
- H. Huang and B. Yan, Mater. Sci. Eng., B, 2005, 117, 261–265 CrossRef PubMed.
- G. A. Kumar, Mater. Lett., 2002, 55, 364–369 CrossRef CAS.
- A. Hassen, T. Hanafy, S. El-Sayed and A. Himanshu, J. Appl. Phys., 2011, 110, 114119–114127 CrossRef PubMed.
- S. El-Sayed, T. A. Abdel-Baset and A. Hassen, AIP Adv., 2014, 4, 037114–037115 CrossRef PubMed.
- K. Pal, D. J. Kang, Z. X. Zhang and J. K. Kim, Langmuir, 2009, 26(5), 3609–3614 CrossRef PubMed.
- B. S. Ince-Gunduz, R. Alpern, D. Amare, J. Crawford, B. Dolan, S. Jones, R. Kobylarz, M. Reveley and P. Cebe, Polymer, 2010, 51, 1485–1493 CrossRef CAS PubMed.
- B. P. Mandal, K. Vasundhara, E. Abdelhamid, G. Lawes, H. G. Salunke and A. K. Tyagi, J. Phys. Chem. C, 2014, 118, 20819–20825 CAS.
- M. Benz, W. B. Euler and O. J. Gregory, Macromolecules, 2002, 35, 2682–2688 CrossRef CAS.
- Y. Li, X. Huang, Z. Hu, P. Jiang, S. Li and T. Tanaka, ACS Appl. Mater. Interfaces, 2011, 3(11), 4396–4403 CAS.
- T. W. Dakin, IEEE Electr. Insul. Mag., 2006, 22(5), 11–28 CrossRef.
- A. Moliton, Applied Electromagnetism and Materials, Springer, New York, 2007, ch. 3 Search PubMed.
- A. C. Lopes, C. M. Costa, R. Sabater i Serra, I. C. Neves, J. L. Gomez Ribelles and S. Lanceros-Mendez, Solid State Ionics, 2013, 235, 42–50 CrossRef CAS PubMed.
Footnotes |
† Present address: Fuel Cell and Battery Division, Central Glass and Ceramic Research Institute, Kolkata-700032, India. |
‡ Present address: Department of Physics, IIEST, Howrah, West Bengal-711103, India. |
|
This journal is © The Royal Society of Chemistry 2015 |